Abstract
Free full text

Repair and tissue engineering techniques for articular cartilage
Abstract
Chondral and osteochondral lesions due to injury or other pathology commonly result in the development of osteoarthritis, eventually leading to progressive total joint destruction. Although current progress suggests that biologic agents can delay the advancement of deterioration, such drugs are incapable of promoting tissue restoration. The limited ability of articular cartilage to regenerate renders joint arthroplasty an unavoidable surgical intervention. This Review describes current, widely used clinical repair techniques for resurfacing articular cartilage defects; short-term and long-term clinical outcomes of these techniques are discussed. Also reviewed is a developmental pipeline of regenerative biological products that over the next decade could revolutionize joint care by functionally healing articular cartilage. These products include cell-based and cell-free materials such as autologous and allogeneic cell-based approaches and multipotent and pluripotent stem-cell-based techniques. Central to these efforts is the prominent role that tissue engineering has in translating biological technology into clinical products; therefore, concomitant regulatory processes are also discussed.
Introduction
The management of articular cartilage defects is one of the most challenging clinical problems for orthopaedic surgeons. Articular cartilage, a highly organized tissue with substantial durability, has a limited intrinsic healing capacity. Damage from trauma or degenerative pathology frequently results in gradual tissue deterioration, leading to debilitating joint pain, functional impairment and degenerative arthritis.1 Currently, the standard surgical intervention for end-stage degenerative joint pathology is total joint replacement. Early surgical intervention for symptomatic cartilage lesions, including osteotomy and autologous osteochondral graft transplantation, has been suggested to restore normal joint congruity and minimize further joint deterioration.2 Often, these techniques are not long-term clinical solutions, prompting the development of regenerative medicine and tissue engineering approaches to restore articular cartilage. Strategies include cell-based (with or without scaffolds) or whole-tissue transplantation techniques.
In this Review, we discuss the basic science, indications, advantages, shortcomings and outcomes of interventions for cartilage healing. The goal is to provide an evidence-based assessment for the treatment of articular cartilage pathology by reviewing the literature, with particular focus on the clinical outcomes of prospective randomized controlled trials (Table 1). Moreover, we discuss current prospective tissue engineering trials for cartilage repair, with an emphasis on cell sources, the clinical aspects of cell administration, the use of mesenchymal stem cells (MSCs), growth-factor-based therapies and cell-free, implantation-based therapies. Finally, the scientific and regulatory challenges in translating these approaches to clinical practice are discussed.
Table 1
Randomized Controlled Trials comparing cartilage regeneration techniques
Study | Treatments | Follow-up (months) | Clinical outcome | |
---|---|---|---|---|
Wong et al.65 (2013) | Bone marrow MSCs + HA + microfracture (n = 28) HA + microfracture (n = 28) | 24 | The bone marrow MSC-treated group were significantly better in IKDC (P = 0.001), Lysholm (P = 0.016), Tegner (P = 0.021) and MOCART (P <0.001) scores | Larger mean lesion size in the MSC-recipient group (6.0 cm2) than in the control group (3.5 cm2) |
Stanish et al.98 (2013) | BST-CarGel® (n = 41) Microfracture (n = 39) | 12 | MRI showed greater lesion filling (P = 0.011) and better hyaline cartilage-like MRI T2 values (P = 0.033) in the BST-CarGel® group than the microfracture group | WOMAC showed equivalent improvement in both groups |
Saw et al.77 (2013) | Subchondral drilling + HA + PBSCs (n = 25) Subchondral drilling + HA (n = 25) | 18 | PBSC group had significantly better ICRS II histological scores (P = 0.022) and MRI morphological scores (P = 0.013) | No significant difference in the mean 24‐month IKDC scores between the control and intervention groups |
Patel et al.95 (2013) | PRP (1 injection; n = 52) PRP (2 injections; n = 50) Normal saline (1 injection; n = 46) | 6 | Both PRP-treated groups had better WOMAC questionnaire results (P <0.001) than the normal saline group; a single dose of PRP is as effective as two PRP injections to alleviate symptoms in OA | The results slightly deteriorated 6 months post surgery in both PRP treated groups |
Filardo et al.100 (2012) | PRP (n = 54) HA (n = 55) | 12 | No significant differences in IKDC, EQ‐VAS, Tegner and KOOS scores between the groups | For middle-aged patients with moderate signs of osteoarthritis, PRP was nonsuperior to hyaluronic acid injections |
Gudas et al.9 (2013) | OAT–ACL (n = 34) Microfracture–ACL (n = 34) Debridement–ACL (n = 34) IAC–ACL (n = 34) | 36 | The IAC–ACL group’s IKDC subjective knee evaluation was significantly better than that of the OAT–ACL group (P = 0.043) The OAT–ACL group’s IKDC subjective knee evaluation was significantly better than that of the microfracture-ACL group (P = 0.024) and debridement-ACL group (P = 0.018) | No significant difference between the microfracture–ACL and debridement-ACL group IKDC subjective scores (P = 0.058) |
Crawford et al.49 (2012) | NeoCart (n = 21) Microfracture (n = 9) | 24 | Improvement in the NeoCart group was significantly better (P <0.05) than in the microfracture group for KOOS pain, IKDC, KOOS sports, VAS pain and the KOOS quality of life scores | Significantly more NeoCart-treated patients (P = 0.013) responded to therapy at 6, and 12 months and the trend continued at 24 months |
Bentley et al.19 (2012) | ACI (n = 58) Mosaicplasty (n = 42) | 120 | The functional outcome (Modified Cincinnati knee score and the Stanmore-Bentley functional rating system) was greater for ACI than for mosaicplasty (P = 0.02) | Repair failed within 10 years of surgery in 10 of 58 (17%) patients in the ACI group and 23 of 42 (55%) in the mosaicplasty group (P <0.001) |
Vanlauwe et al.111 (2011) | CCI (n = 51) Microfracture (n = 61) | 60 | CCI and microfracture were comparable for the KOOS and oKOOS scores; in the early treatment group CCI was statistically significant (P = 0.026) and clinically better than microfracture | Treatment failures were comparable (n = 7 in CCI vs n = 10 in microfracture), although microfracture failures tended to occur earlier |
Cole et al.88 (2011) | CAIS (n = 20) Microfracture (n = 9) | 24 | No differences in the number of adverse events (SF‐36) between CAIS and microfracture groups The IKDC and KOOS scores of the CAIS groups were significantly (P <0.05) higher than the microfracture group | Differences between the 2 groups in fill of the graft bed, tissue integration, or presence of subchondral cysts were not detected by imaging analysis |
Basad et al.20 (2010) | MACI (n = 40) Microfracture (n = 20) | 24 | MACI was significantly more effective than microfracture according to the Lysholm (P = 0.005), Tegner (P = 0.04), ICRS patient (P = 0.03), and ICRS surgeon (P = 0.02) scores | MACI was superior to microfracture in the treatment of large (>4 cm2), symptomatic articular defects |
Zeifang et al.35 (2010) | MACI (n = 11) ACI (n = 10) | 24 | No differences in the IKDC (P = 0.4994), Tegner Activity (P = 0.1043) scores and SF‐36 (P = 0.4063) of ACI and MACI techniques. Lysholm and Gillquist scores at 12 months (P = 0.0449) and 24 months (P = 0.0487) favoured ACI | MOCART revealed no significant difference between the 2 groups (P = 0.692) |
Van Assche et al.22 (2010) | ACI (n = 34) Microfracture (n = 33) | 24 | ACI had similar overall functional outcomes to microfracture | ACI resulted in slower recovery at 9 and 12 months compared with microfracture |
Abbreviations: ACI, autologous chondrocyte implantation; ACL, anterior cruciate ligament; CAIS, cartilage autograft implantation system; CCI, characterized chondrocyte implantation; EQ‐VAS, EuroQol-visual analog scale; HA, hyaluronic acid; IAC, intact articular cartilage; ICRS, International Cartilage Repair Society; IKDC, international knee documentation committee; KOOS, knee injury and osteoarthritis outcome score; MACI, matrix-assisted chondrocyte implantation; MSCs, mesenchymal stem cells; OA, osteoarthritis; OAT, osteochondral autologous transplantation; oKOOS, overall knee injury and osteoarthritis outcome score; PBSC, peripheral blood stem cell; PRP, platelet-rich plasma; SF‐36, short form‐36; WOMAC, Western Ontario and McMaster osteoarthritis index.
Present repair techniques
Microfracture
Microfracture was introduced to the clinic after other bone-marrow-stimulation techniques were used in the late 1980s and early 1990s to penetrate subchondral bone. This technique enhances migration of MSCs from bone marrow to the site of a cartilage defect (Figure 1); however, microfracture often results in the formation of fibrocartilage that is biochemically and biomechanically inferior to hyaline articular cartilage.3,4 A case series study has shown that without the mechanical robustness of hyaline tissue, the repair tissue is vulnerable to mechanical joint forces and typically deteriorates ~18–24 months after surgery, as shown by the modified Cincinnati Rating System for knee and International Cartilage Repair Society (ICRS) scores postoperatively, compared with baseline scores (Box 1).4 Such deterioration is particularly evident when treating large defects or those located in the patellofemoral joint.4 Furthermore, owing to the penetration of the subchondral bone, intralesional osteophytes develop in 20–50% of cases.5 The altered biomechanics of the sclerotic bone might be the cause of a threefold-to-sevenfold increase in the failure rate of autologous chondrocyte implantation (ACI) when performed after prior microfracture.6,7 Specific indications have, therefore, been proposed for performing this technique in cartilage lesions. These indications are based on the size, depth and location of the lesion in the joint, as well as the patient’s age and BMI (Figure 2). Although the FDA and many clinicians still consider microfracture to be the gold standard for cartilage repair, prospective comparative studies show that microfracture might delay cartilage degeneration only in the short-term; more than 5 years after surgery, treatment failure can be expected regardless of the size of the lesion.8,9 A detailed description of the indications, clinical application, rehabilitation and outcomes of the standard microfracture procedure has been reviewed elsewhere,10 and is outside the scope of this article. However, a brief understanding of this technique is important, as microfracture has become a ubiquitous approach to surgical regeneration of cartilage, the shortcomings of which have ultimately spurred the development of procedures that aim to fully regenerate articular cartilage.
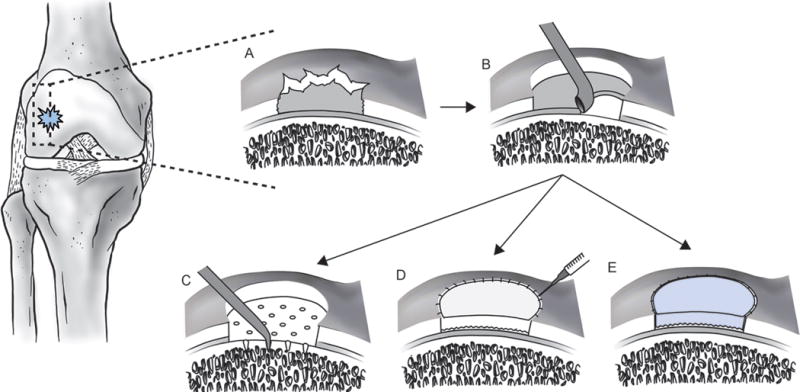
Cartilage regeneration techniques. a | A full-thickness focal chondral lesion. b | The lesion is debrided to ensure healthy, stable margins for integration of the host tissue with the neotissue. c | Microfracture. Channels are created using a 45° awl, spaced 3–4 mm apart, and 3–4 mm deep to penetrate the subchondral bone, allowing MSCs to migrate from the marrow to the cartilage defect. d | ACI. The debrided lesion is filled with 12–48 million autologous chondrocytes and covered with a periosteal flap or mixed collagen type I and type III membrane. e | MACI. The autologous chondrocyte population is expanded in vitro and then seeded for 3 days onto an absorbable 3D (collagen types I and III or hyaluronic acid) matrix prior to implantation. The cell-seeded scaffold is then secured into the lesion with fibrin glue. Abbreviations: ACI, autologous chondrocyte implantation; MACI, matrix-assisted autologous chondrocyte implantation; MSCs, mesenchymal stem cells.
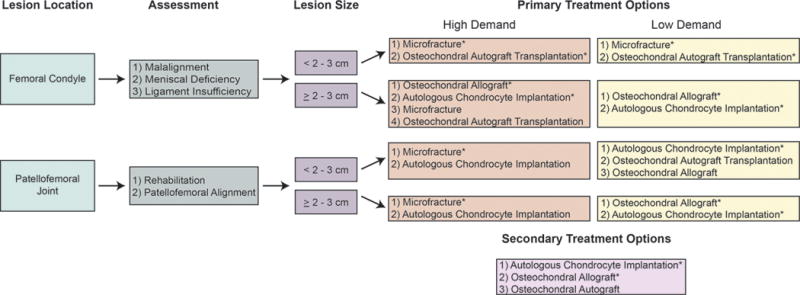
Algorithm for treatment of cartilage defects. If rehabilitation is not a viable option for a patient with a symptomatic cartilage lesion, the surgeon should assess both the size and location of the defect, determine whether the patient desires a more active (high demand) or sedentary (low demand) lifestyle, and consider if the patient has undergone previous cartilage repair treatments. *Recommended treatment options for a given situation.112
Autologous chondrocyte implantation
The inconsistent outcomes of microfracture prompted the development of ACI. To perform this technique, a full-thickness sample from a low-weight-bearing region of the joint is collected by biopsy punch during a first arthroscopic operation to provide a chondrocyte population that is then expanded in vitro, yielding ~12–48 million cells. During a second operation the chondrocytes are implanted into the debrided cartilage defect and covered by a membrane. This technique has two major benefits: using a patient’s own cells avoids potential immune complications or viral infections from transplanting allogeneic cells or foreign materials; and as opposed to autologous osteochondral implantation, the small biopsy minimizes complications for the chondrocyte donor.11,12
Positive clinical and functional outcomes of ACI have been confirmed by clinical trials.13, 14, 15 Long-term case series with >10 years follow-up have demonstrated that ACI is an effective and durable treatment for large (>4 cm2) knee cartilage lesions.13,14 Other clinical trials have reported similar results,16,17 highlighting the benefits of combining ACI with a corrective osteotomy.18 Whether ACI is superior to other standard treatments in prospective randomized controlled clinical trials, however, is controversial. A study comparing ACI to autologous osteochondral transplantation (mosaicplasty) found ACI to have superior clinical results 10 years postoperatively;19 other studies demonstrated superior outcomes for ACI when compared with microfracture for the treatment of cartilage defects >3 cm2 in size.20,21 Studies of ACI and microfracture for smaller defects, however, have found similar clinical and radiographically evident outcomes,22 although ACI has been associated with improved structural repair.12,23 Additional large-scale prospective randomized studies with a long-term follow-up are necessary to identify and understand the clinical criteria, indications and contraindications for when ACI might provide superior clinical outcomes over less invasive and costly techniques, such as microfracture. Ideally, functional evaluation should be supplemented by characterization of neotissue with sophisticated imaging or arthroscopic techniques.
ACI has three major drawbacks: two operations are needed; a long recovery time (6–12months) is required to ensure neotissue maturation and achieve improved clinical scores from baseline; and ACI is a multistage, complex procedure. The most frequently reported adverse event after ACI, using a periosteal flap to seal the implanted cells in the cartilage defect, is hypertrophy of the flap.24 Therefore, alternative approaches utilize artificial matrices such as porcine membranes consisting of mixtures of collagen types I and III or hyaluronic acid scaffolds.25–27 However, these materials increase the likelihood of an immune reaction to the allogeneic scaffold, and their use is currently considered off-label in the USA. Preliminary studies have also shown that autologous chondrocytes ‘dedifferentiate’ into fibrochondrocytes in culture.28 However, other work shows they can redifferentiate and express chondrocytic markers after being reintroduced into a 3D in vitro culture system.29 To standardize clinical outcomes of ACI, patient-specific and cartilage defect-specific factors have been established to generate a treatment algorithm that improves the likelihood of a successful outcome (Figure 2). Nevertheless, large-scale cohort studies are needed to further investigate the cost-effectiveness of ACI.
Overall, ACI has proven beneficial for treating cartilage defects, but more research needs to be done to develop biomechanically stable matrices, to achieve faster maturation of the neotissue and better integration with the host tissue. Furthermore, work is required to standardize and optimize the technique and the post-surgery functional evaluation so that surgeon and patient biases do not affect the functional outcome.
Scaffold-based techniques
The lack of a supportive scaffold material to guide matrix synthesis and organization might, in part, account for the outcome variability across patient populations treated with chondrocyte implantation techniques. Ex vivo studies have shown that successful cartilage regeneration is dependent both on the chondrocyte proliferation rate and on the differentiation capacity of stem cells within a tissue-engineered 3D matrix, a structure that acts as a cell carrier to maintain and support the crucial characteristics of the tissue.30 The second generation of ACI has therefore focused on the development of scaffold-based approaches for delivering chondrocytes to the cartilage defect site. Scaffold-based approaches have major advantages to scaffold-free techniques: these advantages include, increased control to better fill the cartilage defect; fewer donor site complications; a less technically challenging procedure; and shorter postoperative recovery time due to increased graft stability. Additionally, because the chondrocytes are cultured in a 3D environment, they are less prone to dedifferentiation and therefore produce a more hyaline-like cartilage.31 Furthermore, in vitro culture prior to implantation might help to maintain quality control of scaffold-based repair.
MACI
Matrix-induced autologous chondrocyte implantation (MACI) is the most common scaffold-plus-cell-based cartilage repair technique currently in clinical practice. Similar to ACI, MACI requires two surgical procedures. The first surgery collects autologous tissue from which the patient’s chondrocytes are isolated. Once isolated, the cell population is expanded in vitro and then seeded for 3 days on an absorbable porcine-derived mixed collagen (type I and III) membrane prior to implantation. These membranes are specifically engineered to promote chondrocyte infiltration on one side and lubrication on the other. To implant the cell-seeded scaffold a mini-arthrotomy exposes and debrides the lesion, after which the matrix is implanted and secured using fibrin glue, with the cell-laden side facing the subchondral bone and the low-friction surface facing the joint cavity (Figure 1).
Although case series of MACI have had promising clinical and histological results,32,33 the superiority of MACI over existing techniques remains unproven. One of the few prospective randomized clinical trials to assess MACI found comparable clinical, arthroscopic and histological outcomes between MACI-treated or ACI-treated patients;34 however, the MACI-treated group had a lower rate of hypertrophy of the graft. Another study reported no differences in International Knee Documentation Committee (IKDC) scores, Tegner activity scores or the Short Form‐36 from patients 24 months after undergoing either MACI or ACI; however, Lysholm and Guillquist knee functionality scores showed better efficacy of the ACI technique than MACI (Box 1).35 A separate study found outcomes to be better with MACI than microfracture for patients with large (>4 cm2) defects 2 years after surgery.20 Overall, these studies found MACI to have similar, if not superior, functional outcomes to either ACI or microfracture in a follow-up study after ≥2 years. Although MACI is technically attractive in terms of reproducibility, safety, surgical simplicity and reducing invasiveness and intraoperative time, the expense of the technique warrants further investigation. Large prospective randomized trials with long follow-up periods are also necessary to identify clinical criteria, indications and contraindications to optimize the performance of this technique.
Hyaluronan-based scaffolds
Hyaluronan-based scaffolds are another means of implanting chondrocytes within a 3D biodegradable environment. Similar to collagen-derived matrices, the hyaluronic-acid-based scaffold is intended to promote and maintain the chondrocytic phenotype and collagen type II synthesis during in vitro culture, as well as after implantation (Figure 1).36 Although, ex vivo studies have reported that the degradation rate of these materials can be tailored to match the rate of the synthesis of extracellular matrix (ECM) by the cells to aid in integration with the defect site,36,37 this finding has not been confirmed in long-term follow-up clinical studies.
However, treating cartilage defects with autologous chondrocytes delivered with a hyaluronic-acid-based scaffold has shown promise. In case series, hyaline-like cartilage has been shown to develop ~1 year after implantation,27,38 and improved functional and health-related quality of life outcomes have been reported 3 years after implantation, compared with baseline.27 A prospective cohort study, evaluating athletes treated with either hyaluronic-acid-based MACI or microfracture, found comparable outcomes in ICRS, IKDC and Tegner scores (Box 1) between the two groups 2 years postoperatively.38 However, better IKDC scores were detected 7.5 years after operation in the hyaluronic-acid-based MACI group than in the group treated with microfracture. Interestingly, athletes treated by microfracture required at least 8 months of recovery before returning to the field, whereas those receiving hyaluronic-acid-based MACI required 12.5 months.38 Thus, although hyaluronic-acid-based MACI ultimately has better clinical outcomes than microfracture, longer rehabilitation is required. In a small cohort study, statistically significant differences were not found in the functional outcomes or the histological and immunohistochemical composition of hyaluronic-acid-based and fibrin-based scaffolds (both seeded with chondrocytes) 1 year after implantation.39 Although scaffold-based ACI for cartilage regeneration is approved in Europe, and FDA approval is currently pending in the USA, well-designed comparative studies with long follow-up periods are needed to confirm the potential therapeutic and economic benefits.
Future regenerative approaches
Ex vivo chondrocyte-seeded scaffolds
Despite the technical advantages of MACI, clinical studies have revealed the functional outcomes to be similar to those of ACI.34,35 A plausible explanation is that after chondrocyte seeding, the collagen matrices are implanted into the patient within 3 days, leaving the MACI neotissue implant immature and therefore vulnerable. To improve the efficacy of such tissue engineering procedures, novel approaches are in development (Figure 3) to introduce autologous chondrocytes into a 3D matrix and culture them in vitro for longer periods, typically 4–6 weeks. During this time, articular chondrocytes produce their own ECM components within the 3D environment, resulting in an implant with biochemical integrity similar to healthy articular cartilage. Surgical techniques similar to those established for MACI are used to engineer and implant the tissue. The enhanced mechanical robustness of the neotissue after long-term ex vivo 3D culture makes implants structurally and functionally more mature than MACI-based implants and, thus, potentially able to withstand normal joint loads earlier post-implantation. This robust neotissue might improve short-term and long-term functional outcomes for patients, and ex vivo and preclinical outcomes are promising,40–42 although comparative clinical studies are needed to confirm this hypothesis. Also imperative is to investigate methods to form a stable interface between the implant and host tissue environment; a low integration potential raises the failure rate of the technique.43
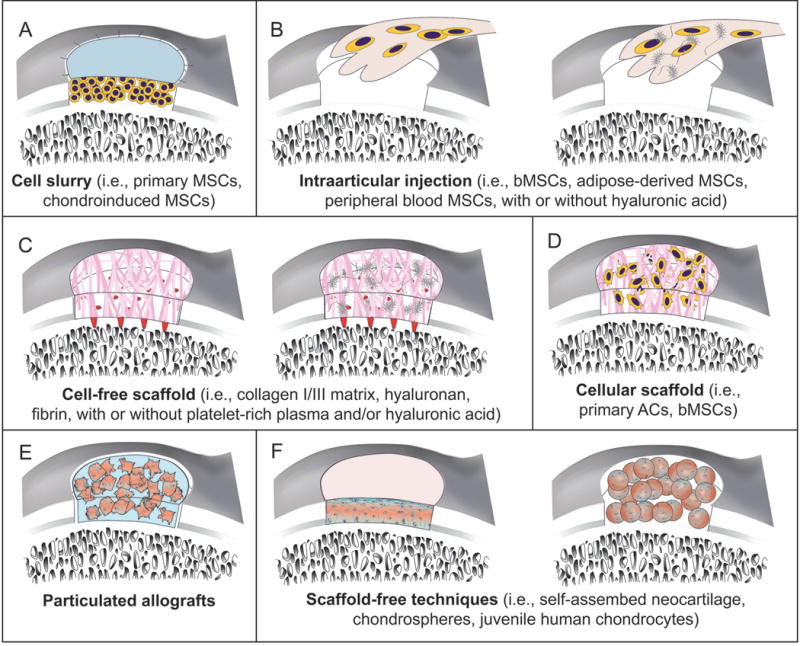
New tissue engineering techniques for treating cartilage lesions. a | Technically mimicking ACI, a debrided chondral lesion is filled with bone-marrow-derived or chondroinduced MSCs, not autologous chondrocytes, and covered with a collagen type I/III membrane. b | Intra-articular injection of MSCs with or without injectable matrices is a single-stage procedure. c | AMIC is a cell-free, scaffold-based single surgery. Microfracture releases blood and bone marrow MSCs, then collagen type I/III, hyaluronic acid or fibrin matrix are sutured or glued into the defect. d | MACI uses scaffolds plus either primary articular chondrocytes or bone-marrow-derived MSCs. e | Neotissue can be formed by combining particulated native cartilage with fibrin glue. Limited autodigestion of ECM releases superficial chondrocytes, which then produce additional ECM that integrates the cartilage particles and fills the defect. f | Scaffold-free techniques include a self-assembling process or chondrospheres. Without a scaffold to interrupt cell–cell signalling and stress shielding, cells are able to respond to stimuli and promote integration of neotisssue ECM with the surrounding tissue. Resulting neotissue is thought to be a bioactive microenvironment. Abbreviations: ACI, autologous chondrocyte implantation; AMIC, autologous matrix-induced chondrogenesis; ECM, extracellular matrix; MACI, matrix-induced autologous chondrocyte implantation; MSCs, mesenchymal stem cells.
Although in vitro culture of autologous chondrocyte-seeded 3D matrices might result in robust chondral implants, time alone is not enough to promote sufficient maturation of the engineered tissue. Researchers have therefore sought exogenous chondrocyte stimuli analogous to those that promote biomechanically robust implants in vivo. Walking, for example, can create loads of ~4–5 times body weight on normal human knee cartilage.44 Chondrocytes respond to high mechanical loads by proliferating and producing ECM.45 Thus, exogenous mechanical stimulation has been applied to cell-laden matrices in vitro, in the form of either hydrostatic pressure40 or dynamic compression,41 to improve matrix maturation and the function of neotissues. Research has also identified growth factors that help chondrocytes to maintain their phenotype and ability to produce ECM. The most important growth factors widely used in cartilage regeneration and tissue engineering ex vivo studies are transforming growth factor β (TGF‐β) family members, particularly TGF‐β1 and TGF‐β3.46 In vitro tissue engineering studies have confirmed that TGF‐β stimulation helps maintain the native chondrocyte phenotype, enhances the biochemical composition and functional properties of the neotissue, and promotes the development of implants that exhibit structure–function relationships similar to native articular cartilage.40,41,47 Despite extensive research in this field, there is no general consensus regarding the optimal type, level and application time of growth factor and mechanical stimulation, or for combinations of these factors, to develop robust hyaline-like tissues. The array of treatment possibilities complicates and thereby increases the cost of research in this field.
Only a few human clinical reports on the outcomes of autologous chondrocyte-laden, engineered scaffold-based approaches to cartilage regeneration are available. In an FDA phase I clinical trial, eight patients were implanted with tissue-engineered, autologous-chondrocyte-seeded, collagen type I scaffolds, which were subjected to hydrostatic pressure prior to surgery.48 After 1 year, cartilage defects in seven of eight patients had almost completely filled with a mature, organized repair-tissue. These promising results led to a phase II clinical trial, which found that the technique had a similar safety profile as microfracture and had better clinical outcomes in most functional scores 2 years after implantation.49 Although immune reactions to the collagen scaffold were not found in this study, long-term studies should evaluate this potential.
Alternative cell and tissue sources
Current cell-based clinical practices for treating articular cartilage defects and degeneration only utilize autologous chondrocytes. Despite efforts to recapitulate the in vivo environment with 3D culture, bioreactors, and exogenous mechanical and biochemical stimulation, a repair tissue with native hyaline cartilage properties has yet to be produced. Thus, in an effort to avoid some of the drawbacks associated with autologous chondrocyte therapy, methods have developed using stem cells, many of which are currently in a queue for FDA approval for clinical studies.
Bone marrow MSCs
Of the various types of stem cell in the body, MSCs derived from bone marrow have many advantages for clinical use. They are comparatively easy to isolate and proliferate, allowing for the potential to obtain them from donors and to store them in readily available cell banks. Furthermore, extensive preclinical work in vitro and in vivo confirmed by clinical trials has found that human bone marrow MSCs are capable of differentiating into both cartilage50–53 and bone,54 meriting their use for regeneration of both osteochondral and chondral defects. Specifically, bone marrow MSCs derived from patients with advanced osteoarthritis were shown ex vivo to produce proteoglycan and collagen type II and to maintain a chondrocytic phenotype in a scaffold in the presence of TGF‐β1.55,56 Another beneficial trait is their ability to selectively migrate to diseased tissues and organs, where they have been found to secrete T‐cell-suppressive cytokines57,58 known to be important for allotransplantation and xenotransplantation.59 In the case of cartilage repair, bone marrow MSCs might enable a targeted repair system that promotes trophic effects through the release of synthetic, proliferative and regenerative factors directly into chondral lesions.60 By creating a regenerative environment with the release of chemotactic factors,61 bone marrow MSCs might also drive endogenous stem cells to enter the cartilage defect and aid in the regeneration of damaged tissue.
Preliminary human clinical studies have investigated the cartilage regenerative potential of injected human bone marrow MSCs (Figure 3). In a case series, four patients with osteoarthritis were treated each with a single injection of bone marrow MSCs into the knee, 1 year after which the patients had decreased pain and improved joint mobility without any adverse effects.62 Good clinical and functional results were also reported in a similar case series at a 6‐month follow-up.63–65 Despite the potential application of bone marrow MSCs, much remains to be investigated: such as, the number of cells required to promote cartilage regeneration; whether one or more injections are required to reach the desired effect; the optimal time-window for these injections; and the long-term safety of the technique. Finally, prospective controlled studies with long-term follow-up are needed to investigate the therapeutic potential of bone marrow MSC injection versus established cartilage repair techniques.
ACI-like and MACI-like strategies can use bone marrow MSCs in conjunction with scaffolds, as opposed to autologous chondrocytes (Figure 3). Many clinical case series have used cultured bone marrow MSCs seeded into collagen scaffolds for the treatment of symptomatic cartilage defects.52,66,67 Most of these studies, which have been with a small number of patients, have reported good results less than 1 year after implantation. In a large-scale observational cohort study, 72 patients with cartilaginous lesions were treated with either ACI or bone marrow MSC implantation covered with a periosteal patch.53 The stem cells were found to treat the chondral defects as effectively as ACI in terms of 2‐year postoperative clinical and functional outcomes. More importantly, the bone marrow MSC treatment was less expensive than ACI, avoided the risk of donor site morbidity and did not require an additional operation to obtain the cells. However, pain from the surgical site from which bone marrow MSCs were harvested, along with other potential adverse effects of the additional surgical intervention, are concerns meriting further investigation.
Overall, the positive outcomes of these studies accentuate the potential of bone marrow MSC therapy for cartilage repair. However, it remains to be seen how these techniques compare with more established procedures in comparative clinical studies, and further research is necessary to understand the feasibility of their clinical application. The potential benefit of preimplantation differentiation of bone marrow MSCs with growth factors or other biophysical or biomechanical stimuli that promote phenotypic change and stability also warrants additional investigation. As MSCs are multipotent, studies to confirm the safety of transplanting them are also needed. Finally, future studies should directly compare bone marrow MSCs with other stem cells for their ability to regenerate cartilage.
Other MSC sources
Most cartilage regeneration work using MSCs has been with bone-marrow-derived cells. Bone marrow aspiration, although painful, is an established method. However, stem cells can be derived from other sources, including adipose tissue, muscle, corticocancellous bone, synovium, periosteum and umbilical cord.68 Human adipose-derived stem cells are the most readily accessible, and in vitro studies have shown these cells to have a similar proliferative profile and differentiation capacity to bone marrow MSCs.69,70 Adipose-derived stem cells have been regarded as an ‘ideal’ cell source due to our ability to isolate them in comparatively large quantities, their nonimmunogenic and anti-inflammatory properties,71 minimal ethical considerations associated with their use and, compared with stem cells from other sources, their capacity for proliferation and differentiation is less likely to be affected by a person’s age.72 The clinical feasibility and safety of human adipose-derived MSCs to treat degenerative cartilage pathology was investigated in a case series by injecting the cells into the osteoarthritic knee joint of elderly patients (mean age 70.3 years, range 65–80 years) in combination with arthroscopic lavage.73 The treatment resulted in improved physical function, cartilage healing and pain reduction; however, this technique has some of the same problems as bone marrow MSC joint injections: these problems include the number of cells required to promote cartilage regeneration; whether one or more injections are required to reach the desired effect; the optimal time-window for these injections; and the long-term safety of the technique. In addition, quantitative evidence of improvement, for example with biopsy or MRI, is needed.
The chondrogenic potential of stem cells from other sources has also been studied; autologous peripheral blood stem cells (PBSCs) have been the most clinically investigated, albeit primarily in case series.74–76 A randomized trial investigated articular cartilage regeneration in patients aged 18–50 years with knee chondral lesions (ICRS grade 3 and grade 4 lesions) treated with arthroscopic subchondral drilling followed by postoperative intra-articular injections of hyaluronic acid, with or without PBSCs. The study found the PBSC group had improved quality of cartilage as shown in the ICRS visual assessment scale histology score and an MRI-based morphological score over those of the control group 18 months after injection.77 In vitro, synovial-derived stem cells have a better chondrogenic potential than stem cells from other sources,78,79 as shown by the development of hyaline-like neotissue when they are seeded onto scaffolds,80 although clinical studies using these cells have not yet been conducted. Multilineage dermal stem cells have also been isolated and have a high capacity for in vitro chondroinduction in a cartilage tissue engineering study.81 Much in vitro work remains to be done to understand the chondrogenic potential of stem cells and to optimize chondrocyte differentiation. Future preclinical and comparative clinical studies will hopefully answer the many questions concerning the benefits, clinical applicability and cost-effectiveness of these cells, and they might identify indications and criteria for their use in cartilage healing.
Induced pluripotent stem cells
Induced pluripotent stem cells (iPSCs) also show promise for chondrogenic application in the clinic. These cells have the ability to differentiate in vitro into either cartilage51,82 or bone.83,84 The expression of chondrogenic markers in human iPSCs was shown to be either comparable or superior to bone marrow MSCs,51 whereas the quality of cartilage repair tissue formed by either human iPSC pellets or a human iPSC–alginate hydrogel in a rat osteochondral defect model was substantially better than the tissue formed in empty defects or defects filled with alginate hydrogel.51,82 In an in vitro study, iPSCs derived from reprogrammed human synovial cells of patients with advanced osteoarthritis were used to generate mesenchymal lineage cells, such as chondrocytes and osteoblasts.84 Despite these promising results, several questions remain to be answered: these questions pertain to the chondrogenic efficacy of these cells; optimal practices for isolating, differentiating and purifying them ex vivo; the undesired genomic modifications associated with most reprogramming protocols, and the potential of teratogenesis or other in vivo tissue malformations; and validation of iPSC-based therapies by the FDA and the European Medicines Agency. Although much remains to be understood, the enormous potential of iPSCs for treating cartilage degeneration merits future studies to address these uncertainties.
Fresh and particulated allografts
Aside from cell-based methods, fresh osteochondral allografts, as well as particulated cartilage allografts, have been used to fill cartilage defects and promote regeneration (Figure 3). An advantage of using allograft tissues is that they are not regulated by the FDA, and thus bypass costly and time-consuming clinical trials. Fresh osteochondral allograft transplantation has had positive clinical benefits for young, active individuals. Clinical results have found that these grafts last 1–25 years, depending on graft chondrocyte viability and mechanical stability of the host–graft interface; when such criteria were met, histological evaluation consistently detected hyaline repair tissue.85
Particulated cartilage allografts, on the other hand, are the focus of fewer scientific publications. These grafts are formed by combining particulated cartilage with a fibrin glue to form a construct that is then placed into the cartilage defect. Limited autodigestion of the ECM releases superficial chondrocytes, which produce additional ECM that integrate the cartilage particles, filling the defect. Clinical experience with this technique is limited to short-term (2-year follow-up) studies, most of which are case reports and case series demonstrating feasibility, safety and efficacy, with improvements in subjective patient clinical scores and MRI evidence of good defect filling.86,87 An alternative approach to introducing autologous cartilage fragments into the cartilage defect utilizes a scaffold-based technique. Specifically, in a single surgery, hyaline cartilage is arthroscopically harvested from a low load-bearing region of the joint, mechanically minced and then glued onto a synthetic, absorbable scaffold before transplantation into the cartilage defect. In a prospective clinical safety study, this novel autologous scaffold-based allograft procedure was compared with microfracture; clinical and imaging results showed the scaffold-based approach resulted in better functional outcomes, better cartilage development and fewer adverse events.88 A larger prospective controlled study investigating the mechanical integrity of neotissue formed with this technique, by quantitative MRI techniques and second-look arthroscopy, was stopped by the sponsor due to the difficulty in adhering to strict FDA inclusion criteria.113 Future studies should correlate structural changes (as measured by quantitative MRI techniques or established morphological methods) with either clinician-based evaluation scales or patient-reported outcome assessment tools.
Novel cell-free biomaterials
Although autologous chondrocytes and MSC-based techniques offer much promise for articular cartilage repair, methods for extracting, proliferating and differentiating cells are both timely and costly. Furthermore, because MACI-based and hyaluronic-acid-scaffold-based techniques are cellular, the FDA might consider them both as a medical device and as biological, resulting in long and expensive regulatory approval. These regulatory advantages thereby enhance interest in these cell-free material-based products for cartilage regeneration and repair.
AMIC
Autologous matrix-induced chondrogenesis (AMIC) is a cell-free technique that can be performed in a single surgery (Figure 3). To perform AMIC, a mini-arthrotomy exposes and cleans the defect site, then microfracture releases both blood and bone marrow containing MSCs, and finally a mixed collagen type I and type III matrix is sutured or glued into the cartilage defect.89 The implanted collagen matrix is thought to stabilize the resulting blood clot, helping to promote early mechanical stability and cartilage regeneration. Case series have found AMIC to be both safe and effective in treating full-thickness cartilage defects.90–93 In one study, patients followed-up for as long as 5 years had substantial improvements in Tegner, Lysholm, ICRS, and Cincinatti scores (Box 1) as early as 12 months after surgery.92 Furthermore, MRI revealed moderate-to-complete filling of all chondral defects. In another AMIC study, a biphasic osteochondral biodegradable scaffold consisting of calcium triphosphate in the osseous region and poly(lactic-co-glucolic acid) in the cartilaginous region was used to treat full-thickness ankle cartilage defects.93 At a 1 year follow-up, American Orthopaedic Foot & Ankle Society and Ankle Osteoarthritis Scale clinical scores (Box 1) were increased in all six patients compared with preoperative values. Interestingly, the observed clinical outcomes in AMIC-treated patients did not correlate with age, BMI or the number of previous orthopaedic operations of these patients. The small number of cases in these studies, the short follow-up and not having control groups might explain the lack of such correlations. The simplicity and cost-effectiveness of AMIC merits further studies to identify the quality of neocartilage formation, the reproducibility of the data and any potential pitfalls associated with the technique.
Active in situ approaches
A novel approach to enhance cartilage repair with AMIC is to deliver growth factors that selectively recruit and stimulate MSCs from the subchondral bone marrow into cell-free scaffolds. Such growth factors can be tailored to also activate chondrocytes in the surrounding healthy tissue to help remodel the repair tissue filling the cartilage defect.
Implanting polymer-based materials combined with autologous serum or platelet-rich plasma and hyaluronic acid after initial microfracture of a defect has been proposed as a novel strategy for active in situ AMIC.94 Autologous serum and platelet-rich plasma can recruit bone marrow MSCs from the underlying subchondral bone,95 and hyaluronic acid might help the bone marrow MSCs differentiate into a chondrocytic phenotype.114 A clinical trial found 52 patients receiving this treatment had improved patient-reported Knee injury and Osteoarthritis Outcome Scores (KOOS) 1 year after surgery, compared with their preoperative status (Box 1), and defects were shown, by histological analysis, to be filled with a hyaline-like repair tissue.96 However, controlled trials with a longer follow-up period are needed to confirm these data and prove that the neotissue is durable. In another method, chitosan in a glycerol phosphate buffer is mixed with freshly drawn autologous whole blood to form a clot.97 A multicentre, randomized comparative clinical trial found that 1 year after operation a combined treatment of chitosan–glycerol phosphate and microfracture resulted in greater lesion filling and a superior quality of repair tissue than microfracture alone.98 However, no significant differences in the functional outcomes were detected between the groups at follow-up. Given that fibrocartilage formed after microfracture starts to deteriorate as soon as 2 years after surgery,4 longer follow-up studies of the functional outcomes are needed to investigate the potential benefits of these active in situ AMIC techniques.
Knowledge of the dose dependence and tissue specificity of MSC chemoattractants, including bone morphogenetic proteins, whole blood serum, chemokines and platelet-rich plasma could be used as part of an active in situ AMIC cartilage repair strategy.99,100 Potentially, these treatments could be tailored for a specific condition and anatomical location. Although most of these treatments are FDA-approved and are immediately available for clinical application, well-designed preclinical studies followed by controlled clinical trials are first needed to investigate their effectiveness. Additionally, growth factors belonging to the TGF‐β superfamily, as well as insulin-like growth factors, platelet-derived growth factors and pro-epidermal growth factor, have been shown to enhance stem cell chemotactic migration.101 Methods could therefore be developed to combine both growth factors and MSC chemoattractants to further promote cartilage regeneration by tailoring their release in a controlled manner, for example on the surface of matrices or within nanoparticles.102
Scaffold-free approaches
Self-assembling process
Although several tissue engineering approaches for cartilage repair forgo the use of either cells or exogenous stimulation, another option is a self-assembling process that requires no scaffold (Figure 3).103 Without a scaffold to interrupt cell–cell signalling and stress shielding, the neotissue microenvironment might be more bioactive, enhancing the response to stimulation and integration with surrounding tissue (Box 2). Ex vivo studies found that stimulation of self-assembled articular cartilage with exogenous (hypoxia, growth factors, crosslinking agents) and biomechanical (hydrostatic pressure) stimulation promotes the development of robust neotissue with similar functional properties as native cartilage.104–106 These studies also found that scaffold-free neotissue is capable of both integration and in vivo maturation.107 When compared with scaffold-based techniques, the scaffold-free neotissue had superior mechanical properties and a higher percentage of ECM.108 Thus, scaffold-free neotissue is a promising new development in the generation of highly bioactive implants to enhance cartilage repair.
Chondrospheres
Another scaffold-free technology for articular cartilage regeneration is based on generating spheroids of autologous chondrocytes (chondrospheres) for implantation (Figure 3). In a minipig cartilage defect model, these spheroids adhered and integrated with full-thickness cartilage defects and produced cartilaginous ECM.109 The clinical efficacy of chondrospheres is currently being investigated in a phase III controlled clinical trial in Europe;115 the preliminary human clinical outcomes have not yet been published. Another scaffold-free technology is to utilize juvenile allogeneic chondrocytes. These cells have been shown to have a stronger and more stable chondrogenic activity in vitro than human adult cells and can avoid immunological responses and form hyaline-like tissue when grafted into goat cartilage defects.110,111 A phase III clinical trial comparing this technology with microfracture is ongoing; clinical data from this trial are not yet available.
Conclusions
This Review focuses on the basic science, indications, advantages, limitations and outcomes of current and future cartilage regeneration strategies. Emphasis is on tissue engineering approaches because such techniques are the basis for the next generation of cartilage regeneration technology. Specifically, tissue engineering aims to develop biomimetic tissues that recapitulate the biological, structural and functional features of native cartilage, increasing the ability of implants to withstand and adapt to the highly loaded environment of the knee. Such strategies are needed, not only stop the progression of cartilage degeneration, but also to improve long-term functional outcomes for patients. These novel biomimetic solutions might help treat an increasing number of individuals with cartilage pathology, as well as limit the economic burden.
Despite the efforts discussed, the generation of truly biomimetic chondral and osteochondral replacements is elusive; however, technological development in many areas is rapid (Figure 4). For example, tissue engineering approaches using a variety of cell sources, including autologous, allogeneic, xenogeneic and stem cells, have yielded repair tissues with hyaline-like characteristics, thereby decreasing pain and in many cases delaying joint degeneration. Although a ‘gold standard’ cell source has yet to be identified, stem cells stand out in terms of their availability and minimization or lack of donor morbidity. Furthermore, the combination of tissue engineering with both biochemical and biomechanical exogenous stimulation has resulted in repair tissues with even better hyaline-like properties. Tissue-engineered, cell-free scaffolds, as well as cell-based, scaffold-free approaches offer even further hope for the treatment of cartilage repair.
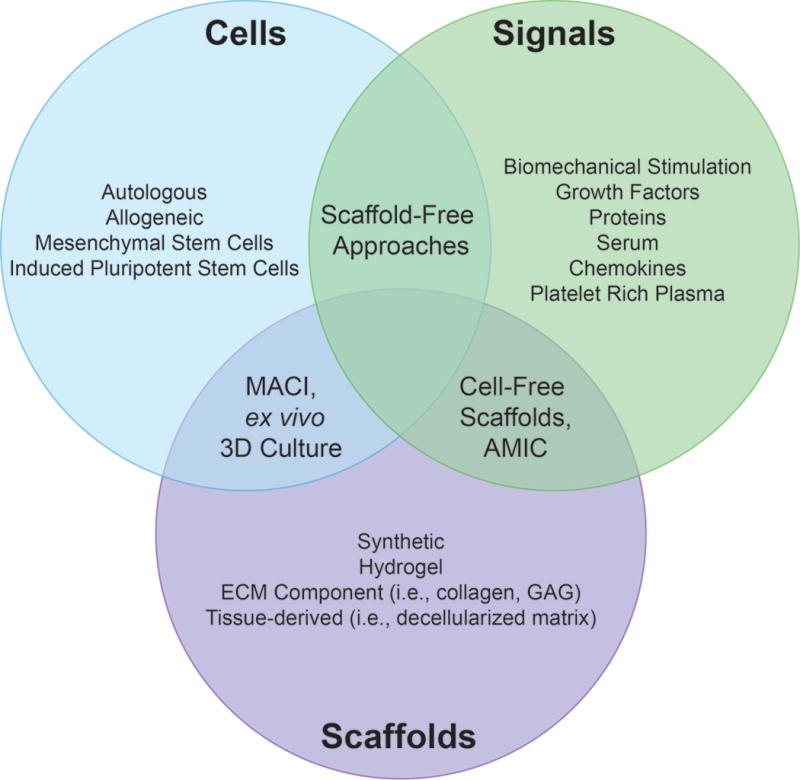
The tissue engineering paradigm. Cells, signals and scaffolds are the major elements of tissue engineering approaches to cartilage repair. For this purpose, many different cell sources (autologous cells, allogeneic cells or stem cells) have been tested in vitro. Neotissue has been cultured ex vivo with various stimuli and chemicals to enhance synthesis and chondrogenic potential. To further improve the integrity of neotissues, scaffolds have been used to create a 3D environment to maintain the phenotype of, and carry, integrated cells in vivo, and to recruit cells from the host environment. Combinations of these factors drive the three major cartilage engineering strategies that exist: cell-free, scaffold-based implants that promote cell recruitment with chemoattractants; cell-seeded scaffolds that mimic the structure of native tissues; and scaffold-free, cell-based biomimetic techniques. Abbreviations: AMIC, autologous matrix-induced chondrogenesis; ECM, extracellular matrix; GAG, glycosaminoglycans; MACI, matrix-assisted autologous chondrocyte implantation.
Overall, a technology has yet to be developed that satisfies the fundamental requirements of successful cartilage healing, namely, one that embodies the appropriate structure–function relationship, ECM organization, bioactivity and surgical logistics. To address these criteria, work must be done to improve not only the mechanical properties of the tissue, but also to shape and organize neotissue development so that it better mimics native morphogenesis. Furthermore, emphasis must be placed on the degradation characteristics of scaffold-based approaches to ensure degradation rates match tissue regeneration. In cell-based applications, a thorough understanding of how to maintain the chondrocytic phenotype of autologous and allogeneic chondrocytes, and how to foster complete chondrogenic differentiation of stem cells, must be established. Once technologies are able to pass these criteria, stringent manufacturing methods that ensure reproducibility of the resulting implants and long-term functional clinical outcomes in humans will need to be developed. Standardization of the approval process should also be streamlined across countries; current practices in Europe allow faster translation of promising technologies than the FDA.
Crucial to these endeavours is the development of better animal models of cartilage damage for in vivo testing prior to clinical application in well-designed, prospective, randomized controlled clinical trials. Furthermore, such clinical studies should compare novel technologies to current ‘gold standard’ clinical approaches. The resulting repair tissue must also be subjected to rigorous biochemical and biomechanical evaluation to determine how similar the regenerative tissue is to native tissue. What quantifies a robust, hyaline-like repair tissue, and what threshold of such quantifiable characteristics is necessary for successful clinical outcomes must be clearly determined. Use of noninvasive, quantitative measures both before and after surgery is important to understand long-term clinical trial outcomes. Such information will be critical to determine which technologies result in regenerative tissue that truly recreates the structure–function relationship of healthy, native articular cartilage. Equally important, measures should be taken to determine the appropriate indications, contraindications, patient selection criteria, surgical practices and rehabilitation protocols for each technology. Importantly, the necessity of minimizing health-care costs must also be seriously considered. All-in-all, much work is required to develop truly biomimetic cartilage regenerative therapies, but we are closer than ever to making such a concept a clinical reality.
Acknowledgments
The authors acknowledge funding from NIH R01 AR061496, NIH R01 DE019666, NIH R01 DE015038, and California Institute for Regenerative Medicine (CIRM) TR3‐05709.
Biographies
Dr Eleftherios A. Makris gained his medical degree in 2009 from Aristotle University (Medical School), Thessaloniki, Greece. In 2014 he completed his PhD in biomedical engineering, focusing on developing tissue-engineering methods for cartilage regeneration. He has published more than 10 papers focused on cartilage regeneration and tissue engineering. Translating new cartilages technologies to clinical practice is one of his main research interests.
Dr Andreas H. Gomoll received his medical degree from Ludwig-Maximilians Universität in Munich, Germany in 1996, and his PhD in 1997 from Technische Universität, Germany. Currently, Dr Gomoll is on staff at Brigham and Women’s Hospital in Boston, USA, and he is Associate Professor of Orthopedic Surgery at Harvard Medical School. He specializes in reconstructive procedures for cartilage defects and early arthritis, such as cartilage and meniscal transplantation, and osteotomy.
Dr Konstantinos N. Malizos gained his medical degree in 1978 from Aristotle University (Medical School), Thessaloniki, Greece. Since 1998 he has served as the Chairman of the Department of Orthopedics and Musculoskeletal Trauma at the Medical School, University of Thessaly, Greece. He has published more than 200 papers in peer-reviewed journals and is International Deputy Editor of the Journal of Bone & Joint Surgery.
Dr Jerry C. Hu holds a doctoral degree in Bioengineering form Rice University, USA. He has published more than 40 papers. Since 2000 Dr Hu has focused on the development of cartilage tissue engineering approaches, pioneering the development of the self–assembling process in articular cartilage.
Dr Kyriacos A. Athanasiou holds a doctoral degree in Mechanical (Biomedical) Engineering from Columbia University, USA. He has published approximately 300 papers in peer-reviewed journals and holds 30 patents on numerous FDA-approved medical devices. Since 1989, Dr Athanasiou has focused on cartilage tissue engineering research.
Footnotes
Competing interests
A.H.G. declares that he consults for SANOFI S.A. The other authors declare no competing interests.
Review criteria
We searched for original articles focusing on cartilage repair and regeneration in MEDLINE, PubMed, Embase and the Cochrane Controlled Trials Register, published 2000–2014. The search terms alone and in combination were “cartilage repair”, “cartilage regeneration”, “cartilage randomized controlled trials”, “cartilage cohorts”, “cartilage systematic reviews”, “cartilage scaffolds”, “engineered cartilage”, “chondrocyte tissue engineering”, “stem cells and chondrocyte” and “chondrocyte and growth factors”. Preliminary searches were undertaken in March 2014 and were updated in May 2014. All publications identified were English-language, full-text papers. We also searched the reference lists of identified articles for additional publications.
Author contributions
All authors contributed equally to researching data for the article, substantially contributing to discussion of content, writing and review/editing of the manuscript before submission.
References
Full text links
Read article at publisher's site: https://doi.org/10.1038/nrrheum.2014.157
Read article for free, from open access legal sources, via Unpaywall:
https://escholarship.org/content/qt76m4g3q5/qt76m4g3q5.pdf?t=oe66jy
Citations & impact
Impact metrics
Article citations
An Adhesive Hydrogel Technology for Enhanced Cartilage Repair: A Preliminary Proof of Concept.
Gels, 10(10):657, 14 Oct 2024
Cited by: 0 articles | PMID: 39451310 | PMCID: PMC11507104
Polysaccharide-based hydrogels for cartilage regeneration.
Front Cell Dev Biol, 12:1444358, 11 Oct 2024
Cited by: 0 articles | PMID: 39463764 | PMCID: PMC11503028
Review Free full text in Europe PMC
Three-Dimensional Printed Silk Fibroin/Hyaluronic Acid Scaffold with Functionalized Modification Results in Excellent Mechanical Strength and Efficient Endogenous Cell Recruitment for Articular Cartilage Regeneration.
Int J Mol Sci, 25(19):10523, 29 Sep 2024
Cited by: 0 articles | PMID: 39408852 | PMCID: PMC11477338
Electrical stimulation for cartilage tissue engineering - A critical review from an engineer's perspective.
Heliyon, 10(19):e38112, 23 Sep 2024
Cited by: 0 articles | PMID: 39416819 | PMCID: PMC11481755
Review Free full text in Europe PMC
Cartilage Repair: Promise of Adhesive Orthopedic Hydrogels.
Int J Mol Sci, 25(18):9984, 16 Sep 2024
Cited by: 1 article | PMID: 39337473 | PMCID: PMC11432485
Review Free full text in Europe PMC
Go to all (465) article citations
Data
Data behind the article
This data has been text mined from the article, or deposited into data resources.
BioStudies: supplemental material and supporting data
Similar Articles
To arrive at the top five similar articles we use a word-weighted algorithm to compare words from the Title and Abstract of each citation.
Autologous-cell-derived, tissue-engineered cartilage for repairing articular cartilage lesions in the knee: study protocol for a randomized controlled trial.
Trials, 18(1):519, 06 Nov 2017
Cited by: 9 articles | PMID: 29110690 | PMCID: PMC5674846
Treatment of osteochondral defects in the rabbit's knee joint by implantation of allogeneic mesenchymal stem cells in fibrin clots.
J Vis Exp, (75):e4423, 21 May 2013
Cited by: 8 articles | PMID: 23728213 | PMCID: PMC3711376
[Treatment strategy of osteochondral defects of knee joint].
Zhongguo Xiu Fu Chong Jian Wai Ke Za Zhi, 28(1):113-118, 01 Jan 2014
Cited by: 0 articles | PMID: 24693792
Review
Clinical relevance of scaffolds for cartilage engineering.
Orthop Clin North Am, 43(2):245-54, vi, 07 Mar 2012
Cited by: 6 articles | PMID: 22480473
Review
Funding
Funders who supported this work.
NIAMS NIH HHS (1)
Grant ID: R01 AR061496
NIDCR NIH HHS (2)
Grant ID: R01 DE015038
Grant ID: R01 DE019666