Abstract
Free full text

Focus on Extracellular Vesicles: Therapeutic Potential of Stem Cell-Derived Extracellular Vesicles
Abstract
The intense research focus on stem and progenitor cells could be attributed to their differentiation potential to generate new cells to replace diseased or lost cells in many highly intractable degenerative diseases, such as Alzheimer disease, multiple sclerosis, and heart diseases. However, experimental and clinical studies have increasingly attributed the therapeutic efficacy of these cells to their secretion. While stem and progenitor cells secreted many therapeutic molecules, none of these molecules singly or in combination could recapitulate the functional effects of stem cell transplantations. Recently, it was reported that extracellular vesicles (EVs) could recapitulate the therapeutic effects of stem cell transplantation. Based on the observations reported thus far, the prevailing hypothesis is that stem cell EVs exert their therapeutic effects by transferring biologically active molecules such as proteins, lipids, mRNA, and microRNA from the stem cells to injured or diseased cells. In this respect, stem cell EVs are similar to EVs from other cell types. They are both primarily vehicles for intercellular communication. Therefore, the differentiating factor is likely due to the composition of their cargo. The cargo of EVs from different cell types are known to include a common set of proteins and also proteins that reflect the cell source of the EVs and the physiological or pathological state of the cell source. Hence, elucidation of the stem cell EV cargo would provide an insight into the multiple physiological or biochemical changes necessary to affect the many reported stem cell-based therapeutic outcomes in a variety of experimental models and clinical trials.
1. Introduction
Stem cells or progenitor cells are presently our best candidate therapeutic to treat intractable degenerative or genetic diseases through their capacity to engraft, differentiate, and generate new healthy cells to replace injured or diseased cells. This is best evidenced by the clinical success of hematopoietic stem cells as used in bone marrow transplantation to re-populate the recipient’s hematopoietic compartment with donor cells and treat a myriad of diseases such as cancer and genetic blood diseases such as thalassemia. Hence, the discovery of pluripotent stem cell (PSC) and non-hematopoietic tissue stem cells such as mesenchymal stem cell (MSC), neural stem cell (NSC), endothelial progenitor cell (EPC), or cardiac progenitor cells (CPC) has generated much optimism that non-hematopoietic diseases could also be similarly treated by replacing diseased cells with newly generated cells from pluripotent or tissue stem cells. Preliminary animal studies have demonstrated that many pluripotent or tissue stem cells have the potential to reduce the severity of many intractable degenerative or genetic non-hematopoietic diseases [1,2,3,4,5]. In fact, many of these stem or progenitor cells are being tested in clinical trials to treat many different diseases such as acute myocardial infarction (AMI), liver damage, ischemic kidney failure or stroke, amyotrophic lateral sclerosis (ALS), spinal cord injury, graft-versus-host disease (GVHD), etc. (Available online: http://www.clinicaltrials.gov).
1.1. Stem Cells
Stem cells (SCs) are generally defined as undifferentiated renewable cells that can differentiate into tissue-specific cell types with specialized functions. Currently, stem cells are divided into embryonic or “pluripotent” stem cells, and non-embryonic “somatic”, “adult” or “tissue” stem/progenitor cells. Embryonic stem cells (ESCs) are pluripotent stem cells derived from the inner cell mass of a blastocyst, an early-stage preimplantation embryo [6], and they are distinguished by two distinctive properties: pluripotency and the ability to replicate indefinitely. They are able to differentiate into the more than 200 cell-type derivatives of the three primary germ layers: ectoderm, endoderm, and mesoderm. Adult stem/progenitor cells, also known as somatic stem cells, are undifferentiated cells found throughout the body in juveniles as well as adults. These cells have large proliferative capacity and lineage-restricted differentiation potential, and could regenerate and contribute to physiological cell turnover [7,8,9,10]. To date, a large number of adult stem cells have been identified and isolated, and many have been reported to elicit therapeutic efficacy in animal studies and clinical trials. Some of these adult stem cells are MSCs, EPCs, CPCs, and NSCs. MSCs are multipotent stromal cells that can differentiate into a variety of mesodermal cell types [11] such as osteoblasts, chondrocytes, and adipocytes. They are also the most used stem cell type in clinical trials, primarily because they are multipotent, can be easily isolated from adult tissues, and have a large ex vivo expansion capacity [12]. EPCs, a subset of bone marrow-derived cells, are generally defined as circulating cells that express cell surface markers similar to those expressed by vascular endothelial cells, adhere to endothelium at sites of hypoxia/ischemia, and participate in new vessel formation [13,14]. CPCs are resident cardiac progenitor cells that are postulated to be derived from bone marrow or the embryonic cell population. They are thought to contribute to the physiological turnover of cardiac myocytes and vascular endothelial cells [15,16]. NSCs are self-renewing, multipotent cells that could be isolated from the fetal and adult brain, and have the potential to differentiate into neurons, astrocytes, and oligodendrocytes [17].
The use of embryonic versus adult stem cells for cell-based regenerative therapies has its own unique advantages and disadvantages [18]. Unlike adult stem cells whose ex vivo expansion capacity and differentiation potential are limited, embryonic stem cells have unlimited ex vivo expansion capacity and the potential to differentiate and replace almost every cell type in the adult body. However, adult stem cells are technically more amenable to our present regulatory framework and are ethically less controversial. In addition, the risk of immune rejection could be greatly reduced as adult stem cells could be harvested from the patient’s own body for ex vivo expansion and transplantation [19,20]. Their limited differentiation potential also mitigates the risk of forming aberrant or inappropriate tissues that could be deleterious, e.g., the formation of hard bone tissue in soft tissues like the brain. As such, the use of adult stem cells as therapeutic agents far exceeds that of ESCs and is currently being tested in the clinic against a large variety of disease indications.
1.2. Therapeutic Stem Cell Extracellular Vesicles (EVs)
Of the stem cells that are currently in clinical trials, the most widely used cell type is MSC and the other cell types are EPC, NSC and CPC (Available online: http://www.clinicaltrials.gov). The use of stem cells as therapeutics is often rationalized on their differentiation potential to generate replacement cell types. However this differentiation rationale was found to be increasingly inadequate, particularly for MSC which, being the widely used cell type, is also the best scrutinized. There are presently sufficient MSC studies to support an alternative proposal that MSC exerts its therapeutic effects through a secretion, and not a differentiation mechanism [12,21]. In many studies where functional improvement was reported after MSC transplantation, it was observed that migration, engraftment, and differentiation of MSCs at the sites of injury were rare [22,23,24], and involved <1% of transplanted cells [12]. It was also observed that migration of transplanted MSCs to the injured tissue is not necessary for efficacy [25,26,27,28].
The hypothesis that stem cells could exert therapeutic activity through their secretions is highly plausible as stem cell secretions are known to include many biologically potent molecules such as growth factors, cytokines, chemokines, and bioactive lipids that could elicit wide-ranging physiological effects [29]. This hypothesis was first validated for MSCs simply because they are the most studied stem cell type in therapeutic applications [21]. MSC-conditioned culture medium alone has been reported to recapitulate the efficacy of MSCs in cardioprotection [30,31,32], renal tubular cell survival [33], protection against fulminant hepatic failure [34,35], and immunomodulatory activity to alleviate immune disease [36]. However, it is unlikely that the capacity of MSCs in ameliorating complex and diverse tissue injuries such as myocardial ischemia/reperfusion injury or graft-versus-host disease could be attributed to a single molecular factor (reviewed [37,38]). Hence, EVs with their large and complex cargo of lipids, proteins, and RNAs are more likely candidates (reviewed [37,38]), and many stem cells are known to secrete EVs. Beside MSCs [39], ESCs [40], EPCs [41], NPCs [42], and CPCs [43] also secrete EVs. Some of the characteristics of EVs are comprehensively discussed in this focus edition by Kalra et al. [44].
With increasing evidence that EVs are major mediators of intercellular communication in many cell types [45,46], it is likely that EVs also perform similar functions for stem cells. As such, they would be expected to be significant in the hypothesis that stem cells exert therapeutic activity through their secretions by communicating therapeutic signals from stem cells to recipient cells to initiate repair and regeneration. The first therapeutically efficacious EVs secreted by stem cells were reported in 2009 when Bruno et al. reported that 180 nm MSC-derived microvesicles protect against acute tubular injury [39]. We subsequently reported that the smaller MSC-derived exosomes with a hydrodynamic radius of 55–65 nm also protect against acute myocardial ischemia/reperfusion injury [38,47], enhance wound healing [48], alleviate GVHD [49], reduce renal injury [50], and promote damaged hepatic regeneration [51]. Other groups have also reported the potential efficacy of MSC exosomes or EVs in treating other disease indications. For example, Xin et al. reported that MSC-derived exosomes promote neural plasticity and functional recovery in stroke via the transfer of miR-133b [52,53]. Katsuda et al. reported that human adipose MSC-derived exosomes contain functional neprilysin, a major β-amyloid peptide-degrading enzyme and, thus, have the potential to reduce the pathological accumulation of β-amyloid peptide in Alzheimer’s disease [54]. MSC-derived EVs were also found to protect against hypoxia- and endotoxin-induced lung injury [55,56]. More recently, EVs from CPCs have also been reported to be efficacious in cardiovascular disease [43,57,58,59]. EPC was reported to secrete EVs as early as 2007 [41], and these EVs enhance vascularization of xenotransplanted human islets in mice, alleviate renal ischemia/reperfusion injury in rats, and induce neovascularization in a murine model of hindlimb ischemia [60,61,62]. NSCs were first reported to secrete EVs in 2005 [42] and were shown in 2013 to promote neural plasticity and functional recovery after treatment of stroke. Together, these studies demonstrated that eutherapeutic outcomes of stem cell-treated tissue injury could be mediated by EVs [43,63,64,65] (as summarized in Table 1). Camussi et al. proposed that these EVs could potentially be home to target cells through receptors present on their surface such that, upon internalization, the cargo of EVs is loaded into diseased or injured target cells to initiate tissue repair and regeneration [66].
Table 1
Therapeutic role of stem cell EVs in various tissue injury models.
Author | Year | EV a Source | Disease or Assay Model | Therapeutic Effects | Ref. |
---|---|---|---|---|---|
Bruno et al. | 2009 | BM-MSC b | Glycerol-induced acute kidney injury (AKI) in SCID c mice | Protect against acute tubular injury | [39] |
Lai et al. | 2011 | ESC-MSC d | Myocardial ischemia/reperfusion injury | Protect against acute myocardial ischemia/reperfusion injury | [38] |
2010 | [47] | ||||
Zhang et al. | 2014 | UC-MSC e | Rat skin burn model | Accelerate skin damage repair | [48] |
Kordelas et al. | 2014 | BM-MSC | A therapy-refractory GVHD f patient | Improved the clinical GVHD symptoms significantly | [49] |
Bruno et al. | 2012 | BM-MSC | Lethal cisplatin-induced AKI in SCID mice | Exert a pro-survival effect | [50] |
Tan et al. | 2014 | ESC-MSC | Carbon tetrachloride (CCl4)-induced liver injury mouse model | Elicit hepatoprotective effects against toxicants-induced injury | [51] |
Xin et al. | 2012 | BM-MSC | Middle cerebral artery occlusion and reperfusion model | Promote neural plasticity and functional recovery | [52] |
2013 | [53] | ||||
Katsuda et al. | 2013 | ADSC g | Co-culture of N2a cells with ADSCs | Decrease β-amyloid peptide (Aβ) levels in the N2a cells | [54] |
Lee et al. | 2012 | UC-MSC | Murine model of hypoxic pulmonary hypertension | Exert a lung protection and inhibit pulmonary hypertension | [55] |
Zhu et al. | 2014 | BM-MSC | E. coli endotoxin-induced acute lung injury (ALI) in mice | Restore lung protein permeability and reduce inflammation | [56] |
Barile et al. | 2014 | CPC h | Rat acute myocardial infarction (AMI) model | Inhibit cardiomyocyte apoptosis and improve cardiac function | [43] |
Chen et al. | 2013 | CPC | Acute mouse myocardial ischemia/reperfusion (MI/R) model | Protect myocardium from acute MI/R injury | [57] |
Ibrahim et al. | 2014 | CPC | Acute and chronic myocardial infarction model in SCID mice | Enhance angiogenesis and promote cardiomyocyte survival | [58] |
Vrijsen et al. | 2010 | CPC | The in vitro scratch wound assay | Enhance migration of endothelial cells | [59] |
Ranghino et al. | 2012 | EPC i | Murine model of hindlimb ischemia in SCID mice | Induce neoangiogenesis and favor recovery | [60] |
Cantaluppi et al. | 2012 | EPC | Rat acute kidney ischemia-reperfusion injury model | Protect the kidney from ischemic acute injury | [61] |
Cantaluppi et al. | 2012 | EPC | Human islet transplantation model in SCID mice | Enhance insulin secretion, survival, and revascularization | [62] |
a EV: extracellular vesicles; b BM-MSC: bone marrow-derived mesenchymal stem cell; c SCID: severe combined immunodeficiency; d ESC-MSC: embryonic stem cell-derived mesenchymal stem cell; e UC-MSC: umbilical cord-derived mesenchymal stem cell; f GVHD: graft-versus-host disease; g ADSC: adipose tissue-derived mesenchymal stem cell; h CPC: cardiac progenitor cells; i EPC: endothelial progenitor cells.
2. Mechanisms Underlying the Therapeutic Potential of Stem Cell EVs
In general, the functions of stem cell-derived EVs do not differ much from those found in EVs derived from non-stem cells. Essentially, stem cell EVs, like other EVs, function to transfer lipids, nucleic acids, and proteins from one cell to another to elicit biological responses, and it is well documented that EVs from stem cells could indeed elicit biological responses from recipient cells that are consistent with the contents of the EVs. For example, Ratajczak et al. [67] demonstrated that EVs from mouse ESCs enhanced survival and expansion of hematopoietic progenitor cells, and upregulated early pluripotent (Oct-4, Nanog, and Rex-1) and early hematopoietic stem cell (Scl, HoxB4, and GATA 2) markers. They attributed these effects to the presence of Wnt-3 protein and mRNA for pluripotent transcription factors in the mESC-derived EVs [67] (as illustrated in Figure 1 and Table 2).
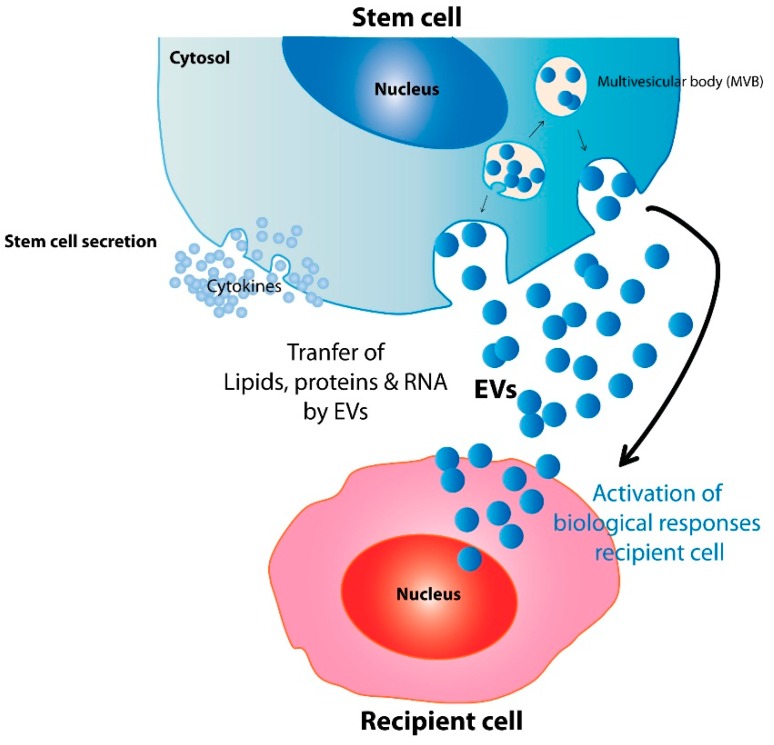
A proposed model for mechanisms underlying stem cell EV therapeutic potential. By delivery of lipids, proteins, and genetic information, stem cell-derived excellular vesicles (EVs) may biologically activate recipient cells to elicit relevant therapeutic effects.
Table 2
Examples of mechanisms underlying stem cell EV therapeutic potential.
EV Source | EV-Associated Active Contents | Biological Activities | Ref. |
---|---|---|---|
ESC | Wnt-3 protein and mRNA | Enhance hematopoietic progenitor cell survival and upregulate Oct-4, Nanog, Rex-1, Scl, HoxB4, and GATA 2 | [67] |
EPC | miR-126, miR-296 | Angiogenesis or protection against angiotensin II-induced cardiac hypertrophy and apoptosis | [41,60,61,68] |
CPC | miR-451, miR-146a | Protect against cardiac ischemia-reperfusion injury and recapitulate the regenerative and functional effects | [57,58] |
NSC | IFN-γ/Ifngr1 complexes | Induce interferon gamma signaling | [69] |
MSC | RNA/protein cargo | Protect against acute tubular injury and myocardial ischemia-reperfusion injury | [37,39,47,70,71,72,73] |
Like ESC-derived EVs, EPC-derived EVs were also biologically active and their RNA cargo were implicated in EV-elicited biological or therapeutic responses such as angiogenesis [41] or protection against angiotensin II (Ang II)-induced cardiac hypertrophy and apoptosis [68]. Subsequent studies specifically implicated miR-126 and miR-296 [60,61] as the RNAs responsible for the angiogenic effect of EPC-derived EVs. CPCs are also known to exert therapeutic effects through RNAs delivered by their EVs. CPC-derived EVs protect against ischemia-reperfusion injury by delivering miR-451 to injured cardiomyocytes [57] or miR-146a to recapitulate the regenerative and functional effects of CPC transplantation [58]. Recently, it was suggested that grafted NSCs communicate with the host immune system by inducing interferon gamma signaling through EV-associated IFN-γ/Ifngr1 complexes [69] (as illustrated in Figure 1 and Table 2).
The earliest stem cell reported to exert therapeutic effects through EVs is MSC, where MSC-derived EVs reportedly protect against acute tubular injury through their RNA cargo [39]. MSC-derived EVs also protect against myocardial ischemia-reperfusion injury [47]. Based on the cargo load of EVs [37,70,71], and the proteomic changes in the heart during myocardial ischemia-reperfusion injury [72], we hypothesize that MSC-derived EVs protect heart tissues against the injury by proteomic complementation to compensate for the proteomic alterations in myocardial ischemia-reperfusion injury and restore ATP production and induce survival signaling [73] (as illustrated in Figure 1 and Table 2).
We had also derived and characterized human fetal MSC and umbilical cord MSC as an alternative cell source for large-scale production of therapeutic cardioprotective EVs [74,75]. Consistent with the well-documented observation that the therapeutic efficacy of MSC is inversely correlated with the developmental stage of the donor, this correlation extended to the EVs. We observed that cord-derived MSC produced the least amount of therapeutic EVs, followed by fetal- and then ESC-derived MSC, suggesting that the inverse correlation between the therapeutic efficacy of MSC and the developmental stage of the donor is underpinned by the rate of EV production [75].
3. Conclusions
Stem cell EVs exert their therapeutic potential through the transfer of biologically active molecules in their vesicular cargo, which includes proteins, bioactive lipids, mRNA, and microRNA. The diversity of this EV cargo provides a rationale for the many reported stem cell-based therapeutic outcomes. There is now ample evidence of the effective recapitulation of the therapeutic efficacy of stem cells by their secreted EVs. This renders stem cell-derived extracellular vesicles a compelling alternative off-the-shelf, cell-free therapeutic modality that could be effective, safer, and cheaper. However, realizing this promising therapeutic modality of stem cell EVs would require extensive testing to validate their safety and efficacy.
For further information on the basic properties of EVs, their involvement in neurodegenerative and malignant diseases, their role in cell-cell communication, their potential as drug delivery vehicles, etc., the reader is referred to the various reviews in this focus edition [44,76,77,78,79].
Acknowledgments
This work has been funded by the Agency for Science, Technology and Research—Biomedical Research Council (A*STAR BMRC).
Author Contributions
Bin Zhang assisted in drafting the manuscript, Ronne Wee Yeh Yeo edited the tables, Kok Hian Tan critiqued and assisted in drafting the manuscript, and Sai Kiang Lim wrote and revised the review.
References
Articles from International Journal of Molecular Sciences are provided here courtesy of Multidisciplinary Digital Publishing Institute (MDPI)
Full text links
Read article at publisher's site: https://doi.org/10.3390/ijms17020174
Read article for free, from open access legal sources, via Unpaywall:
https://www.mdpi.com/1422-0067/17/2/174/pdf
Citations & impact
Impact metrics
Citations of article over time
Alternative metrics
Smart citations by scite.ai
Explore citation contexts and check if this article has been
supported or disputed.
https://scite.ai/reports/10.3390/ijms17020174
Article citations
Mesenchymal stem cell-derived extracellular vesicles therapy for primary ovarian insufficiency: a systematic review and meta-analysis of pre-clinical studies.
J Ovarian Res, 17(1):200, 14 Oct 2024
Cited by: 0 articles | PMID: 39402602 | PMCID: PMC11472498
Review Free full text in Europe PMC
Desktop-Stereolithography 3D Printing of a Decellularized Extracellular Matrix/Mesenchymal Stem Cell Exosome Bioink for Vaginal Reconstruction.
Tissue Eng Regen Med, 21(6):943-957, 27 Jun 2024
Cited by: 1 article | PMID: 38937423
The role of small extracellular vesicles and microRNA as their cargo in the spinal cord injury pathophysiology and therapy.
Front Neurosci, 18:1400413, 07 May 2024
Cited by: 0 articles | PMID: 38774785 | PMCID: PMC11106386
Review Free full text in Europe PMC
Stem Cell-Derived Extracellular Vesicles in the Treatment of Cardiovascular Diseases.
Pharmaceutics, 16(3):381, 11 Mar 2024
Cited by: 0 articles | PMID: 38543275 | PMCID: PMC10974254
Review Free full text in Europe PMC
Spinal Cord Injury: From MicroRNAs to Exosomal MicroRNAs.
Mol Neurobiol, 61(8):5974-5991, 23 Jan 2024
Cited by: 0 articles | PMID: 38261255
Review
Go to all (51) article citations
Other citations
Similar Articles
To arrive at the top five similar articles we use a word-weighted algorithm to compare words from the Title and Abstract of each citation.
The Role of Extracellular Vesicles as Paracrine Effectors in Stem Cell-Based Therapies.
Adv Exp Med Biol, 1201:175-193, 01 Jan 2019
Cited by: 17 articles | PMID: 31898787
Review
Stem Cells-Derived Extracellular Vesicles: Potential Therapeutics for Wound Healing in Chronic Inflammatory Skin Diseases.
Int J Mol Sci, 22(6):3130, 19 Mar 2021
Cited by: 14 articles | PMID: 33808520 | PMCID: PMC8003197
Review Free full text in Europe PMC
Focus on Extracellular Vesicles: Development of Extracellular Vesicle-Based Therapeutic Systems.
Int J Mol Sci, 17(2):172, 06 Feb 2016
Cited by: 70 articles | PMID: 26861303 | PMCID: PMC4783906
Review Free full text in Europe PMC
Extracellular Vesicles in Cardiovascular Theranostics.
Theranostics, 7(17):4168-4182, 26 Sep 2017
Cited by: 75 articles | PMID: 29158817 | PMCID: PMC5695004
Review Free full text in Europe PMC