Abstract
Free full text

Local production of astrocytes in the cerebral cortex
Abstract
Astrocytes are the largest glial population in the mammalian brain. Astrocytes in the cerebral cortex are reportedly generated from four sources, namely radial glia, progenitors in the subventricular zone (SVZ progenitors), locally proliferating glia, and NG2 glia; it remains an open question, however, as to what extent these four cell types contribute to the substantial increase in astrocytes that occurs postnatally in the cerebral cortex. Here we summarize all possible sources of astrocytes and discuss their roles in this postnatal increase. In particular, we focus on astrocytes derived from local proliferation within the cortex.
Number of glia in the cerebral cortex
There is no significant difference in neuronal number between neonates and adults in the rodent cortex, but most glia in the rodent cortex are produced early in the postnatal period (Bandeira et al., 2009). At birth, non-neuronal cells (most of which are glia) in the rat brain comprise ~6% of brain cells; in adult rats, however, they account for nearly 50% of brain cells. Glial number in the cortex increases 6- to 8-fold from 4 to 6 million during postnatal (P) days 1–6 to 35 million at P21 and remains stable throughout adulthood (Bandeira et al., 2009). Glial number in the brain of other mammals, such as cats, undergoes a similar postnatal increase (Brizzee and Jacobs, 1959). From P60 in cat (juvenile period) to adulthood, glial number in the cerebral cortex increases and is accompanied by a huge change in the glia-to-neuron ratio; this ratio is ~0.83 at P60 and reaches 1.42 in adulthood, and then it increases slightly to 1.48 in late adulthood. In addition, the density of glia in the juvenile cat cortex increases by 60% upon reaching adulthood, and then it increases slightly thereafter (Brizzee and Jacobs, 1959). Astrocytes are the largest glial population in the mammalian brain, and most astrocytes are produced postnatally (Sauvageot and Stiles, 2002; Freeman, 2010). Researchers have identified multiple sources of astrocyte production in the cerebral cortex, including radial glia, SVZ progenitors, NG2 glia, and locally proliferating glia. However, the contribution of each of these sources differs among developmental stages. Below, we address recent evidence pertaining to this developmental change.
Radial glia–derived astrocytes and their contribution
Radial glia were originally discovered by Camillo Golgi in 1885 (Rakic, 2003). They have radially oriented long processes spanning the entire cortical wall in the human fetal cortex and spinal cord (Rakic, 1972; Choi and Lapham, 1978). Based on their morphology illustrated with Golgi impregnation, Cajal posited that radial glia likely transform into astrocytes in the cortex (Cajal, 1911). In the early embryonic stage of rhesus monkey, transitional radial glia detach from the ventricle surface with a long process terminating at blood vessels during the first half of gestation (Schmechel and Rakic, 1979; Levitt and Rakic, 1980). They become astrocytes with subsequent loss of radial orientation and extension of multiple stellate processes (Schmechel and Rakic, 1979). Similar observations were reported in the ferret brain (Voigt, 1989). Radial glia can be labeled via injection of tracers into the pial surface where radial glia endfeet are numerous. The tracers spread from the endfeet to the entire cell body of radial glia, so it is possible for researchers to follow the radial glia lineage (Voigt, 1989). In newborn ferrets, most tracer-labeled radial glia were found to become astrocytes in postnatal week 3 (Voigt, 1989). These results were confirmed by labeling translocating radial glia via DiI injection under the pial surface in the brain of human fetuses (deAzevedo et al., 2003) or by labeling foci of radial glia via adenovirus-Cre infection in the mouse cortex (Tsai et al., 2012). However, direct live imaging results to demonstrate that radial glia transform into astrocytes were obtained using cultured rat brain slices (Noctor et al., 2004). After 114 hours of time-lapse imaging with confocal microscopy, the clonal progeny of labeled radial glia were traced after they were infected with GFP-expressing viruses. Individual radial glia began to transform into astrocytes after they completed neurogenesis in late embryonic stages (Noctor et al., 2004). Radial glia translocated from the ventricular zone (VZ) to the intermediate zone and became immature astrocytes by retracting their long leading processes (Noctor et al., 2004). The transformed cells were characterized based on their astrocytic electrophysiological properties (Noctor et al., 2004). Given that astrocytes undergo a dramatic change in morphology during culture, it will be necessary to validate these results using in vivo imaging.
How do astrocytes derived from radial glia contribute to the entire mature astrocyte population in the cerebral cortex? After neurogenesis is completed in the mammalian brain, individual radial glia transform into individual astrocytes (Schmechel and Rakic, 1979; Voigt, 1989; Gressens et al., 1992; Noctor et al., 2004). However, because the astrocyte population of an adult brain is much larger than the radial glial population in a developing brain, the contribution of radial glia–derived astrocytes is believed to be small. Recent results suggest that a single radial glia might yield multiple astrocytes in the cerebral cortex. This is supported by genetic fate mapping with a Thy1.2-Cre mouse line (Magavi et al., 2012). Crossing this line with a reporter line resulted in a low rate of recombination. This enabled the analysis of a single column of clustered cells within the mouse cortex that were produced from an individual radial glia or neural progenitor. The cells in this single column included neurons and astrocytes at a relative ratio ranging from 1:6 to 1:8 (Magavi et al., 2012). In such columns, ~70% of neurons were projection neurons (Jones, 1993; Wonders and Anderson, 2005). According to the calculations of Magavi et al. (2012), most of the cortical astrocytes were originally derived from such developmental columns. Interestingly, most labeled cortical columns contained ~3 multiple-astrocyte clusters (a group of GFP-expressing astrocytes each within 25 μm of another GFP-expressing astrocyte). The authors mentioned that a single radial glia likely transforms into multiple astrocytes, but so far direct evidence is lacking. Each cluster comprised 1–15 astrocytes (average, 3.6; Magavi et al., 2012). The phenomenon of multiple astrocytes in a single cluster strongly indicates active proliferation of astrocytes within the cortex shortly after their transformation from radial glia. This phenomenon is consistent with time-lapse imaging results from brain slices and in vivo results showing that astrocytes enter the cell cycle and proliferate locally in cortical layers (Burns et al., 2009; Ge et al., 2012). Based on the observations of Magavi et al., radial glia contribute 1 of every 3.6 astrocytes (~30%) present in the mature cerebral cortex. It is also possible that one radial glia transforms into one astrocyte progenitor (Noctor et al., 2004). These astrocyte progenitors are migratory and retain the capacity to proliferate (Burns et al., 2009; Ge et al., 2012), and consequently a single radial glia–derived progenitor can produce clusters with multiple astrocytes in the cortex (Fig. 1).
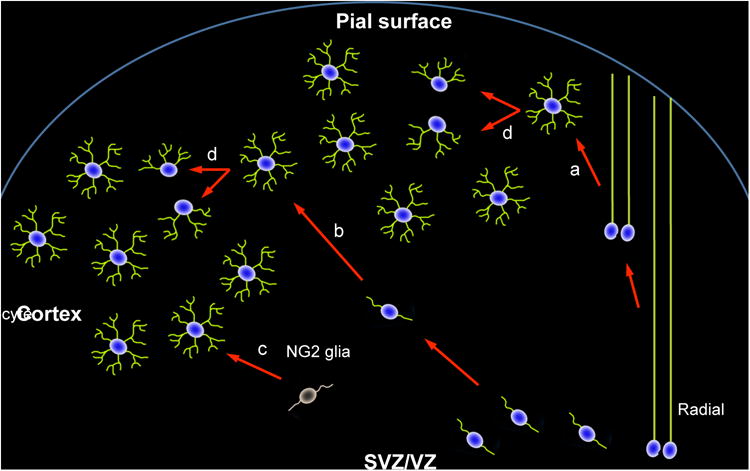
There are four astrocyte sources in the cerebral cortex: radial glia, SVZ-derived progenitors, locally proliferating glia, and NG2 glia. (a) Radial glia translocate from the VZ to the cortex and become immature astrocytes by retracting their long leading processes. (b) Astrocytes derived from the SVZ migrate into the cerebral cortex likely along radial glial shafts. Most radial glia in rodents start to disappear during late embryonic stages, and few remain after postnatal week 2. (c) NG2 glia produce a portion of astrocytes in the ventral cortex of the forebrain. (d) Locally proliferating astrocytes in the cortex undergo symmetric division to generate additional astrocytes. These locally produced astrocytes are a major astrocyte source in the postnatal cortex. Note: locally proliferating astrocytes are originally produced from progenitors in the SVZ and radial glia.
SVZ progenitor–derived astrocytes and their contribution to the total astrocyte population
Abundant evidence has shown that SVZ progenitors produce both astrocytes and oligodendrocytes in the postnatal rodent cerebral cortex (Smart, 1961; Lewis, 1968; Privat and Leblond, 1972; Paterson et al., 1973, 1983; Levison et al., 1993; Levison and Goldman, 1993; Marshall and Goldman, 2002; Burns et al., 2009). Retroviral infection is frequently used to analyze the progeny of the labeled SVZ progenitors in vivo. Because only a small portion of SVZ-derived progenitors are labeled with this method, however, direct evidence demonstrating the contribution of SVZ-derived astrocytes to the overall astrocyte population is still needed. The difficulty of traditional methods, such as [3H]thymidine or BrdU labeling, is that they cannot be used to distinguish the contribution of SVZ-derived astrocytes from that of radial glia–derived astrocytes and locally produced astrocytes.
Astrocytes derived from the SVZ are believed to migrate to the cerebral cortex along radial glial shafts, as do projection neurons in embryos. In rodents, most radial glia start to disappear beginning in the late embryonic stages, and few remain after postnatal week 2. Shortly after birth, many axons pass through the white matter in the rodent forebrain (Wang et al., 2007; Zhou et al., 2013) and likely form a physical barrier to astrocyte migration after disappearance of radial glia; moreover, the number of SVZ progenitor–derived astrocytes that migrate into the cortex with assistance from radial glia also decreases substantially (Burns et al., 2009). Our group used electroporation to label both SVZ-derived progenitors and VZ radial glia in P0–2 mice and analyzed their progeny 1–2 weeks later. Approximately 25% of astrocytes derived from the VZ and SVZ migrated into six cortical layers, and ~75% remained in the SVZ and white matter (Ge et al., 2012). After P14, SVZ progenitor– derived astrocytes in rats do not colonize the cerebral cortex (Levison et al., 1993). Because no good method has been developed to efficiently label all SVZ progenitors, we still do not know the percentage of SVZ progenitor–derived astrocytes that contribute to the entire astrocyte population in the postnatal cortex.
NG2 glia-derived astrocytes and their contribution the total astrocyte population
NG2 glia account for 5–8% of the glial population (Levine et al., 2001) and form synapses with neurons (Bergles et al., 2000; Lin and Bergles, 2004). These glia are the major dividing cells in the nervous system outside of neurogenic regions in the adult rodent brain (Levine et al., 1993; Horner et al., 2000; Dawson, 2003; Kukley et al., 2008; Ge et al., 2009). After pulse-chase with BrdU, 70–75% of BrdU+ cells in the rat cerebral cortex were found to be NG2 glia (Dawson et al., 2003). It is well established that NG2 glia have the potential to produce both oligodendrocytes and astrocytes in vitro (Raff et al., 1983). Recently, NG2 glia were found to produce astrocytes in the ventrolateral forebrain of NG2CreBac;Z/EG mice, including the temporal cortex, ventrolateral stratum, septum, hippocampus, and thalamus (Zhu et al., 2008). NG2 glia–derived astrocytes contributed 18% and 36% of all astrocytes in the ventral cortical regions of the anterior and posterior forebrain, respectively; however, very few astrocytes were derived from NG2 glia in the dorsal cortex (Zhu et al., 2008). Moreover, because NG2 glia of these NG2CreBac;Z/EG mice began producing astrocytes in the late embryonic stage (Zhu et al., 2008), two independent groups did fate mapping by crossing PdgfraCreER mice with different reporter lines including Rosa26-YFP, Z/EG, and ROSA26-mGFP. They administrated tamoxifen at P4, P30, P45, and P180 and then analyzed the progeny after days or months to analyze whether NG2 glia produced astrocytes in the postnatal brain (Revers et al., 2008; Kang et al., 2010; Clarke et al., 2012). Interestingly, both groups found that astrocytes were not produced from NG2 glia in the cerebral cortex. However, another group used Plp-Cre-ERT2;Rosa26-EYFP (Plp implies proteolipid) mice and obtained different results (Guo et al., 2009). Plp promoter activity is restricted to the oligodendrocyte lineage (Doerflinger et al., 2003). When Guo et al. administered mice with tamoxifen at P7 and carried out immunochemistry at P15, they observed that astrocytes from NG2 glia were distributed in the ventral forebrain including the piriform cortex, amygdala, and hypothalamus. Of the astrocytes in the ventral cortex, 15.9% were from NG2 glia in these mice (Guo et al., 2009). Interestingly, no astrocytes were seen from NG2 glia in the dorsal cortex, which is consistent with the results from NG2creBac;Z/EG (Zhu et al., 2008). In short, the contribution of astrocytes derived from the oligodendroglial lineage to dorsal cortical astrocytes is zero and to ventral cortex is possibly small in the postnatal rodent brain.
Local proliferation of glia in the developing cortex
Cell proliferation in the cortex has been reported for over a century in different species including dogs, cats, rats, and mice (Buchholtz, 1890; Sclavunos, 1899; Hamilton, 1901; Addison, 1911; Allen, 1912). The huge advancements in the study of radial glia and SVZ progenitors have drawn much attention from researchers in the past three decades, but the importance of local glial production has been neglected. There are two peaks of local proliferation of glia in the rat cerebral cortex after birth: the first is at P3–7, and the second is at P16 (Allen, 1912; Ichikawa et al., 1983). Dividing astrocytes mainly contribute to the first peak (Ge et al., 2012), and dividing NG2 glia mainly contribute to the second peak (Levison et al., 1993; Zerlin et al., 1995; Parnavelas et al., 1999; Kukley et al., 2008; Ge et al., 2009, 2012). The abundance of locally proliferating glia varies in different layers. In P0 rat brain, more dividing glia (~70%) are located within inner layers of the cerebral cortex. However, this is reversed in the P4 brain, in which ~70% of all dividing cells from the cerebral cortex are located in outer layers. At P6–P8, there is no significant difference in the density of proliferating cells between layers (Ichikawa et al., 1983). It remains unknown why astrocyte proliferation peaks during postnatal week 1 but then ceases shortly after week 2 in the rodent brain.
Glia continue to proliferate locally in adult mice
Although cell proliferation outside the SVZ and VZ is quite rare in the adult brain, cell division in glia occurs in nearly all major rodent brain regions including the cerebral cortex, corpus callosum, stratum, hypothalamus, and septum (Messier et al., 1958; Walker and Leblond, 1958; Hain et al., 1961; Smart and Leblond, 1961; Dalton, et al., 1968; Dawson et al., 2003). The mean percentage of dividing cells at four ages (at age 23, 100, 200, and 400 days) in mouse brain was determined to be: 0.142% in the septum, 0.445% in the corpus callosum, 0.048% in the corpus stratum, 0.058 in the hypothalamus and 0.090% in the cerebral cortex (Dalton et al., 1968).
Dividing astrocytes can be identified via electron microscopy after [3H]thymidine labeling (Kaplan and Hinds 1980; Reyners et al., 1986). Thirty days after one injection of [3H]thymidine, 0.077% of astrocytes in the rat visual cortex underwent division and were labeled (Kaplan and Hinds, 1980). With Ki67 staining in hGFAP-GFP mouse cortical sections, our group observed that 0.30% of astrocytes were undergoing cell division at P48–52 (Ge et al., 2012).
Frequency of local proliferation of glial cells
Do astrocytes undergo cell division multiple times within the first two postnatal weeks in rodents? Clusters with large numbers of glia labeled with 3H]thymidine (with subsequent visualization via autoradiography) could be observed in the cerebral cortex of both young adult rat and cat brain (Altman, 1963). Retrovirus-mediated gene transfer is an ideal tool for lineage tracing because replication-incompetent retroviruses can be used to introduce new genes (e.g., lacZ or EGFP) into the genome of dividing cells (Turner and Cepko, 1987). The progeny of infected mother cells retain these marker genes. Ventricular cells were labeled via retroviruses at E16 and clonal analysis carried out at P14; clones with 2–3 closely packed glia were observed within the rat cortex, and these clones were produced by local glial proliferation after they migrated into the cortex (Price and Thurlow, 1988). A similar phenomenon was also reported in glial clusters by labeling SVZ cells with two retroviruses at extremely low multiplicity of infection that expressed two different markers to ensure that each cluster of cells was derived from an individual cell after its progeny migrated into the rat cerebral cortex (Levison and Goldman, 1993). The study yielded very interesting results from one of the Thy1.2-Cre mouse lines in that there was a low rate of recombination after the line was crossed with a reporter line (Magavi et al., 2012). In the progeny, Magavi et al. found that an individual column of cells was produced from a single progenitor or radial glia. Most labeled cortical columns contained astrocyte clusters (a group of GFP+ astrocytes within 25 μm of another GFP+ astrocyte). Each cluster contained an average of 3.6 astrocytes, indicating that cortical astrocytes entered the cell cycle approximately two times within the cortex. At 2 to 4 days after dividing, astrocytes in the cerebral cortex can be labeled using GFP-encoding retroviruses (Ge et al., 2012), and ~10% of the retrovirus-infected astrocytes (i.e., that had divided) can be stained by an antibody against Ki67 (Ge et al., 2012). These observations suggest that some astrocytes enter the cell cycle again shortly after their initial division. In glia, DNA synthesis during S-phase lasts ~10 hours (Korr et al., 1973), and the subsequent G2 lasts 2–3 hours (Hommes and Leblond, 1967; Korr et al., 1973). Dividing astrocytes complete mitosis (from metaphase to telophase) in 2–3 hours (Ge et al., 2012), and the time needed for an astrocyte to complete one cell cycle is less than 24 hours in the developing mouse brain (Burns et al., 2009). Therefore, cortical astrocytes can potentially amplify their number 6- to 8-fold via local proliferation within a period of 1–2 weeks. Whether all astrocytes in the cerebral cortex—or only a small percentage—have comparable potential to frequently divide remains an open question.
Locally produced astrocytes and their contribution to the total astrocyte population
Although we have known about local glial proliferation in the brain for a very long time, owing to the diversity of astrocytes from different sources, it was not until recently that we started to understand that the local production of glia is a major source of astrocytes in the cerebral cortex. The main challenge to studying astrocyte generation in the cortex is the lack of specific markers for labeling cortical astrocytes. To date, the efficient way of identifying cortical astrocytes is to fluorescently label them by expressing an exogenous gene such as GFAP-GFP (Zhuo et al., 1997, Matthias et al., 2003) or Aldh1L1-GFP (Heintz, 2001) under control of the respective promoter. Alternatively, some researchers have used Aldh1l1-Cre and GFAP-CreER to label astrocytes after a mouse line is crossed with an appropriate reporter line (Gong et al., 2003; Casper et al., 2007; Chow et al., 2008; Ge et al., 2012; Tien et al., 2012; Tsai et al., 2012). In such genetic labeling methods, the astrocytes must be distinguished from other glia types (especially NG2 glia) using another method such as electrophysiology or immunostaining (Matthias et al., 2003; Ge et al., 2012). To determine the contribution of astrocytes derived from local proliferation, we injected retroviruses having a high titer into the cerebral cortex of P0–2 mice with subsequent comparison of the number of virally infected astrocytes with the total number of astrocytes within an infected region after 1 week post-infection. We found that approximately half (46.8%) of the astrocytes were locally produced. Because the half-life of infectivity of the retrovirus we used is nearly 8 hours at 37 °C and retroviruses likely cannot infect all dividing cells, the actual contribution of astrocytes from local production is likely >46.8%. These results demonstrate that local production accounts for a major portion of astrocytes in the postnatal cerebral cortex (Fig. 1).
Although astrocyte generation has been studied for more than a century, certain fundamental questions remain unclear including the following. (1) What is the molecular mechanism underlying the difference in astrocyte sources from different brain regions and different developmental stages? (2) What is the role of neuronal activity in astrocyte production? (3) What mechanisms underlie the interaction between astrocytes and vascular cells and the formation of astrocytic endfeet in the developing brain? New techniques and approaches for glia-specific studies would greatly enhance the ability of researchers to answer these questions.
Table 1
Sources | Methods | Species | References |
---|---|---|---|
| |||
Radial glia | Golgi staining | Monkey | Schmechel and Rakic, 1979 |
Labeling with DiI | Ferret | Voigt 1989 | |
Labeling with dyes (DiI/DiA) | Human (fetus) | deAzevedo et al., 2003 | |
Organotypic slice cultures and time-lapse imaging | Rat | Noctor et al., 2004 | |
Organotypic slice cultures and time-lapse imaging | Mouse | Burns et al., 2009 | |
Genetic tracing | Mouse | Magavi et al., 2012 | |
Adenovirus-Cre infection | Mouse | Tsai et al., 2012 | |
SVZ progenitors | Radioautography | Mouse | Smart, 1961 |
Radioautography | Rat | Lewis, 1968 | |
Radioautography | Rat | Paterson et al., 1973 | |
Radioautography | Mouse | Paterson, 1983 | |
Retroviral labeling | Rat | Levison et al., 1993 | |
Retroviral labeling | Rat | Levison and Goldman, 1993 | |
Retroviral labeling | Mouse | Marshall and Goldman, 2002 | |
NG2 glia | Genetic tracing | Mouse | Zhu et al., 2008 |
Genetic tracing | Mouse | Guo et al., 2009 | |
Locally proliferating glia | Radioautography | Rat | Kaplan and Hinds, 1980 |
Retroviral labeling | Rat | Price and Thurlow, 1988 | |
Retroviral labeling | Rat | Levison and Goldman, 1993 | |
Organotypic slice culture and time-lapse imaging | Mouse | Burns et al., 2009 | |
In vivo imaging, retroviral labeling, and genetic tracing | Mouse | Ge et al., 2012 | |
Genetic tracing | Mouse | Magavi et al., 2012 |
Acknowledgments
We thank Wei Zhou (Huazhong University of Science and Technology), Edward Kim (UT Southwestern), Grant Li (UCSF), and Chao Guo (UT Southwestern) for their critical reading of the manuscript. This work was supported by a NINDS K99/R00 award (1K99NS073735) and startup funds from the Children's Research Institute, UT Southwestern Medical Center, to W.-P.G.
Footnotes
Publisher's Disclaimer: This is a PDF file of an unedited manuscript that has been accepted for publication. As a service to our customers we are providing this early version of the manuscript. The manuscript will undergo copyediting, typesetting, and review of the resulting proof before it is published in its final citable form. Please note that during the production process errors may be discovered which could affect the content, and all legal disclaimers that apply to the journal pertain.
References
- Addison WHF. The development of the Purkinje cells and of the cortical layers in the cerebellum of the albino rat. J Comp Neurol. 1911;21:459–489. [Google Scholar]
- Allen E. The cessation of mitosis in the central nervous system of the albino rat. J Comp Neurol. 1912;22:547–568. [Google Scholar]
- Altman J. Autoradiographic investigation of cell proliferation in the brains of rats and cats. Anat Rec. 1963;145:573–591. [Abstract] [Google Scholar]
- Bandeira F, Lent R, Herculano-Houzel S. Changing numbers of neuronal and non-neuronal cells underlie postnatal brain growth in the rat. Proc Natl Acad Sci U S A. 2009;106:14108–14113. [Europe PMC free article] [Abstract] [Google Scholar]
- Bergles DE, Roberts JD, Somogyi P, Jahr CE. Glutamatergic synapses on oligodendrocyte precursor cells in the hippocampus. Nature. 2000;405:187–191. [Abstract] [Google Scholar]
- Brizzee KR, Jacobs LA. The glia/neuron index in the submolecular layers of the motor cortex in the cat. Anat Rec. 1959;134:97–105. [Abstract] [Google Scholar]
- Buchholtz A. Ueber das vorkommen von Karyokinesen in Zellen des Centernervensystems von neugeborenen und jungen Hunden u. Kanininchen. Neur Centralblatt Bd. 1890;9:140–142. [Google Scholar]
- Burns KA, Murphy B, Danzer SC, Kuan CY. Developmental and post-injury cortical gliogenesis: a genetic fate-mapping study with Nestin-CreER mice. Glia. 2009;57:1115–1129. [Europe PMC free article] [Abstract] [Google Scholar]
- Cajal SRy. Histologie du Système Nerveux de l'Homme et des Vertébrés. 1911 French edition reviewed and updated by the author, translated from Spanish by L. Azoulay. [Google Scholar]
- Casper KB, Jones K, McCarthy KD. Characterization of astrocyte-specific conditional knockouts. Genesis. 2007;45:292–299. [Abstract] [Google Scholar]
- Choi BH, Lapham LW. Radial glia in the human fetal cerebrum: A combined golgi, immunofluorescent and electron microscopic study. Brain Res. 1978;149:295–311. [Abstract] [Google Scholar]
- Chow LM, Zhang J, Baker SJ. Inducible Cre recombinase activity in mouse mature astrocytes and adult neural precursor cells. Transgenic Res. 2008;17:919–928. [Europe PMC free article] [Abstract] [Google Scholar]
- Clarke LE, Young KM, Hamilton NB, Li H, Richardson WD, Attwell D. Properties and fate of oligodendrocyte progenitor cells in the corpus callosum, motor cortex, and piriform cortex of the mouse. J Neurosci. 2012;32:8173–8185. [Europe PMC free article] [Abstract] [Google Scholar]
- Dalton MM, Hommes OR, Leblond CP. Correlation of glial proliferation with age in the mouse brain. J Comp Neurol. 1968;134:397–400. [Abstract] [Google Scholar]
- Dawson MR, Polito A, Levine JM, Reynolds R. NG2-expressing glial progenitor cells: an abundant and widespread population of cycling cells in the adult rat CNS. Mol Cell Neurosci. 2003;24:476–488. [Abstract] [Google Scholar]
- deAzevedo LC, Fallet C, Moura-Neto V, Daumas-Duport C, Hedin-Pereira C, Lent R. Cortical radial glial cells in human fetuses: depth-correlated transformation into astrocytes. J Neurobiol. 2003;55:288–98. [Abstract] [Google Scholar]
- Doerflinger NH, Macklin WB, Popko B. Inducible site-specific recombination in myelinating cells. Genesis. 2003;35(1):63–72. [Abstract] [Google Scholar]
- Ge WP, Miyawaki A, Gage FH, Jan YN, Jan LY. Local generation of glia is a major astrocyte source in postnatal cortex. Nature. 2012;484:376–380. [Europe PMC free article] [Abstract] [Google Scholar]
- Ge WP, Zhou W, Luo Q, Jan LY, Jan YN. Dividing glial cells maintain differentiated properties including complex morphology and functional synapses. Proc Natl Acad Sci U S A. 2009;106:328–33. [Europe PMC free article] [Abstract] [Google Scholar]
- Geha S, Pallud J, Junier MP, Devaux B, Leonard N, Chassoux F, Chneiweiss H, Daumas-Duport C, Varlet P. NG2+/Olig2+ cells are the major cycle-related cell population of the adult human normal brain. Brain Pathol. 2010;20:399–411. [Europe PMC free article] [Abstract] [Google Scholar]
- Gong S, Zheng C, Doughty ML, Losos K, Didkovsky N, Schambra UB, Nowak NJ, Joyner A, Leblanc G, Hatten ME, Heintz N. A gene expression atlas of the central nervous system based on bacterial artificial chromosomes. Nature. 2003;425:917–925. [Abstract] [Google Scholar]
- Gressens P, Richelme C, Kadhim HJ, Gadisseux JF, Evrard P. The germinative zone produces the most cortical astrocytes after neuronal migration in the developing mammalian brain. Biol Neonate. 1992;61:4–24. [Abstract] [Google Scholar]
- Guo F, Ma J, McCauley E, Bannerman P, Pleasure D. Early postnatal proteolipid promoter-expressing progenitors produce multilineage cells in vivo. J Neurosci. 2009;29:7256–7270. [Europe PMC free article] [Abstract] [Google Scholar]
- Freeman MR. Specification and morphogenesis of astrocytes. Science. 2010;330:774–778. [Europe PMC free article] [Abstract] [Google Scholar]
- Hain RF, Rieke WO, Everett NB. Evidence of mitosis in neuroglia as revealed by radioautography employing tritiated thymidine. J Neuropathol Exp Neurol. 1961;20:141–157. [Google Scholar]
- Hamilton A. The division of differentiated cells in the central nervous system of the white rat. J Comp Neurol. 1901;11:297–320. [Google Scholar]
- Heintz N. BAC to the future: the use of bac transgenic mice for neuroscience research. Nat Rev Neurosci. 2001;2:861–870. [Abstract] [Google Scholar]
- Hommes OR, Leblond CP. Mitotic division of neuroglia in the normal adult rat. J Comp Neurol. 1967;129:269–278. [Abstract] [Google Scholar]
- Horner PJ, Power AE, Kempermann G, Kuhn HG, Palmer TD, Winkler J, Thal LJ, Gage FH. Proliferation and differentiation of progenitor cells throughout the intact adult rat spinal cord. J Neurosci. 2000;20:2218–2228. [Europe PMC free article] [Abstract] [Google Scholar]
- Ichikawa M, Shiga T, Hirata Y. Spatial and temporal pattern of postnatal proliferation of glial cells in the parietal cortex of the rat. Dev Brain Res. 1983;9:181–187. [Abstract] [Google Scholar]
- Jones EG. GABAergic neurons and their role in cortical plasticity in primates. Cereb Cortex. 1993;3:361–372. [Abstract] [Google Scholar]
- Kang SH, Fukaya M, Yang JK, Rothstein JD, Bergles DE. NG2+ CNS glial progenitors remain committed to the oligodendrocyte lineage in postnatal life and following neurodegeneration. Neuron. 2010;68:668–681. [Europe PMC free article] [Abstract] [Google Scholar]
- Kaplan MS, Hinds JW. Gliogenesis of astrocytes and oligodendrocytes in the neocortical grey and white matter of the adult rat: electron microscopic analysis of light radioautographs. J Comp Neurol. 1980;193:711–727. [Abstract] [Google Scholar]
- Korr H, Schultze B, Maurer W. Autoradiographic investigations of glial proliferation in the brain of adult mice. I. The DNA synthesis phase of neuroglia and endothelial cells. J Comp Neurol. 1973;150:169–175. [Abstract] [Google Scholar]
- Kukley M, Kiladze M, Tognatta R, Hans M, Swandulla D, Schramm J, Dietrich D. Glial cells are born with synapses. FASEB J. 2008;22:2957–2969. [Abstract] [Google Scholar]
- Levine JM, Stincone F, Lee YS. Development and differentiation of glial precursor cells in the rat cerebellum. Glia. 1993;7:307–321. [Abstract] [Google Scholar]
- Levine JM, Reynolds R, Fawcett JW. The oligodendrocyte precursor cell in health and disease. Trends Neurosci. 2001;24:39–47. [Abstract] [Google Scholar]
- Levison SW, Chuang C, Abramson BJ, Goldman JE. The migrational patterns and developmental fates of glial precursors in the rat subventricular zone are temporally regulated. Development. 1993;119:611–622. [Abstract] [Google Scholar]
- Levison SW, Goldman JE. Both oligodendrocytes and astrocytes develop from progenitors in the subventricular zone of postnatal rat forebrain. Neuron. 1993;10:201–212. [Abstract] [Google Scholar]
- Lewis PD. The fate of the subependymal cell in the adult rat brain, with a note on the origin of microglia. Brain. 1968;91:721–738. [Abstract] [Google Scholar]
- Levitt P, Rakic P. Immunoperoxidase localization of glial fibrillary acidic protein in radial glial cells and astrocytes of the developing rhesus monkey brain. J Comp Neurol. 1980;193:815–840. [Abstract] [Google Scholar]
- Lin SC, Bergles DE. Synaptic signaling between GABAergic interneurons and oligodendrocyte precursor cells in the hippocampus. Nat Neurosci. 2004;7:24–32. [Abstract] [Google Scholar]
- Magavi S, Friedmann D, Banks G, Stolfi A, Lois C. Coincident generation of pyramidal neurons and protoplasmic astrocytes in neocortical columns. J Neurosci. 2012;32:4762–4772. [Europe PMC free article] [Abstract] [Google Scholar]
- Marshall CA, Goldman JE. Subpallial dlx2-expressing cells give rise to astrocytes and oligodendrocytes in the cerebral cortex and white matter. J Neurosci. 2002;22:9821–9830. [Europe PMC free article] [Abstract] [Google Scholar]
- Matthias K, Kirchhoff F, Seifert G, Hüttmann K, Matyash M, Kettenmann H, Steinhäuser C. Segregated expression of AMPA-type glutamate receptors and glutamate transporters defines distinct astrocyte populations in the mouse hippocampus. J Neurosci. 2003;23:1750–1758. [Europe PMC free article] [Abstract] [Google Scholar]
- Messier B, Leblond CP, Smart I. Presence of DNA synthesis and mitosis in the brain of young adult mice. 1958;14:224–226. [Abstract] [Google Scholar]
- Noctor SC, Martínez-Cerdeño V, Ivic L, Kriegstein AR. Cortical neurons arise in symmetric and asymmetric division zones and migrate through specific phases. Nat Neurosci. 2004;7:136–144. [Abstract] [Google Scholar]
- Paterson JA. Dividing and newly produced cells in the corpus callosum of adult mouse cerebrum as detected by light microscopic radioautography. Anat Anz. 1983;153:149–168. [Abstract] [Google Scholar]
- Paterson JA, Privat A, Ling EA, Leblond CP. Investigation of glial cells in semithin sections. 3. Transformation of subependymal cells into glial cells, as shown by radioautography after 3 Hthymidine injection into the lateral ventricle of the brain of young rats. J Comp Neurol. 1973;149:83–102. [Abstract] [Google Scholar]
- Parnavelas JG. Glial cell lineages in the rat cerebral cortex. Exp Neurol. 1999;156:418–429. [Abstract] [Google Scholar]
- Price J, Thurlow L. Cell lineage in the rat cerebral cortex: a study using retroviral-mediated gene transfer. Development. 1988;104:473–482. [Abstract] [Google Scholar]
- Privat A, Leblond CP. The subependymal layer and neighboring region in the brain of the young rat. J Comp Neurol. 1972;146:277–302. [Abstract] [Google Scholar]
- Raff MC, Miller RH, Noble M. A glial progenitor cell that develops in vitro into an astrocyte or an oligodendrocyte depending on culture medium. Nature. 1983;303:390–396. [Abstract] [Google Scholar]
- Rakic P. Mode of cell migration to the superficial layers of fetal monkey neocortex. Journal of Comparative Neurology. 1972;145:61–83. [Abstract] [Google Scholar]
- Rakic P. Developmental and evolutionary adaptations of cortical radial glia. Cereb Cortex. 2003;13(6):541–9. [Abstract] [Google Scholar]
- Reyners H, Gianfelici de Reyners E, Regniers L, Maisin JR. A glial progenitor cell in the cerebral cortex of the adult rat. J Neurocytol. 1986;15:53–61. [Abstract] [Google Scholar]
- Rivers LE, Young KM, Rizzi M, Jamen F, Psachoulia K, Wade A, Kessaris N, Richardson WD. PDGFRA/NG2 glia generate myelinating oligodendrocytes and piriform projection neurons in adult mice. Nat Neurosci. 2008;11:1392–1401. [Europe PMC free article] [Abstract] [Google Scholar]
- Sauvageot CM, Stiles CD. Molecular mechanisms controlling cortical gliogenesis. Curr Opin Neurobiol. 2002;12:244–249. [Abstract] [Google Scholar]
- Schmechel DE, Rakic P. A golgi study of radial glial cells in developing monkey telencephalon: morphogenesis and transformation into astrocytes. Anat Embryol. 1979;156:115–152. [Abstract] [Google Scholar]
- Sclavunos G. über Keimzellen in der weissen Substanz des Rückenmarks von alteren Embryonen und Neugeborenen. Anat Anzeiger Bd. 1899;16:467–473. [Google Scholar]
- Smart I. The subependymal layer of the mouse brain and its cell production as shown by radioautography after thymidine-H3 injection. J Comp Neurol. 1961;116:325–347. [Google Scholar]
- Smart I, Leblond CP. Evidence for division and transformations of neuroglia cells in the mouse brain, as derived from radioautography after injection of thymidine-H3. J Comp Neurol. 1961;116:349–367. [Google Scholar]
- Tien AC, Tsai HH, Molofsky AV, McMahon M, Foo LC, Kaul A, Dougherty JD, Heintz N, Gutmann DH, Barres BA, Rowitch DH. Regulated temporal-spatial astrocyte precursor cell proliferation involves BRAF signalling in mammalian spinal cord. Development. 2012;139:2477–2487. [Europe PMC free article] [Abstract] [Google Scholar]
- Tsai HH, Li H, Fuentealba LC, Molofsky AV, Taveira-Marques R, Zhuang H, Tenney A, Murnen AT, Fancy SP, Merkle F, Kessaris N, Alvarez-Buylla A, Richardson WD, Rowitch DH. Regional astrocyte allocation regulates CNS synaptogenesis and repair. Science. 2012;337:358–362. [Europe PMC free article] [Abstract] [Google Scholar]
- Turner DL, Cepko CL. A common progenitor for neurons and glia persists in rat retina late in development. Nature. 1987;328:131–136. [Abstract] [Google Scholar]
- Wang CL, Zhang L, Zhou Y, Zhou J, Yang XJ, Duan SM, Xiong ZQ, Ding YQ. Activity-dependent development of callosal projections in the somatosensory cortex. J Neurosci. 2007;27:11334–11342. [Europe PMC free article] [Abstract] [Google Scholar]
- Zerlin M, Levison SW, Goldman JE. Early patterns of migration, morphogenesis, and intermediate filament expression of subventricular zone cells in the postnatal rat forebrain. J Neurosci. 1995;15:7238–7249. [Europe PMC free article] [Abstract] [Google Scholar]
- Zhu X, Bergles DE, Nishiyama A. NG2 cells generate both oligodendrocytes and gray matter astrocytes. Development. 2008;135:145–157. [Abstract] [Google Scholar]
- Zhou J, Wen Y, She L, Sui YN, Liu L, Richards LJ, Poo MM. Axon position within the corpus callosum determines contralateral cortical projection. Proc Natl Acad Sci U S A. 2013;110:2714–2723. [Europe PMC free article] [Abstract] [Google Scholar]
- Zhuo L, Sun B, Zhang CL, Fine A, Chiu SY, Messing A. Live astrocytes visualized by green fluorescent protein in transgenic mice. Dev Biol. 1997;187:36–42. [Abstract] [Google Scholar]
- Walker BE, Leblond CP. Sites of nucleic acid synthesis in the mouse visualized by radioautography after administration of C14-labelled adenine and thymidine. Exp Cell Res 1958. 1958;14:510–531. [Abstract] [Google Scholar]
- Wonders C, Anderson SA. Cortical interneurons and their origins. Neuroscientist. 2005;11:199–205. [Abstract] [Google Scholar]
- Voigt T. Development of glial cells in the cerebral wall of ferrets: direct tracing of their transformation from radial glia into astrocytes. J Comp Neurol. 1989;289:74–88. [Abstract] [Google Scholar]
Full text links
Read article at publisher's site: https://doi.org/10.1016/j.neuroscience.2015.08.057
Read article for free, from open access legal sources, via Unpaywall:
https://europepmc.org/articles/pmc4779064?pdf=render
Citations & impact
Impact metrics
Citations of article over time
Alternative metrics
Smart citations by scite.ai
Explore citation contexts and check if this article has been
supported or disputed.
https://scite.ai/reports/10.1016/j.neuroscience.2015.08.057
Article citations
Association between subcortical nuclei volume changes and cognition in preschool-aged children with tetralogy of Fallot after corrective surgery: a cross-sectional study.
Ital J Pediatr, 50(1):189, 19 Sep 2024
Cited by: 0 articles | PMID: 39300569 | PMCID: PMC11414171
Temporal-spatial Generation of Astrocytes in the Developing Diencephalon.
Neurosci Bull, 40(1):1-16, 16 Oct 2023
Cited by: 2 articles | PMID: 37843774 | PMCID: PMC10774245
Two-photon live imaging of direct glia-to-neuron conversion in the mouse cortex.
Neural Regen Res, 19(8):1781-1788, 02 Oct 2023
Cited by: 3 articles | PMID: 38103245 | PMCID: PMC10960291
δ-Catenin controls astrocyte morphogenesis via layer-specific astrocyte-neuron cadherin interactions.
J Cell Biol, 222(11):e202303138, 14 Sep 2023
Cited by: 7 articles | PMID: 37707499 | PMCID: PMC10501387
Epigenetic Alterations of Brain Non-Neuronal Cells in Major Mental Diseases.
Genes (Basel), 14(4):896, 12 Apr 2023
Cited by: 11 articles | PMID: 37107654 | PMCID: PMC10137903
Review Free full text in Europe PMC
Go to all (30) article citations
Similar Articles
To arrive at the top five similar articles we use a word-weighted algorithm to compare words from the Title and Abstract of each citation.
Local generation of glia is a major astrocyte source in postnatal cortex.
Nature, 484(7394):376-380, 28 Mar 2012
Cited by: 284 articles | PMID: 22456708 | PMCID: PMC3777276
GFAPdelta in radial glia and subventricular zone progenitors in the developing human cortex.
Development, 137(2):313-321, 01 Jan 2010
Cited by: 54 articles | PMID: 20040497
Effect of 6-hydroxydopamine (6-OHDA) on proliferation of glial cells in the rat cortex and striatum: evidence for de-differentiation of resident astrocytes.
Cell Tissue Res, 342(2):147-160, 27 Oct 2010
Cited by: 22 articles | PMID: 20976472
Astrocyte development and heterogeneity.
Cold Spring Harb Perspect Biol, 7(1):a020362, 20 Nov 2014
Cited by: 178 articles | PMID: 25414368 | PMCID: PMC4292163
Review Free full text in Europe PMC
Funding
Funders who supported this work.
Children’s Research Institute
NINDS (1)
Grant ID: R00NS073735
NINDS NIH HHS (3)
Grant ID: K99 NS073735
Grant ID: R00 NS073735
Grant ID: R00NS073735