Abstract
Free full text

Evolution of central pattern generators and rhythmic behaviours
Abstract
Comparisons of rhythmic movements and the central pattern generators (CPGs) that control them uncover principles about the evolution of behaviour and neural circuits. Over the course of evolutionary history, gradual evolution of behaviours and their neural circuitry within any lineage of animals has been a predominant occurrence. Small changes in gene regulation can lead to divergence of circuit organization and corresponding changes in behaviour. However, some behavioural divergence has resulted from large-scale rewiring of the neural network. Divergence of CPG circuits has also occurred without a corresponding change in behaviour. When analogous rhythmic behaviours have evolved independently, it has generally been with different neural mechanisms. Repeated evolution of particular rhythmic behaviours has occurred within some lineages due to parallel evolution or latent CPGs. Particular motor pattern generating mechanisms have also evolved independently in separate lineages. The evolution of CPGs and rhythmic behaviours shows that although most behaviours and neural circuits are highly conserved, the nature of the behaviour does not dictate the neural mechanism and that the presence of homologous neural components does not determine the behaviour. This suggests that although behaviour is generated by neural circuits, natural selection can act separately on these two levels of biological organization.
1. Evolution of rhythmic behaviours
‘… from so simple a beginning endless forms most beautiful and most wonderful have been, and are being, evolved.’
—Charles Darwin [1, p. 490]
When contemplating life on this planet, one is struck by the vast variety of animal behaviours. Considering just locomotor behaviours alone, there is swimming, crawling, flying, gliding, brachiating and running. Reflecting on only terrestrial locomotion, a small sample includes bipedal walking (humans), knuckle walking (gorillas), ‘tripedal’ gait (kangaroos), quadrupedal gaits (horses), hexapodal gaits (insects), octopedal walking (spiders), hopping (frogs), sinusoidal movements (snakes), contraction and elongation (worms), mucosal gliding (snails) and metacronal waves (millipedes). Any comprehensive description of the evolution of nervous systems needs to take into account how nervous systems generate this great diversity of behaviours.
(a) Evolution of central pattern generators
Central pattern generators (CPGs) are neural circuits that produce the patterns of neural activity that underlie rhythmic motor behaviours such as walking, swimming and feeding [2,3]. As the name implies, these patterns are generated centrally, without the need for sensory feedback or other patterned input. Thus, many experimental CPG preparations can continue to produce rhythmic neural activity even when isolated from the animal, facilitating access to the neurons. The rhythmic motor behaviour is a direct readout of the pattern of neuronal activity, making it straightforward to make conclusions about the neural basis of the behaviour. The cyclic nature of motor patterns provides a robust means of delineating which neurons produce the rhythmicity and pattern because cellular members of the CPG are rhythmically active and synaptically connected to other members of the CPG. Furthermore, artificially exciting or inhibiting CPG members perturbs the motor pattern. In many invertebrates, individual neurons can be identified from animal to animal within a species, allowing the neural circuits to be determined with cellular precision. Moreover, homologous neurons can be identified across species, permitting comparative analyses of CPG circuits and the rhythmic behaviours that they produce [4,5]. Thus CPGs provide extraordinary opportunities to study the evolution of behaviour and neural circuits.
(b) Behaviours and neural mechanisms represent different levels of organization
Generally, rhythmic behaviours and the CPGs that produce them evolved slowly and in unison. However, there are examples where behaviour and neural mechanisms had different evolutionary histories. Figure 1 illustrates a hypothetical phylogenetic tree containing three clades (α, β, γ) in which animals exhibit three basic behaviours (A, B, C), which are produced by variants of three neural mechanisms (a, b, c). In this tree, the common ancestor for clade α used neural mechanism a to produce behaviour A. Similarly, the common ancestors for clades β and γ used neural mechanisms b and c to produce behaviours B and C, respectively. For the most part, the neural mechanisms and the behaviours are conserved within each clade. The bars mark transitions in the neural mechanisms. For example, a gradual change in neural mechanism (a → a′ → a″) was accompanied by corresponding changes in behaviour (A → A′ → A″). Even in cases of convergent evolution, the same neural mechanisms might produce analogous behaviours as seen with the independent evolution of neural mechanism a and behaviour A in clade γ (figure 1).
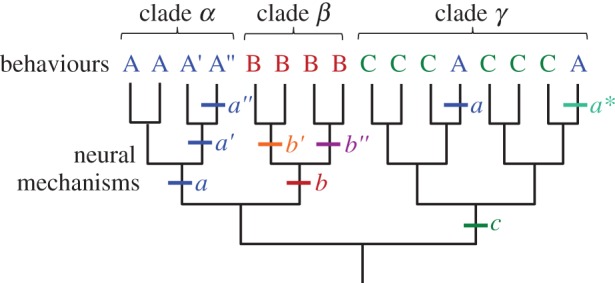
Independence of behaviours and neural mechanisms. This hypothetical tree shows a how behaviours A, B and C and their variations might have evolved. There are three clades (α, β, γ) which have ancestral neural mechanisms a, b and c. See §1b for further explanation. (Online version in colour.)
Striedter & Northcutt [6] and Sommer [7] proposed that behaviour and neural mechanisms represent separate levels of biological hierarchy. Thus, for example, convergent evolution of behaviour A in clade γ could be through the novel neural mechanism a* rather than through re-evolution of neural mechanism a (figure 1). Conversely, species might have homologous behaviours, such as behaviour B in clade β, yet have diverged in the neural mechanisms that produce them (b′ and b″). It is important to recognize that there might not be a clean hierarchical distinction between behaviours and the neural mechanisms that produce them; the organization or development of the nervous system itself constrains potential behaviours [8–10]. In §§2–5, I will discuss real world examples of some of these scenarios as well as others.
2. Divergence of central pattern generators and rhythmic behaviours
Nervous systems tend to be highly conserved. The basic developmental mechanisms that form the spinal cord are shared by all vertebrates [11–14]. Similarly, there is a common design for the segmentally repeated CPGs underlying undulatory movements in lampreys [12], Xenopus tadpoles [15], and possibly zebrafish larvae [16] (figure 2a,b). This basic organization, which was first proposed by Brown [17], is the half-centre oscillator, in which two mutually inhibitory ‘halves' alternate in activity. Addition of limbs required an elaboration of oscillator to include flexors and extensors in tetrapods (figure 2c). Similarly, control of fins in fish required more complex patterning than simple left–right alternation.
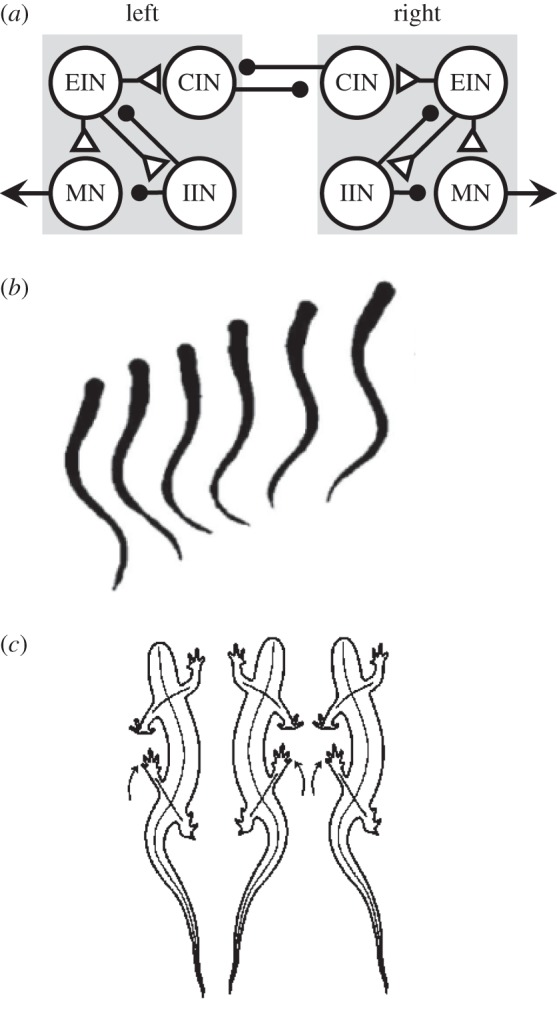
Conservative evolution of axial locomotion. (a) The segmental CPG for swimming in lampreys, tadpoles and zebrafish larvae is organized as a half-centre oscillator. The left and right sides are mutually inhibitory through commissural interneurons (CIN). Excitatory and inhibitory interneurons (EIN and IIN) participate in motor pattern generation. EIN synapses on motor neurons (MN) that cause muscle contraction. Each neuron in the diagram represents large pools of heterogeneous neurons. Triangles are excitatory synapses and circles are inhibitory. CPG based on ref. [16]. (b) The lamprey swims with a sinusoidal movement. The left and right sides of the body alternately flex. (c) Salamanders walk using an alternating axial muscle gait with left and right sides in alternation.
(a) Evolutionary developmental mechanisms for rewiring central pattern generators
We are just at the threshold of understanding the evo-devo [18,19] mechanisms underlying neural circuit evolution. Frog metamorphosis offers a glimpse into how the evolutionary process of transforming a CPG based on an axial half-centre to a limb-based locomotor system might have occurred [20–22]. Tadpoles swim by progressive undulations of their tail (figure 3a), with the left and right sides in alternation at any time. In contrast, adult frogs use their hindlimbs for propulsion with the left and right hind limbs working in synchrony and alternation occurring between limb flexors and extensors (figure 3d). During metamorphosis, there is a gradual transition of the legs from left–right alternation (figure 3b) to left–right synchrony (figure 3c,d). At one point, there is coexistence of the tail and leg motor patterns, indicating that these two CPGs coexist in the spinal cord (figure 3c). The leg circuitry arises from the axial CPG circuitry. As the leg CPG becomes separate, the role of nitric oxide (NO) shifts. Initially, nitric oxide synthase (NOS) is not expressed in the regions of the spinal cord where the presumptive limb networks are developing [23]. It is thought that NO inhibits the development of these networks and that its absence permits the proliferation of the neurons involved in limb movements. By late pre-metamorphosis (figure 3c), NOS staining is found throughout the spinal cord, possibly to end proliferation. Thus, even within one animal, there is a divergence of CPG circuits underlying radically different behaviours. It is not hard to extrapolate from this example how limbed movement might have evolved from an axial-based CPG.
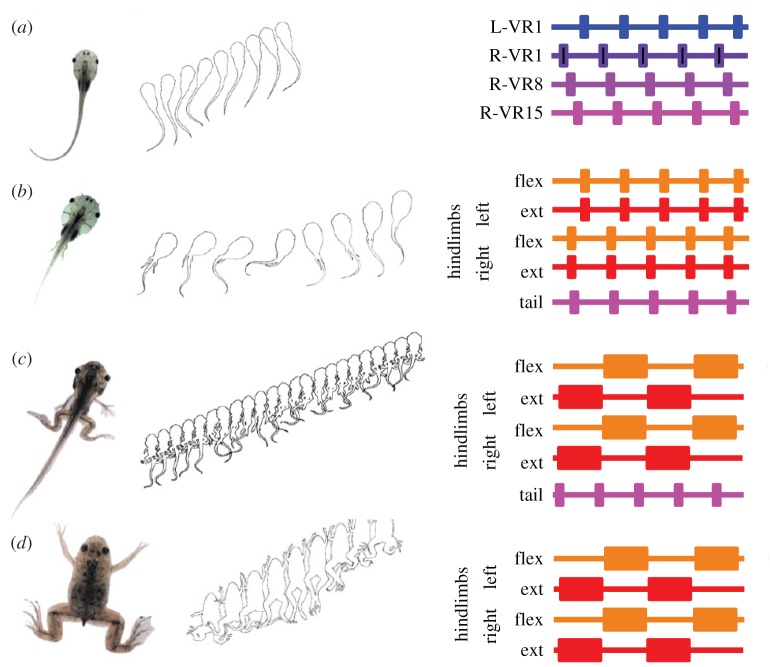
Metamorphosis of Xenopus showing changes in motor patterns. (a) Tadpoles exhibit axial locomotion with left and right sides of the tail alternating. The plot on the right shows an idealized recording of ventral root activity, with alternation between left and right at root one (VR1) and progressive activity down spinal segments on the right side (VR1, 8, 15). (b) In early premetamorphosis, limb buds have emerged, but activity is still strictly axial with left right alternation. Flexors and extensors on hindlimbs are coactive with tail. (c) In late premetamorphosis, the legs are now independent of the tail and two motor patterns exist at the same time, a rapid tail movement and a slower bilaterally symmetric kicking movement. Now, flexors and extensors alternate, but left and right legs are coactive. (d) In the adult frog, the tail has been absorbed and the legs continue to exhibit a bilaterally symmetric kicking movement. Adapted from [20]. (Online version in colour.)
There are some interesting studies with regard to evolutionary development of CPGs, which suggest that small genetic changes can rewire a CPG and cause significant changes to the motor pattern. For example, a single gene mutation in Icelandic horses affects their gait. The horses normally prefer a trotting gait, in which diagonally opposite legs move together (figure 4a). A premature stop codon in the DMRT3 gene is permissive for the horse to have a ‘pacing’ gait, in which the legs on the same side of the body move together (figure 4b) [24]. When expressed in mice, this allele disrupts motor pattern coordination (figure 4c,d). DMRT3 is normally expressed in a subset of spinal interneurons that project ipsilaterally and contralaterally, but its exact role is not known. It might serve as a signalling molecule for axon growth. For example, the ephrin receptor A4 (EphA4) and ephrin ligand B3 (EphrinB3) are axon guidance molecules that regulate whether axons cross the midline of the spinal cord during development (figure 4e). When either of these molecules is knocked out in the spinal cord, excitatory interneurons are allowed to cross the midline. Mice with EphA4 knocked out have a hopping gait instead of an alternating gait (figure 4f,g) [25,26]. Thus, changes in the expression of single genes can rewire CPGs for locomotion and thereby alter gait in a heritable manner. Interestingly, regulation of genes for the SLIT-ROBO axon guidance molecules are associated with the evolution of vocal learning in birds and humans [27], suggesting that axon guidance signalling pathways, such as these, play an important role in the evolution of neural circuits in general.
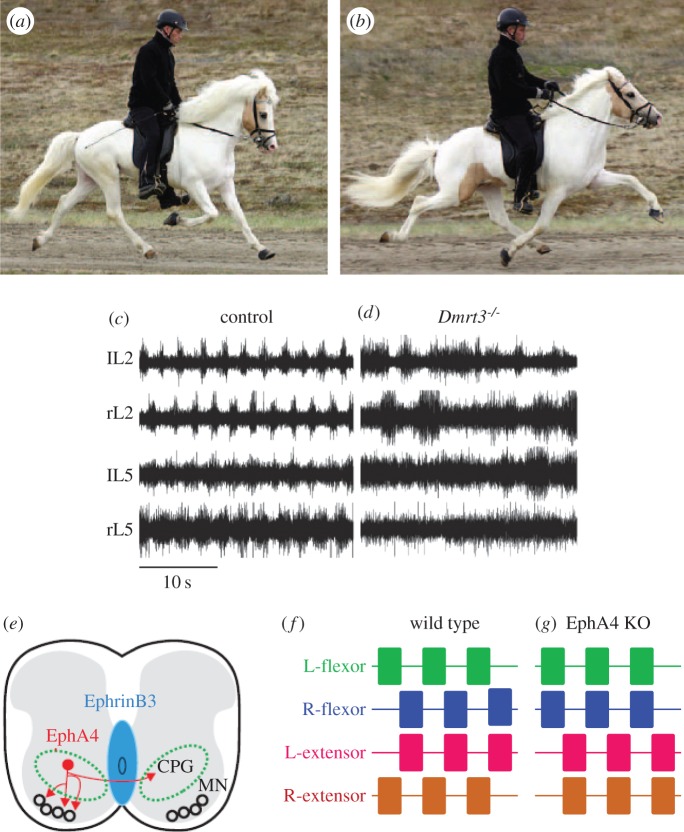
Single gene mutations cause changes in mammalian gait. (a) When trotting, the legs on the same side move in opposite directions. (b) When pacing, ipsilateral legs move in the same direction. Icelandic horses homozygous for a nonsense mutation in the DMRT3 gene naturally pace. (c) In typical fictive locomotion from a neonatal mouse spinal cord, regular rhythmic activity occurs in the left (l) and right (r) lumber (L) roots from spinal ventral roots 2 and 5. (d) In mice lacking Dmrt3 expression, the fictive motor pattern is irregular. (e) Neurons expressing the ephrin receptor A4 (EphA4, black) normally are repelled by the ephrin ligand B3 (EphrinB3, dark grey). In EphA4 knock-out mice, these neurons can cross the midline. (f) Schematic of the fictive motor pattern from a wild-type mouse shows normal left–right alternation. (g) In EphA4 knockout mice, left and right flexors burst synchronously and out of phase with extensors, producing a hopping gait. (a–d) Adapted with permission from [24]; (e) based upon Kiehn [25]. (Online version in colour.)
(b) Restructuring central pattern generators of identified neurons
Invertebrates with homologous identified neurons permit a view of the extent of neural circuit change that can occur during divergence of behaviour. In nudibranch molluscs, homologous neurons differ in function in species exhibiting different behaviours. Most nudibranchs do not swim, but those species that do, generally use one of two modes, alternating dorsal–ventral (DV) whole body flexions or rhythmic left–right (LR) flexions [4]. Neurons that are part of the DV swim CPG have homologues in species that produce LR swimming [28–30], but these homologous neurons are not part of the LR swim CPG. Instead, the two types of CPG are composed of non-overlapping sets of neurons [4]. Nonetheless, homologues of DV swim CPG neurons can have a neuromodulatory effect on the LR swim CPG [31]. Thus, the functions of homologous neurons differ across species; they are intrinsic to the DV swim CPG, but extrinsic modulators of the LR swim CPG.
In nematodes, entire neural networks have been rewired to produce divergent behaviours from the same set of neurons. For example, two species of nematode, Caenorhabditis elegans, which is the common laboratory species, and Pristionchus pacificus, a predatory nematode, each have individually identifiable neurons. The pharyngeal system used for feeding in both species is composed of homologous neurons and muscles. However, these species differ in feeding behaviour: C. elegans feeds on bacteria with pharyngeal pumping [32], whereas P. pacificus has jaws that it uses to feed upon other nematodes, including C. elegans (figure 5a). Neurons that produce pharyngeal pumping in C. elegans have different connectivity in P. pacificus, allowing them to control the jaw (figure 5b) [33]. In other nematode species that also exhibit pharyngeal pumping, there has been respecification of the roles of motor neurons [34]. Remodelling of synaptic connections has also been seen when comparing nociceptive circuits in different nematode species [35]. Thus, even in species with strictly defined cell numbers, homologous neurons have been rewired to produce divergent behaviour.
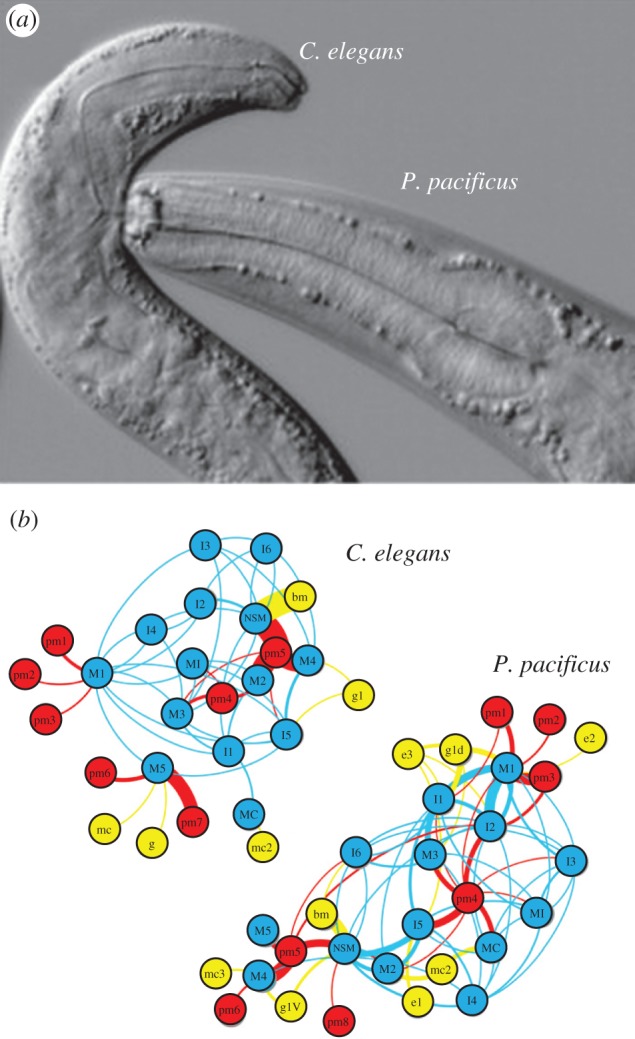
Different feeding neural circuitry in two nematodes. (a) The nematodes C. elegans and P. pacificus have different feeding behaviours. In C. elegans, a terminal bulb structure called the grinder mechanically breaks up bacteria. The grinder is missing in P. pacificus, which instead has a predatory dorsal tooth that breaks open prey items. The picture shows P. pacificus feeding on C. elegans. (b) Schematic of the C. elegans and P. pacificus feeding neural networks showing massive rewiring of neurons and their connections to muscles and other outputs such as epithelial cells and glands. Lines curve clockwise from presynaptic to postsynaptic targets. Line width indicates weight according to the number of synaptic connections observed in serial electron microscopic images. Based on Bumbarger et al. [33]. (Online version in colour.)
(c) Neuromodulatory mechanisms for functionally rewiring central pattern generators
Neurons do not need to be rewired physically to change the motor pattern; neuromodulatory actions can functionally rewire a CPG by strengthening or weakening synapses and by altering membrane conductances [36–38]. Therefore, changes in neuromodulation, either through differences in receptor expression or neurotransmitter content, could cause species differences in the output of neural circuits [39].
Homologous neurons that provide neuromodulatory input to CPG circuits in the stomatogastric nervous system of decapod crustaceans differ in the presence of neuropeptides resulting in species-specific changes to the motor pattern [40]. The same is true for serotonin: in the crab, Cancer borealis and the lobster, Homarus americanus, serotonin is released from a set of mechanosensory neurons called GPR cells [41–43]. However, in the spiny lobster, Panulirus interruptus, the GPR cells do not contain serotonin [44]. Neurons in the stomatogastric ganglion of Panulirus are one thousand times more sensitive to serotonin than their homologues in Cancer and Homarus, suggesting that serotonin arrives as a neurohormone in Panulirus instead of as a neurotransmitter from a sensory neuron. The effects of serotonin also differed in each of the species [44]. Thus, the mode of release of a neuromodulatory substance can cause different activity to be produced from homologous networks.
3. Divergence of central pattern generators underlying homologous behaviours
Behaviours, like any other trait, are presumed to be homologous if they are present in every member of a clade. Even if two behaviours are homologous, the neural circuitry can diverge (figure 1b′ and b″). This occurred for the CPGs underlying swimming behaviours of the nudibranchs Dendronotus iris and Melibe leonina. Both species exhibit a similar swimming behaviour consisting of alternating LR whole body flexions (figure 6a,d). The two species are members of the monophyletic clade, Cladobranchia [47,48]. Recent phylogenic analysis suggests that Dendronotus and Melibe belong to a monophyletic subclade that, to the best of our knowledge, contains only species that swim with LR body flexions [49]. Therefore, these behaviours are likely to be homologous.
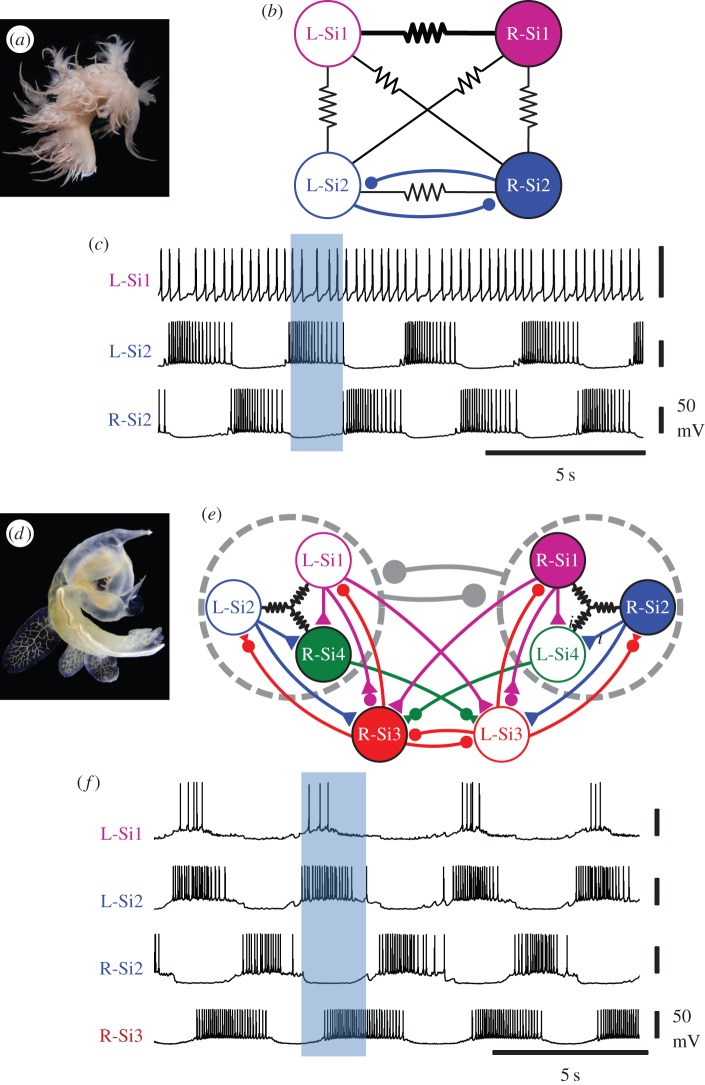
Divergent CPGs underlying homologous behaviours. (a) Dendronotus iris swims with left–right whole body flexions. (b) The CPG underlying this behaviour has a simple half-centre organization with the left and right Si2 inhibiting each other. Si1 does not make or receive any contralateral inhibition. (c) Simultaneous intracellular recordings show that Si1 fires irregularly, whereas the two contralateral Si2 burst in alternation during the swim motor pattern. (d) Melibe leonina swims in the same manner as Dendronotus. (e) The swim CPG in Melibe is more complicated than that of Dendronotus. Si1, Si2 and the contralateral Si4 are electrically coupled and each inhibits its own contralateral counterpart (represented by the grey dashed circle). The left and right Si3 inhibit each other and have complex synaptic interactions with the other CPG neurons. (f) Simultaneous intracellular microelectrode recordings show that the ipsilateral Si1 and Si2 fire in bursts of action potentials in alternation with the contralateral Si2. The contralateral Si3 follows Si2. Filled circles represent inhibitory synapses, triangles represent excitatory synapses and mixtures of the two represent multicomponent synapses. Resistor symbols represent electrical connections. Based on Sakurai et al. [45] and Sakurai & Katz [46]. (Online version in colour.)
The Dendronotus swim CPG is a half-centre oscillator consisting of two neurons (left and right Si2) that are mutually inhibitory and fire action potentials in strict alternation (figure 6b,c) [45]. Another bilaterally represented pair of neurons (Si1) does not have mutual inhibition and does not exhibit rhythmic bursting (figure 6b,c). In contrast, the Melibe swim CPG is much more complex [46]. There is a kernel of electrically coupled neurons, which forms a half-centre: Si1, Si2 and the contralateral Si4 are electrically coupled to each other and each inhibits its contralateral counterpart. There is another pair of mutually inhibitory neurons (Si3) that interacts with the Si1, Si2, Si4 kernel (figure 6e). Unlike in Dendronotus, Si1 fires bursts of action potentials in phase with the ipsilateral Si2 (figure 6f). Although the behaviour is a simple alternation, there are multiple phases of activity within the CPG; Si3 fires bursts after the contralateral Si2. Thus, even though Dendronotus and Melibe have homologous swimming behaviours, the CPGs have diverged, while the outward behaviours have remained similar.
4. Independent evolution of rhythmic behaviours
Although the list of all locomotor behaviours is long, it is not ‘endless' as Darwin wrote because they are constrained by development and physics [50]. Developmental patterning in vertebrates means that there has never existed, nor is there likely to ever exist, a horse that resembles Pegasus, with wings emerging from the back. Not only is the range of possible behaviours finite, but many of the existing modes of locomotion have evolved independently several times. For example, kangaroos, kangaroo rats and jerboas independently evolved bipedal hopping. Thus, rather than there being endless forms, in fact there are a limited number of forms that are reused. The notion that evolution can repeat itself has suggested to some that it might in fact be predicable [51–54] and that there might be genetic causes for convergent evolution of some features [55]. Similarly, if rhythmic behaviours repeatedly evolved, perhaps there are configurations of neural circuits that re-evolved with them.
It is important to distinguish convergent evolution from parallel evolution [56–58]. In the former, non-homologous traits come to have similar properties, whereas in the latter, homologous traits come to have similar properties independently. Both are examples of ‘independent evolution’ or homoplasy. However, whereas convergent evolution provides information about the function of the trait, parallel evolution provides additional information about the processes necessary to develop or evolve the character. In other words, the endpoint similarity in parallel evolution might reveal more about constraints on phenotypic space than about the ultimate function of the trait [59]. These distinctions are important for understanding the evolution of neural mechanisms and behaviour. If similar behaviours evolved convergently, then information about the neural mechanisms could provide insights into general properties of neural circuits. In contrast, if similar behaviours evolved through parallel evolution, then the neural mechanisms could provide insights into constraints or opportunities for evolution, in other words, the ‘evolvability’ of these behaviours [8,10].
(a) Flapping flight provides examples of divergence, homology, convergence and parallel evolution
The term ‘homology’ has been defined in many ways [60]. Here, homology refers to any character that was present in the last common ancestor of two species as determined by a cladistic analysis. For example, flight is a behaviour that is homologous in all birds because the common ancestor of birds flew. The evolution of flight involved changes not only in the morphology and musculature of the forelimbs, but in the pattern of neural activity that drives them [61,62] and in the sensory feedback that they receive [63]. Comparisons of muscle histology in birds suggest further that changes in muscle chemistry also played a role in the evolution of flight movements [61].
Flight behaviour has diverged significantly across birds from the graceful flapping of owls to the rapid movements of hummingbirds [64]. Flight has been lost in many bird lineages, such as ratites [65,66]. It is possible that the circuitry underlying flight still exists in non-flying birds, but the external apparatus has been rendered incapable of flight. Certainly, flightless birds are still capable of flapping their wings. Clearly, there must have been divergence in the neural mechanisms underlying diverse forms of flight.
Flapping flight evolved independently in birds, bats, extinct pterosaurs and insects. There are mechanical differences in how these animals fly [67]. Furthermore, the sensory input to the flight circuits differs. Bats have specialized hairs on the skin of their wings that help sense airflow [68], birds use feather movements to sense airflow, and insects have various other types of receptors [69]. Birds, bats and pterosaurs rhythmically flap their forelimbs, which are homologous in all tetrapods. In contrast, insect wings are dorsal appendages that are quite distinct from limbs and not homologous to tetrapod legs. This mixture of homology and novel components for the tetrapods demonstrates that for complex traits such as flapping flight, homoplasy is generally not due to convergence or parallelism alone. In contrast, powered insect flight appears to be completely convergent with the others.
(b) Independent evolution of central pattern generators and rhythmic behaviours
Although there are many examples of convergent evolution of rhythmic behaviours, few of these seem to involve convergent evolution of neural mechanisms as illustrated by behaviour A produced by neural mechanism a in clade γ (figure 1). There are, however, examples of independent evolution of behaviour though parallel evolution of neural mechanisms. That is to say, homologous neural substrates independently evolved the same mechanism to produce similar behaviours. For example, DV swimming evolved independently in two sea slugs Tritonia diomedea (figure 7a) and Pleurobranchaea californica (figure 7c). Yet their CPGs contain homologous neurons, including a set of three serotonergic neurons called the DSIs, which participate in the rhythmic motor pattern. In both species, release of serotonin from the DSIs increases the strength of synapses made by another CPG member, C2 (figure 7b,d). Furthermore, serotonin is necessary for the activation of the swim motor pattern. This neuromodulatory action is missing in another nudibranch, Hermissenda crassicornis, which is not a DV swimmer (figure 7e,f) [70]. This suggests that evolution of the DV swim CPG may have involved parallel changes in the serotonergic neuromodulation of homologous neurons.
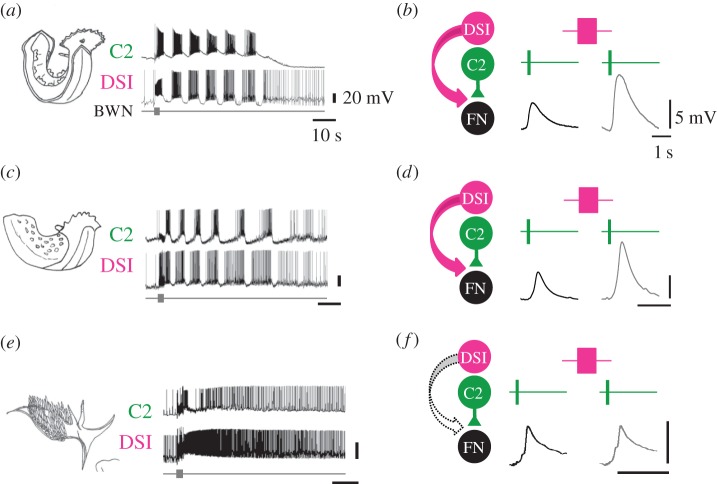
Parallel evolution of neuromodulatory actions may occur under independent evolution of behaviour. Tritonia diomedea (a,b) and P. californica (c,d) swim with alternating dorsal and ventral body flexions. C2 and DSI, members of the swim CPG, fire rhythmic bursts of action potentials during swim motor patterns evoked by stimulation of a body wall nerve (BWN). DSI stimulation increases the size of EPSPs evoked by C2 in a follower neuron (FN) (bottom). Hermissenda crassicornis (e,f) does not swim with dorsal ventral flexions and BWN stimulation does not cause bursting in the C2 and DSI homologues. Although the DSIs are serotonergic, DSI stimulation also does not increase the size of C2-evoked EPSPs. Based on Lillvis & Katz [70]. (Online version in colour.)
(c) Repeated evolution of rhythmic behaviours through latent central pattern generators
Some traits have been lost and then regained in the same lineage. In some cases, the reversion is permitted by the retention of gene networks for the lost feature that can be reactivated. For example, there is strong evidence for repeated limb and digit loss and the repeated reappearance of this complex morphological trait in a clade of lizards [71]. It is of interest to understand whether, like reactivation of gene networks, the re-evolution of rhythmic behaviour involves the reactivation of latent neural circuitry.
In general, the nervous system seems to be more highly conserved than the neuromuscular system. Arbas [72,73] compared the nervous system of locusts, which are excellent flyers, to that of flightless grasshoppers, which lack hindwings and have only vestigial forewings. Homologues of motor neurons that innervate flight muscles in locusts persist into adulthood in the grasshoppers, even maintaining presynaptic terminals despite the absence of muscles to innervate [74]. Wing stretch receptor cells are also retained [72]. Furthermore, interneurons that play an important role in locust flight are also found, with very little change in morphology in the flightless grasshoppers [73]. This suggests that the components of the nervous system are highly conserved, whereas the periphery can exhibit much more phylogenetic variability.
Insect flight has been lost several times [65,75]. In a study on stoneflies, it was found that the loss of flight preceded loss of the wings [76], suggesting that the evolutionary pressure is on the behaviour, not the structure. The ability to generate the flight motor pattern and the presence of wings are therefore disconnected. For example, larval locusts lack wings, yet the flight motor pattern can be recorded from the nervous system when the monoamine, octopamine, is applied [77]. This suggests that the flight CPG is latent in the larval nervous system and requires octopamine to be activated. Similarly, a recent molecular phylogeny of stick insects indicates that they have lost and regained flight several times [78]. One potential mechanism for such apparent repeated loss and gain is that the developmental programme was never actually lost. It is possible that the flightless stick insects have the potential for flight, but perhaps have had their development arrested in a neotenous state that lacks the correct neuromodulatory activation.
(d) Independent evolution of rhythmic behaviours produced by distinct neural mechanisms
Leeches and C. elegans have sinusoidal locomotor behaviours that resemble lamprey swimming behaviour, but are produced by CPG circuits that are fundamentally different from each other and from that of lamprey. In lampreys, the left and right sides contract in antiphase, producing an LR alternation of each body segment (figure 2b). However, in leeches and nematodes, the dorsal and ventral sides are out of phase with each other, causing them to undulate up and down rather than side-to-side (figure 8a,b).
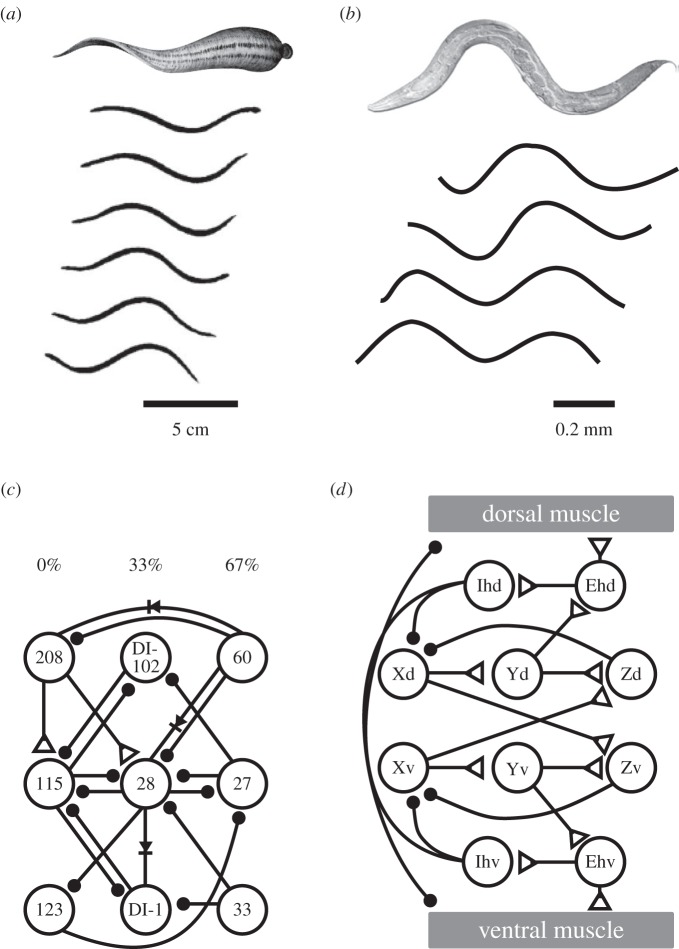
Leeches and nematodes have independently evolved sinusoidal locomotor behaviours using different CPG circuit motifs. (a) Top image is of a leech swimming, the bottom is a series of traces from a movie, showing the sinusoidal dorsal–ventral movements (adapted from [79]). (b) The nematode C. elegans also moves using sinusoidal dorsal–ventral movements (adapted from [80]). (c) The leech segmental CPG has neurons that fire in three phases of activity, represented at per cent of the period (0%, 33%, 67%). Although most connections are inhibitory, there is no half-centre motif (based on Mullins et al. [79]). (d) A hypothetical computational model of the C. elegans locomotor CPG suggests that it could be located in the head. It contains mostly excitatory connections with inhibitory feedback. There are also inhibitory inputs to muscles that help set up the alternating movements. Note that the neurons in this model, which is based on Karbowski et al. [81] are hypothetical and do not follow the C. elegans nomenclature.
Although these behaviours resemble each other, the composition and structure of the underlying CPGs differ substantially. Whereas the lamprey segmental CPG has a half-centre organization with the left and right sides mutually inhibitory and firing 180° out of phase [82] (figure 2a), the neurons in each segment of the leech swim CPG fire in three phases, each 33% out of phase with the others [79,83] (figure 8c).
The CPG for sinusoidal movement in C. elegans has not been definitively determined [84]. However, one model suggests that it is not segmentally organized [81]. Instead, the CPG is located in the head ganglion (figure 8d). The CPG outputs to muscles in the head and to two interneurons that run the length of the animal (not shown). These interneurons, along with a third interneuron from the tail, coordinate the activity of excitatory and inhibitory motor neurons along the length of the animal. The CPG itself does not resemble the segmental CPGs of either leech or lamprey. The alternations of dorsal and ventral firing do not arise through a set of mutually inhibitory neurons, but rather though crossed excitatory neurons that synapse onto inhibitory neurons. Unlike the leech swim CPG, where most of the connections are inhibitory, most of the synapses in the C. elegans locomotor CPG are excitatory [81]. Thus, lampreys, leeches and nematodes converged onto sinusoidal movement, but used three different network architectures and non-homologous neurons. This is equivalent to the evolution of behaviour A in clade γ using neural mechanism a* (figure 1).
5. Convergent central pattern generator properties underlying distinct behaviours
There are many commonalities to CPGs regardless of the behaviour or species or even phylum. These include membrane and synaptic properties and circuit connectivity. These common properties, which have been called the ‘building blocks' of CPGs [85–88], often represent examples of convergent evolution. Finding them in different phyla provides evidence of their importance for generating rhythmic activity. Most CPG circuits rely on inhibition rather than excitation to maintain rhythmicity. As discussed, mutual inhibition in the form of a half-centre oscillator is a common, but not universal, network architecture of CPGs. It is found in molluscan swimming [46], leech heartbeat [84], lamprey swimming [89] and tadpole swimming [15], among others [90]. The flapping movements of the pteropod mollusc Clione limacina are also produced by a CPG organized around a half-centre oscillator [91]. The membrane properties of neurons in CPGs also show convergence. For example, CPG neurons often exhibit post-inhibitory rebound, intrinsic bursting and bistability [92]. One indication that these are convergent properties rather than conserved in all neurons is that they are emergent properties that arise from different sets of ion conductances [93]. Thus, these common features of CPGs have been established as principles of operation that occur across phylogenetic groups and across different types of behaviours [94]. Comparisons of the mechanisms used by different half-centre oscillators have provided insights into the production of rhythmic behaviour [95].
6. Summary
Work on CPGs has shown that, to a large extent, behaviour and neural mechanism act as separate levels of the biological hierarchy with respect to evolution (figure 1). The nervous system is generally conservative and gradual evolution of CPGs goes hand in glove with evolution of rhythmic behaviours. The mechanisms for rewiring a CPG network might be quite simple, such as changes in axon guidance molecules. Networks also can be functionally rewired through changes in neuromodulation.
There are also striking examples where evolution of neural circuitry and behaviour are not aligned. Analogous behaviours can be produced by different neural mechanisms. In fact, even homologous behaviours can diverge in their neural mechanisms. Homologous neurons can diverge in their functionality in networks, with entire networks being rewired. Within a clade of closely related species, a rhythmic behaviour can be lost and regained several times, perhaps because of retained latent circuitry.
CPG circuitry itself might be under evolutionary pressure that is invisible at the behavioural level. Thus, there can be divergence of neural circuitry underlying similar behaviour. Although the details of the behaviours and the circuits differ, CPG circuits continually converge on particular properties, such as mutual inhibition and post-inhibitory rebound, which have been recognized as important from a reverse engineering standpoint. The convergent evolution of these emergent properties across the phylogeny is a strong indication of their importance in production of rhythmic behaviour.
In summary, the diversity of animal behaviour comes about through a variety of different mechanisms. It is clear that knowing the neurons and knowing the behaviour is not sufficient for understanding the neural basis of that behaviour, but it is an important start. This has significance for neuroscientists trying to extrapolate from one species to another and for evolutionary biologists interested in explaining the evolution of behaviours.
Acknowledgements
I thank Michael Dickinson for insightful discussions about insect flight and Jessica Goodheart for sharing her phylogenetic analysis. I am grateful for the feedback that I received on this manuscript from Sarah Pallas, Akira Sakurai, Charuni Gunaratne and Jonathan Boykin.
Funding
Research in support to P.S.K. from NSF-IOS-1120950 and the March of Dimes Foundation 6-FY14–441.
References
Articles from Philosophical Transactions of the Royal Society B: Biological Sciences are provided here courtesy of The Royal Society
Full text links
Read article at publisher's site: https://doi.org/10.1098/rstb.2015.0057
Read article for free, from open access legal sources, via Unpaywall:
https://royalsocietypublishing.org/doi/pdf/10.1098/rstb.2015.0057
Citations & impact
Impact metrics
Article citations
Ancestral neural circuits potentiate the origin of a female sexual behavior in Drosophila.
Nat Commun, 15(1):9210, 28 Oct 2024
Cited by: 1 article | PMID: 39468043 | PMCID: PMC11519493
The central role of the individual in the history of brains.
Neurosci Biobehav Rev, 163:105744, 31 May 2024
Cited by: 0 articles | PMID: 38825259
Review
Bioinspired Control Architecture for Adaptive and Resilient Navigation of Unmanned Underwater Vehicle in Monitoring Missions of Submerged Aquatic Vegetation Meadows.
Biomimetics (Basel), 9(6):329, 30 May 2024
Cited by: 0 articles | PMID: 38921208 | PMCID: PMC11201441
Pairing cellular and synaptic dynamics into building blocks of rhythmic neural circuits. A tutorial.
Front Netw Physiol, 4:1397151, 25 Jun 2024
Cited by: 0 articles | PMID: 38983123 | PMCID: PMC11231435
Changes in the cellular makeup of motor patterning circuits drive courtship song evolution in Drosophila.
Curr Biol, 34(11):2319-2329.e6, 29 Apr 2024
Cited by: 2 articles | PMID: 38688283
Go to all (70) article citations
Similar Articles
To arrive at the top five similar articles we use a word-weighted algorithm to compare words from the Title and Abstract of each citation.
Parallel evolution of serotonergic neuromodulation underlies independent evolution of rhythmic motor behavior.
J Neurosci, 33(6):2709-2717, 01 Feb 2013
Cited by: 26 articles | PMID: 23392697 | PMCID: PMC6619161
Neural mechanisms underlying the evolvability of behaviour.
Philos Trans R Soc Lond B Biol Sci, 366(1574):2086-2099, 01 Jul 2011
Cited by: 46 articles | PMID: 21690127 | PMCID: PMC3130364
Review Free full text in Europe PMC
Rhythmic behaviour and pattern-generating circuits in the locust: key concepts and recent updates.
J Insect Physiol, 56(8):834-843, 26 Mar 2010
Cited by: 16 articles | PMID: 20303972
Pedigrees of neurobehavioral circuits: tracing the evolution of novel behaviors by comparing motor patterns, muscles, and neurons in members of related taxa.
Brain Behav Evol, 38(4-5):226-239, 01 Jan 1991
Cited by: 15 articles | PMID: 1777805
Review
Funding
Funders who supported this work.
Directorate for Biological Sciences (1)
Grant ID: IOS-1120950