Abstract
Free full text

Numb family proteins: novel players in cardiac morphogenesis and cardiac progenitor cell differentiation
Abstract
Vertebrate heart formation is a spatiotemporally regulated morphogenic process that initiates with bilaterally symmetric cardiac primordial cells migrating toward the midline to form a linear heart tube. The heart tube then elongates and undergoes a series of looping morphogenesis, followed by expansions of regions that are destined to become primitive heart chambers. During the cardiac morphogenesis, cells derived from the first heart field contribute to the primary heart tube, and cells from the secondary heart field, cardiac neural crest, and pro-epicardial organ are added to the heart tube in a precise spatiotemporal manner. The coordinated addition of these cells and the accompanying endocardial cushion morphogenesis yield the atrial, ventricular, and valvular septa, resulting in the formation of a 4-chambered heart. Perturbation of progenitor cells’ deployment and differentiation leads to a spectrum of congenital heart diseases. Two of the genes that were recently discovered to be involved in cardiac morphogenesis are Numb and Numblike. Numb, an intracellular adaptor protein, distinguishes sibling cell fates by its asymmetric distribution between the two daughter cells and its ability to inhibit Notch signaling. Numb regulates cardiac progenitor cell differentiation in Drosophila, and controls heart tube laterality in Zebrafish. In mice, Numb and Numblike, the Numb Family Proteins (NFPs), function redundantly and have been shown to be essential for epicardial development, cardiac progenitor cell differentiation, outflow tract alignment, atrioventricular septum morphogenesis, myocardial trabeculation and compaction. In this review, we will summarize the functions of NFPs in cardiac development, and discuss potential mechanisms of NFPs in the regulation of cardiac development.
Introduction: Numb and cardiac development
During the development of Drosophila peripheral nervous system, a single sensory organ precursor (SOP) cell undergoes several divisions to produce four cells that form an external sensory organ (Fig. 1A&B). In the first division of the SOP cell, Numb localizes asymmetrically at one pole of the mitotic cell cortex, so that only one daughter cell inherits the protein (Fig. 1C). As a result, this daughter becomes a pIIb cell, and the other becomes a pIIa cell (Fig. 1B&C) (1, 2). These two cells then divide to produce the different cell types of the sensory organ (Fig. 1A&B). Numb gain or loss of function results in two IIb cell or IIa cells, respectively, and it was discovered that Numb promotes IIb cell fate by inhibiting Notch signaling (1, 3, 4).
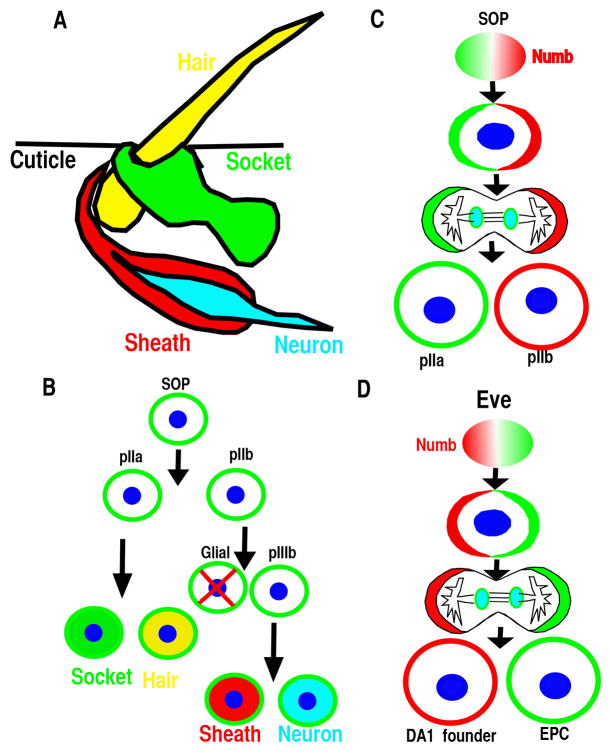
(A–B) The Drosophila sensory organ consists of four cells: hair, socket, sheath and neuron and is derived from the sensory organ precursor (SOP). (B–C) SOP divides asymmetrically in a stem cell-like fashion to generate the various cells of the sensory organ. The glial cell undergoes programmed cell death. (D) Eve positive mesoderm progenitor cell divides asymmetrically to generate DA1 founder and eve-expressing pericardial cells (EPC).
Since then, many more functions of Numb have been revealed. It functions as a component of the adherens junctions to regulate cell adhesion and cell migration (5), and controls the stability of p53 (6) and Gli1 (7) to regulate cancer initiation. Numb has also been reported to complex with β-catenin and to regulate neuroepithelial and epicardial development (8, 9). The functions of Numb specifying neural cell fate are conserved in vertebrates (10–12) (2, 4, 12, 13).
Recently, Numb has been revealed to regulate cardiac progenitor cell differentiation and cardiac development in different species. In Drosophila, Numb is involved in specification of cardiac cell type via Notch signaling interference (14). In Zebrafish, Numb is required for heart left-right asymmetric morphogenesis via regulating Notch signaling (15). In mice, there are two homologs Numb and Numblike (16, 17). Numb is expressed in adult cardiac cKit cells and is asymmetrically distributed during their asymmetric cell divisions (18, 19). Furthermore, Numb and Numblike, the Numb Family Proteins (NFPs), are essential for cardiac morphogenesis and differentiation during development as evidenced by a variety of defects in cardiac morphogenesis and progenitor differentiation in the cardiac specific NFPs knockout embryos(20).
The vertebrates’ cardiac morphogenesis depends on the addition and differentiation of progenitor cells from four different sources (21) (Fig. 2A–C). At approximately embryonic day 7.5 (E7.5), cardiac mesodermal cells arising in the anterior primitive streak migrate to the anterior ventral aspect to form a bilaterally symmetric heart field called the cardiac crescent (Fig. 2A) (22) (23). The cardiac crescent, the source of the first two progenitor sources, consists of first heart field (FHF) and secondary heart field (SHF) with the SHF residing dorsomedially relative to FHF in the crescent (Fig. 2A). Cells from FHF of the cardiac crescent will fold toward the ventral midline to form a linear heart tube at about E8.0 (Fig. 2B). The SHF cells initially residing dorsomedially to FHF are subsequently located to the pharyngeal and splanchnic mesoderm, from which they migrate to the pre-existing scaffold of the linear heart tube. The SHF cells will contribute to the right ventricle, OFT myocardium and to some endocardium at E8.5-E10.25 (Fig. 2C) (24–27). The cells derived from the SHF play an essential role in the orientation and patterning of the outflow tract (OFT) (28). Cardiac neural crest cells (CNCC), originating from postotic rhombomeres 6, 7 and 8, will migrate to the caudal pharynx and contribute significantly to the smooth muscle layer and endocardial cushion in the OFT (Fig. 2C). They are also involved in the formation of the aorticopulmonary septum, as demonstrated by lineage-tracing studies using neural crest-restricted Cre mouse lines (29, 30). CNCCs are essential for normal myocardial differentiation in the OFT and for the formation and remodeling of the great arteries (31, 32). The fourth population is the epicardial cells derived from the Pro-epicardial organ (PEO), which is located at the sinoatrial pole and atrioventricular junction at about E9.5 (Fig. 2C). This population contributes to fibroblast, smooth muscle cell and potentially other cardiac cell types (33). The epicardium regulates coronary vascular development, cardiac growth and morphogenesis.
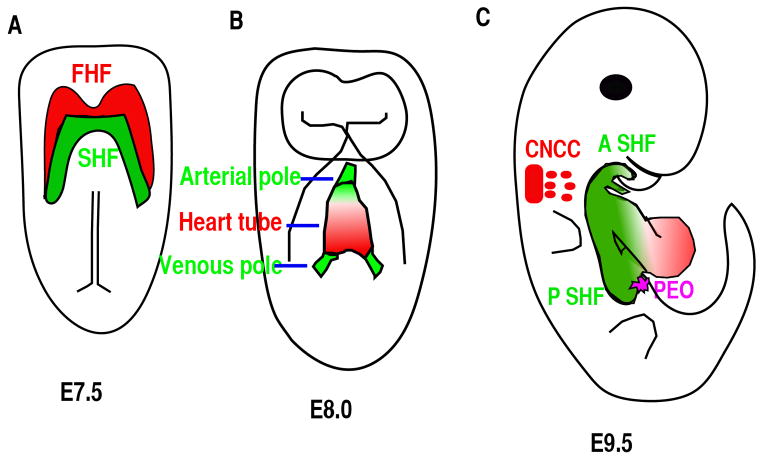
(A) Ventral view of an E7.5 embryo. Red color highlights the cardiac progenitor cells of the first heart field (FHF) and green color highlights another subset of cardiac progenitor cells that form the secondary heart field (SHF), and is located posteriorly and medially to the FHF. (B) Ventral view of the embryo at E8.5. Cells from the FHF migrate and then merge in the midline to form the heart tube, which then elongates on both arterial and venous poles via the addition of progenitor cells from the SHF. (C) Right lateral view of an E9.5 mouse embryo. The cardiac neural crest cells (CNCC) at rhombomere 6–8 gives rise to cells (green) that migrate to and colonize the distal cardiac outflow tract (OFT) to contribute to OFT alignment and septation. Cells from pro-epicardial organ (PEO) will migrate, attach and then cover the whole heart to form epicardium. A SHF: anterior second heart field; P SHF: posterior second heart field.
In a delicate structure like the heart, abnormal cell migration/differentiation during morphogenesis will cause malformations or congenital heart defects (CHD), which is the number one cause of birth defects in the world. Understanding how the heart is assembled at the cellular and molecular level is an essential step toward improving diagnosis and potential treatments for CHD. The variety of defects resulting from NFPs deletions in different cardiac cell types indicates that NFPs are essential and novel factors involved in heart morphogenesis and progenitor differentiation.
NFPs function in cardiac cell specification and differentiation
Numb was identified as the first cell fate determinant in Drosophila (1) due to its ability to inhibit Notch signaling via endocytosis, and its functions are conserved during the specification of neural cell fate in mammals (10–12) (2, 4, 12, 13). Numb’s function as a cell fate determinant for cardiac cell was initially studied in Drosophila. Cardiogenesis in Drosophila can be considered as a series of distinct developmental decisions. These include the sequential specification of mesoderm, dorsal mesoderm, and cardiac fate within the dorsal mesoderm, followed by the cell fate diversification of the cardioblast in each segment and the cardiac cell types in anterior-posterior heart tube (34). Numb mutant Drosophila is not defective in cardiac fate specification, but Numb is involved in the specification and differentiation of cardiac cell types such as pericardial cells at later stages of heart development. During the specification of pericardial cells, even skipped-expressing (Eve) myogenic progenitors divide asymmetrically and Numb is asymmetrically distributed with one daughter cell inheriting the majority of Numb (Fig. 1D). The presence of Numb inhibits Notch and Sanpodo signaling and causes this daughter cell to take the muscle founder cell fate (DA1). The other daughter cell that does not inherit Numb will take eve-expressing pericardial cell fate (Fig. 1D). Disruption of Numb results in more pericardial cells, while over-expressing Numb reduces the number of pericardial cells and induces more DA1 cells (35, 36). When Sanpodo, which is required for Notch signaling, is disrupted, the mutant displayed an opposite phenotype to the Numb mutant (36). Within individual segments of Drosophila heart, there are two nonidentical groups of cardiac cells: four pairs of cardioblasts express Tinman (Tin) and two pairs of cardioblasts alternating with the Tin expressing cells express Seven Up (Svp). The Tin expressing cells are generated by symmetric cell divisions from cardiac progenitor cells (37). The Svp expressing cells are generated by asymmetric cell divisions of heart progenitor cells in the mesoderm, with its sister cell becoming the Odd-pericardial cells (38). Numb promotes Svp-expressing cardiac cell at the expense of Odd-expressing pericardial cells. In the absence of Numb, Svp expressing cells are not observed, while the number of Tin expressing cells did not change (37), indicating that Numb is involved in cell specification only during asymmetric cell division (14).
In the mouse, NFPs global double knockout embryos die around E9.0 (12, 20, 39). Whether the knockout displays a cardiac progenitor specification defect is not clear. The early embryonic lethality of global double knockout prevents the studying of NFPs functions in later stages of cardiac development, but this can be overcome by conditional knockout technology. NFPs’ role in cardiac progenitor cell differentiation and renewal has been investigated with multiple Cre lines, which allow NFPs’ deletion at different stages. NFP deletion via the Mesp1-Cre, which is active in the mesoderm, disrupted cardiac progenitor renewal due to reduced proliferation, which results in hypoplastic OFT and right ventricle (40). The mechanism of how NFPs regulate cardiac progenitor self-renewal is not clear. NFPs regulate epicardial development and cardiac progenitor differentiation at later stages, and their disruptions at these stages cause various defects (9, 20, 41). In the following sections, we will discuss the functions of NFP in epicardial development, SHF progenitor cell differentiation, outflow tract morphogenesis, atrioventricular septation, and myocardial trabeculation.
NFPs are required for epicardial development by maintaining polarity
The epicardium, the outer cell layer of the heart, is composed of a single layer of epithelial cells that arises from the pro-epicardial organ (PEO) (Fig. 2C). Distinct compartments of PEO can be labeled by different molecular markers and contribute to different cardiac cell types (42). The pro-epicardial cells migrate from the PEO, attach and then spread to cover the whole heart beginning at E9.5 (33, 43). Signaling pathways that regulate epicardial cell detachment from the PEO are not clear, while pathways that regulate epicardial cell attachment to the heart and subsequent entry into the myocardium have been extensively studied. α4β1 integrin, which are expressed by epicardial cells (44), interact with fibronectin (45) and VCAM-1(46–48) expressed by myocardial cells to promote epicardial cell adherence to cardiomyocytes and spreading to cover the heart (44, 49). After attaching to the heart, a subset of epicardial cells undergo an epithelial to mesenchymal transition (EMT), and migrate into the myocardium (50–55). They subsequently differentiate into fibroblasts, smooth muscle cells, endocardial cells and potentially cardiomyocytes (42, 56–64). Many signaling pathways, such as FGF (65, 66), PDGF (67), Wnt/β-catenin (52, 68), RXRα (51, 69, 70), TGFβ (55) and Notch (54, 71), play a role in these processes. Other proteins expressed by epicardial cells such as Par3 (72), GATA4 (73), WT1 (68, 74–76), α4β1 integrin and podoplanin (77) are also required for proper epicardial development.
In epicardium, Numb localizes to the adherens junctions of epicardial cells at the G1 phase and to the basal domain at M phase (Fig. 3A) (9), similar to its localization in neuroepithelium (8). Polarity proteins such as Par3, Par6 and aPKC localize to the apical domain of epicardial cells (9, 72), indicating that epicardial cells are polarized in a manner similar to other epithelial cells (78). NFPs are required to maintain epicardial polarity (9). Although, multiple signaling pathways have been reported to regulate epicardial cell entry into the myocardium, the cellular mechanism is not clear. Time-lapse imaging and immunofluorescence staining enabled the discovery of epicardial cells that undergo parallel or perpendicular divisions with respect to the heart wall. The parallel divisions produce daughter cells to cover the heart, while a perpendicular division’s daughter cell invades the myocardium (Fig. 3) (9). NFPs are required for β-catenin to localize to adherens junctions. Conditional deletion of NFPs in epicardium results in disruption of epicardial adherens junction, epicardial polarity, and random mitotic spindle orientations, which might cause the observed epicardial EMT defects (9). The epicardial cell perpendicular division is an asymmetric cell division, as the daughter cell that enters into myocardium inherits more Numb and differentiates into a fibroblast or other cell types. The other daughter cell remaining within the epicardium maintains the epicardial cell fate. NFPs might play multiple roles in epicardial development. First, NFPs are required to stabilize adherens junctions, which are required to establish the mitotic spindle orientation. NFPs might regulate the stability of the components of adherens junctions such as β-catenin via endocytosis in epicardium. Secondly, Numb accumulation at the basal domain of the dividing epicardial cell beginning at the S-phase might also promote epicardial cell migration and differentiation. However, further experiments will be needed to determine whether and how NFPs regulate epicardial cell migration and differentiation.
NFPs regulate second heart field progenitor cell differentiation
In 2001, three groups using various methods including viral infection tracing, vital dye lineage tracing, tissue ablation, and enhancer trap tracing demonstrated that cells in the splanchnic mesoderm migrated to the elongating cardiac linear tube and gave rise to the right ventricle and OFT. These observations led to the discovery of the SHF (79–81) and changed our view of cardiogenesis. We now know the FHF gives rise to the left ventricle and part of the inflow region, while the right ventricle, OFT, interventricular septum, endocardium and part of the inflow region are derived from the SHF (26, 79–83).
Perturbation of SHF deployment and progenitor differentiation leads to a spectrum of CHDs. Several signaling pathways including FGF (79, 84, 85), Wnt (86–89), Hedgehog (90, 91), Notch (92), BMP (93) and retinoic acid (94) are involved in the deployment of Isl1 cells to the elongating linear heart tube and subsequent differentiation (24, 95, 96). FGF8 signaling functions upstream of Isl1 and deletion of Fgf8 specifically in SHF causes SHF morphogenesis defects (79, 84, 97). Type1 BMP receptor deletion and BMP4 deletion decrease the proliferation in SHF and results in OFT septation defects (93, 98, 99). Hedgehog is crucial for cardiac neural crest cell survival and is also required for OFT septation (90, 100, 101).
NFPs deletion via Nkx2.5Cre/+ displayed higher expression of progenitor markers such as Isl1, Tbx1, Fgf8 and Shox2 at E10.5. Isl1 expressional level in the knockout is significantly higher than that in the control littermates from E9.5 to E11.5. In addition, at E12.5 and E13.5, the knockouts displayed abnormal expressional levels of cardiomyocyte maturation/ differentiation markers such as MYH6, MYH11, BMP10, Irx3-5, indicating a role for NFP in cardiomyocyte differentiation from progenitor cells. Supporting this notion, overexpressing Numb in the pluripotent stem cells in an embryoid body culture system promotes cardiac progenitor differentiation and decreases Isl1 expression in an endocytosis dependent manner (20). Surprisingly, the differentiation defects in MDKO appear to be independent of Notch1, as Notch1 suppression in MDKO did not normalize the expression of these progenitor genes including Isl1. Instead, the up-regulation of Fgf8 in the MDKO might be responsible for the up-regulation of Isl1. Moreover, NFP regulation of Isl1 progenitor cells might be a stage or niche dependent manner. Mesp1-Cre, which is active earlier than Nkx2.5Cre/+, mediated NFPs deletion reduced the number of Isl1 cells, possibly due to different niches (40).
NFP regulate outflow tract morphogenesis
The OFT is a single vascular conduit that links the right ventricle to the aortic sac. The septation of OFT into the aorta and pulmonary artery ensures blood flow from the right ventricle to the lung, back to the heart and through the aorta to the whole body. Abnormal morphogenesis of the OFT, such as persistent truncus arteriosus (PTA), transposition of great arterial, double outlet right ventricle and tetralogy of Fallot, causes shunting of oxygenated and deoxygenated blood. Understanding the regulatory pathways that control SHF deployment and progenitor differentiation in the OFT is essential to the understanding of the etiology of these CHDs.
The OFT is formed by several developmentally distinct cell populations, including cardiomyocytes derived from SHF, vascular smooth muscle cells from CNCC and SHF, and endothelial cells from SHF (21). Progenitor cells from SHF migrate to the OFT and differentiate into cardiomyocytes and smooth muscle cells at the arterial pole (102). The SHF-derived myocardium gives rise to the conotruncal myocardium, which is dependent on CNCC and is critical for normal alignment of the two arteries with respect to the ventricles (27, 103). This dependence is evident when CNCC ablation results in PTA and failure of addition of SHF myocardium leads to malalignment of the arterial pole with the ventricles. The development of SHF and CNCCs are interdependent, as ablation of CNCCs results in changes in OFT length, where as loss of Fgf8 can affect both CNCC and SHF development (32, 84, 85, 103, 104).
NFP deletion via Nkx2.5Cre/+ results in OFT alignment defect, delayed OFT septation and AVSD. Wnt1-Cre, which is active in CNCC, mediated NFPs deletion, does not cause any cardiac morphogenetic defect, indicating that NFPs in CNCC are not essential for cardiac morphogenesis and are not responsible for cardiac defects in MDKO. NFPs’ deletion via Mef2c-Cre, which is active in SHF, recapitulates the morphogenetic defects in MDKO, indicating that NFPs in the SHF are essential for OFT alignment, OFT septation and atrioventricular septal morphogenesis. Cardiomyocytes in the Mef2c-Cre mediated NFPs knockouts fail to form a myocardial spike, indicating a differentiation defect (20). NFPs deletion with SM22-Cre, which is active in cardiomyocytes and smooth muscle cells at a later stage compared to Nkx2.5Cre/+, does not show defects in OFT alignment, OFT septation, or atrioventricular septal morphogenesis, which further support the notion that the morphogenesis defects in MDKO might be due to a cardiac progenitor cell differentiation defect in the SHF. SHF cells give rise to OFT myocardium and smooth muscle cells at the base of the aorta and pulmonary trunk to facilitate the separation of the aorta and pulmonary artery (26, 105). Whether NFPs are involved in the cell fate decision between cardiomyocytes and smooth muscle cells or biasing their fate during the OFT alignment/OFT septation is unknown.
NFP regulates atrioventricular septation via DMP formation
Atrioventricular septation is a complex morphogenetic process required for the formation of the four-chambered heart. There are five mesenchymal/muscular tissues involved in this atrioventricular septation: the superior and inferior atrioventricular endocardial cushions (AVC), the mesenchymal cap enveloped muscular atrial septum, the dorsal mesenchymal protrusion (DMP), and the interventricular muscular septum (Fig. 4A&B)(106). The superior and inferior AVCs derive their mesenchymal cells from the endocardium through EMT. The continued growth of this mesenchyme ensures their fusion at the AV canal to separate atria from ventricles, and divides the AV canal into mitral and tricuspid orifices. DMP derived cells proliferate and migrate through the dorsal mesocardium and bulge into the atrial chamber as a mesenchymal protrusion to reach other mesenchymal tissues (107). Simultaneously, the primary atrial septum grows from the atrial roof towards the AVC and merges with the AVC and DMP, anteriorly and posteriorly, respectively (Wessels et al., 1996; Schroeder et al., 2003; Wessels and Sedmera, 2003; Mommersteeg et al., 2006). The atrial muscular outgrowth partially septates the atrial chamber to left and right atria. Within the ventricular chamber, an interventricular muscular septum emerges between the primitive left and right ventricles from the apex and grows superiorly to fuse with AVC, dividing the ventricular chamber into left and right ventricles. These mesenchymal tissues are later muscularized to form sturdy septum, and eventually to septate the heart into a 4-chambered functional heart. The abnormal atrioventricular morphogenesis results in a variety of congenital heart defects. The AVSD in MDKO is not caused by the functions of NFP in the mesenchymal tissues, as endocardial and endothelial cell specific Tie2-Cre mediated NFPs knockouts do not display any abnormal heart morphogenesis. Instead, it might be caused by the abnormal differentiation and migration of posterior SHF progenitor cell.
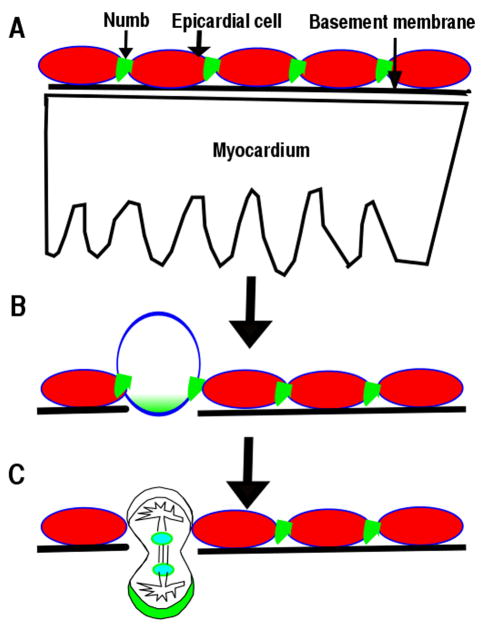
Five mesenchymal /muscular tissues contribute to atrioventricular septation. (A&B) Different views of an E12–E13 heart. There are five mesenchymal /muscular tissues including the superior (sAVC) and inferior atrioventricular endocardial cushions (iAVC), lateral AV cushions (LAVC), the mesenchymal cap (MC) enveloped muscular atrial septum (AS), the dorsal mesenchymal protrusion (DMP), and the interventricular muscular septum (VS) that are involved in this atrioventricular septation (Fig. 4A&B).
The SHF gives rise to two spatially different populations: the anterior SHF, which is adjacent to the arterial pole, and the posterior SHF, which is adjacent to the venous pole (108). The posterior SHF contributes to the DMP, an essential structure for chamber septation (109). Abnormal differentiation and development of the posterior SHF has been associated with cardiac morphogenesis defects, such as atrial septal defect and atrioventricular septal defect (AVSD) (110) (107, 111). Nkx2.5Cre/+ and Mef2c-Cre mediated NFPs mutants lack the DMP, which is required for atrioventricular septation (112). Using lineage tracing and 3D imaging, we observed that cells derived from the SHF migrated to the AVC and formed the DMP in the control, but fewer or no cells were observed in the DMP in the knockout. NFPs have been reported to be involved in cell migration via recycling of different integrin subunits to the leading edge of migrating cells (113). This recycling might be involved in progenitor cell migration in the posterior SHF to form the DMP and the atrioventricular septum. Other possible mechanisms are either that NFPs regulate Isl1 cells to differentiate to the cardiac through Hedgehog signaling, etc., or NFPs maintain the cell-cell junctions via endocytosis to regulate the morphogenesis of DMP. However, more work will be needed to determine the detailed molecular mechanisms used by NFPs to regulate posterior SHF development.
NFPs regulation of trabeculation and compaction extends beyond Notch 1 signaling inhibition
Trabeculae are sheet-like structures extending from the myocardium to the heart lumen (114). A lack of trabeculation causes embryonic lethality in mice and excess trabeculation causes cardiomyopathy and heart failure in humans (115–117). Trabecular morphogenesis is a multiple-step process including but not limited to trabecular initiation, trabecular proliferation/growth, trabecular differentiation, and trabecular compaction. Trabeculation initiates at E9.0-E9.5 (118, 119). Trabecular cardiomyocytes proliferate at a similar rate as cardiomyocytes in the compact zone at an early stage, then gradually at a lower rate during later stages. Trabecular cardiomyocytes become more differentiated than cells in the compact zone. Myocardial compaction occurs at about E14.5 and the failure of trabeculae to coalesce with the compact zone is defined as left ventricular noncompaction (LVNC, OMIM300183) (120). Disruptions of genes that code components of the sarcomere and the Z-disk cause noncompaction (121, 122). Adding a layer of complexity is the physical environment, as the hemodynamics are required for trabeculation (123).
Signaling between endocardium and myocardium such as Brg1/ADAMTS1 (124), NRG1/ErBb2,4 (125–127), EphrinB2/EphB4 (128, 129), BMP10 (130), and Notch1 are required for trabeculation. Notch1 signaling activation in endocardium regulates trabeculation in an instructing manner (131). Global or endothelial specific Notch1 deletion causes ventricular hypoplasia and trabeculation defects (131). The observation that both Notch1 loss (131) and gain of function (132) in endocardial cells reduces trabeculation indicates that mechanisms of Notch1 regulation of trabeculation are not clear.
The mechanism that regulates myocardium compaction is much less clear. One of the potential pathways is Notch2 signaling. Notch2 intracellular domain (N2ICD) is detected throughout the myocardium before E11.5, while at a later stage, Notch2 activity is specifically down-regulated in the compact zone and is restricted to trabecular myocardium during ventricular compaction (41). This indicates that Notch2 might be involved in myocardial compaction. As further evidence, Notch2 global knockout displays ventricular hypoplasia (133) and its deletion in the heart via SM22-Cre results in cyanosis at birth due to narrowed arteries. Whether or not the knockout displayed a trabeculation defect was not reported (134).
Interestingly, MDKO displays defects in trabecular initiation, trabecular growth, differentiation, and compaction, indicating that NFPs are essential for trabeculation. MDKO hearts displays a higher level of Notch1 Intracellular Domain (N1ICD). N1ICD is detected in the myocardium of MDKO but not of the control. This suggests that NFPs inhibit N1ICD accumulation in cardiomyocytes. The up-regulation of Notch signaling in MDKO is further confirmed with the transgenic mouse line that bears the canonical Transgenic Notch Reporter (TNR). This is the first in vivo evidence that NFPs inhibit Notch signaling in mammalian system. While all cardiomyocytes display up-regulation of N1ICD, only some cardiomyocytes are TNR positive, indicating that NFP deletion up-regulates both canonical and non-canonical Notch signaling. Genetic epistasis showed that Notch1 up-regulation is responsible for decreased p57 expression and increased proliferative rate in trabeculae, and increased trabecular thickness. However, surprisingly, Notch1 suppression did not rescue the defects of trabecular initiation and non-compaction (20). Another target of NFPs is Notch2. In the control, N2ICD is only present in trabeculae from E11.5 on, while N2ICD continues to be present in compact zone of MDKO. N2ICD overexpression in cardiomyocytes mediated by αMHC-Cre results in hyper-trabeculation and non-compaction, indicating that Notch2 is involved in compaction and NFPs might inhibit Notch2 to regulate compaction (41). In summary, Notch1 and Notch2 might regulate different steps of trabecular morphogenesis. We speculate that NFPs inhibit Notch1 to regulate cardiomyocyte proliferation and trabecular growth/thickness, but not trabecular initiation and compaction, and that NFPs inhibit Notch2 to regulate compaction. However, many questions regarding the regulation of trabecular morphogenesis by NFPs remain. For instance knowing how NFPs inhibit the proliferation and promote differentiation of trabecular cardiomyocytes and knowing how NFPs regulate trabecular initiation will yield a deeper understanding of the relationship between NFP and trabeculation. A broad understanding of how the signaling pathways control cellular dynamics during trabecular initiation and morphogenesis, particularly in the mammalian heart, remains to be clarified.
Conclusions and future questions
In summary, Numb is required to diversify cardiac cell types in Drosophila. In mice, NFPs play essential roles during cardiac development and cardiac progenitor cell differentiation. NFPs are required to establish epicardial polarity and epicardial cell mitotic spindle orientation to regulate epicardial cell entry into the myocardium. NFPs regulate cardiac progenitor cell proliferation early in development and differentiation at late stages. NFPs are required for outflow tract alignment and atrioventricular septation via controlling cardiac progenitor cell differentiation and migration. NFPs also inhibit Notch1 to regulate trabecular growth, and inhibit Notch2 to regulate myocardial compaction. Despite their essential functions during cardiac development, many questions still remain and prevent us from fully understanding how NFPs regulate cardiac development. The molecular mechanisms illuminating how NFPs regulate different biological processes via endocytosis are limited to the in vitro cultured system, and whether these molecular mechanisms also apply to the in vivo system is not clear. An endocytosis defective Numb mouse model should be generated to answer this question. Also, NFPs interfere with signaling pathways at post-translational level via endocytosis and recycling. The direct target of NFPs in different tissues may be distinct, which explains the various phenotypes in different tissue-specific knockouts. A high throughput screening such as quantitative proteomics will be needed to determine the direct targets in each tissue.
Acknowledgments
We thank members of the Wu laboratory for discussions and advice. We thank Dr. John Schwarz for insight comments. We thank Ernest Spiotto and Thomas Myint for editing the manuscript.
Sources of Funding
Work in the Wu laboratory is supported by Albany Medical College start-up fund, the American Heart Association (13SDG16920099 to M.W), and National Institute of Health (HL121700 to M.W.).
Abbreviations
NFPs | Numb Family Proteins |
CHDs | Congenital heart diseases |
SOP | sensory organ precursor |
FHF | first heart field |
SHF | secondary heart field |
OFT | outflow tract |
CNCC | Cardiac neural crest cells |
PEO | Pro-epicardial organ |
MDKO | Nkx2.5Cre/+ mediated myocardium Numb and Numblike double knockout |
AVSD | atrioventricular septal defect |
AVC | atrioventricular endocardial cushions |
DMP | dorsal mesenchymal protrusion |
EMT | epithelial to mesenchymal transition |
N1ICD | Notch1 Intracellular Domain |
N2ICD | Notch2 intracellular domain |
TNR | Transgenic Notch Reporter |
References
Full text links
Read article at publisher's site: https://doi.org/10.1515/bmc-2015-0003
Read article for free, from open access legal sources, via Unpaywall:
http://www.degruyter.com/downloadpdf/j/bmc.2015.6.issue-2/bmc-2015-0003/bmc-2015-0003.xml
Citations & impact
Impact metrics
Article citations
β1 integrins regulate cellular behaviour and cardiomyocyte organization during ventricular wall formation.
Cardiovasc Res, 120(11):1279-1294, 01 Sep 2024
Cited by: 1 article | PMID: 38794925 | PMCID: PMC11416060
Liquid-Liquid Phase Separation in Cardiovascular Diseases.
Cells, 11(19):3040, 28 Sep 2022
Cited by: 3 articles | PMID: 36231002 | PMCID: PMC9562871
Review Free full text in Europe PMC
Hematopoietic plasticity mapped in Drosophila and other insects.
Elife, 11:e78906, 03 Aug 2022
Cited by: 20 articles | PMID: 35920811 | PMCID: PMC9348853
Review Free full text in Europe PMC
Left Ventricular Noncompaction Is Associated with Valvular Regurgitation and a Variety of Arrhythmias.
J Cardiovasc Dev Dis, 9(2):49, 02 Feb 2022
Cited by: 4 articles | PMID: 35200702 | PMCID: PMC8876824
CTRP3 alleviates cardiac ischemia/reperfusion injury via LAMP1/JIP2/JNK signaling pathway.
Aging (Albany NY), 14(3):1321-1335, 03 Feb 2022
Cited by: 2 articles | PMID: 35114641 | PMCID: PMC8876908
Go to all (20) article citations
Similar Articles
To arrive at the top five similar articles we use a word-weighted algorithm to compare words from the Title and Abstract of each citation.
Numb family proteins are essential for cardiac morphogenesis and progenitor differentiation.
Development, 141(2):281-295, 11 Dec 2013
Cited by: 39 articles | PMID: 24335256 | PMCID: PMC3879810
The Spatiotemporal Expression of Notch1 and Numb and Their Functional Interaction during Cardiac Morphogenesis.
Cells, 10(9):2192, 25 Aug 2021
Cited by: 8 articles | PMID: 34571841 | PMCID: PMC8471136
High levels of Notch signaling down-regulate Numb and Numblike.
J Cell Biol, 175(4):535-540, 01 Nov 2006
Cited by: 62 articles | PMID: 17116748 | PMCID: PMC2064589
Numb and Numblike control cell number during vertebrate neurogenesis.
Trends Neurosci, 26(8):395-396, 01 Aug 2003
Cited by: 24 articles | PMID: 12900165
Review
Funding
Funders who supported this work.
NHLBI NIH HHS (2)
Grant ID: R01 HL121700
Grant ID: HL121700