Abstract
Free full text

Airborne Transmission of Influenza A/H5N1 Virus Between Ferrets
Abstract
Highly pathogenic avian influenza A/H5N1 virus can cause morbidity and mortality in humans but thus far has not acquired the ability to be transmitted by aerosol or respiratory droplet (“airborne transmission”) between humans. To address the concern that the virus could acquire this ability under natural conditions, we genetically modified A/H5N1 virus by site-directed mutagenesis and subsequent serial passage in ferrets. The genetically modified A/H5N1 virus acquired mutations during passage in ferrets, ultimately becoming airborne transmissible in ferrets. None of the recipient ferrets died after airborne infection with the mutant A/H5N1 viruses. Four amino acid substitutions in the host receptor-binding protein hemagglutinin, and one in the polymerase complex protein basic polymerase 2, were consistently present in airborne-transmitted viruses. The transmissible viruses were sensitive to the antiviral drug oseltamivir and reacted well with antisera raised against H5 influenza vaccine strains. Thus, avian A/H5N1 influenza viruses can acquire the capacity for airborne transmission between mammals without recombination in an intermediate host and therefore constitute a risk for human pandemic influenza.
Influenza A viruses have been isolated from many host species, including humans, pigs, horses, dogs, marine mammals, and a wide range of domestic birds, yet wild birds in the orders Anseriformes (ducks, geese, and swans) and Charadriiformes (gulls, terns, and waders) are thought to form the virus reservoir in nature (1). Influenza A viruses belong to the family Orthomyxoviridae; these viruses have an RNA genome consisting of eight gene segments (2, 3). Segments 1 to 3 encode the polymerase proteins: basic polymerase 2 (PB2), basic polymerase 1 (PB1), and acidic polymerase (PA), respectively. These proteins form the RNA-dependent RNA polymerase complex responsible for transcription and replication of the viral genome. Segment 2 also encodes a second small protein, PB1-F2, which has been implicated in the induction of cell death (4, 5). Segments 4 and 6 encode the viral surface glycoproteins hemagglutinin (HA) and neuraminidase (NA), respectively. HA is responsible for binding to sialic acids (SAs), the viral receptors on host cells, and for fusion of the viral and host cell membranes upon endocytosis. NA is a sialidase, responsible for cleaving SAs from host cells and virus particles. Segment 5 codes for the nucleocapsid protein (NP) that binds to viral RNA and, together with the polymerase proteins, forms the ribonucleoprotein complexes (RNPs). Segment 7 codes for the viral matrix structural protein M1 and the ion-channel protein M2 that is incorporated in the viral membrane. Segment 8 encodes the nonstructural protein NS1 and the nucleic-export protein (NEP) previously known as NS2. NS1 is an antagonist of host innate immune responses and interferes with host gene expression, whereas NEP is involved in the nuclear export of RNPs into the cytoplasm before virus assembly (2, 3).
Influenza Aviruses show pronounced genetic variation of the surface glycoproteins HA and NA (1). Consequently, the viruses are classified based on the antigenic variation of the HA and NA proteins. To date, 16 major antigenic variants of HA and 9 of NA have been recognized in wild birds and are found in numerous combinations designated as virus subtypes (for instance, H1N1, H5N1, H7N7, and H16N3), which are used in influenza Avirus classification and nomenclature (1, 6). This classification system is biologically relevant, as natural host antibodies that recognize one HA or NA subtype will generally not cross-react with other HA and NA subtypes.
On the basis of their virulence in chickens, influenza A viruses of the H5 and H7 subtypes can be further classified into highly pathogenic avian influenza (HPAI) and low-pathogenic avian influenza (LPAI) viruses. Viruses of subtypes H1 to H4, H6, and H8 to H16 are LPAI viruses. The vast majority of H5 and H7 influenza A viruses are also of the LPAI phenotype. HPAI viruses are generally thought to arise in poultry after domestic birds become infected by LPAI H5 and H7 viruses from the wild-bird reservoir (7, 8). The HA protein of influenza A viruses is initially synthesized as a single polypeptide precursor (HA0), which is cleaved into HA1 and HA2 subunits by trypsin-like proteases in the host cell. The switch from LPAI to HPAI virus phenotype occurs upon the introduction of basic amino acid residues into the HA0 cleavage site, also known as the multibasic cleavage site (MBCS). The MBCS in HA can be cleaved by ubiquitously expressed host proteases; this cleavage facilitates systemic virus replication and results in mortality of up to 100% in poultry (9, 10).
Since the late 1990s, HPAI A/H5N1 viruses have devastated the poultry industry of numerous countries in the Eastern Hemisphere. To date, A/H5N1 has spread from Asia to Europe, Africa, and the Middle East, resulting in the death of hundreds of millions of domestic birds. In Hong Kong in 1997, the first human deaths directly attributable to avian A/H5N1 virus were recorded (11). Since 2003, more than 600 laboratory-confirmed cases of HPAI A/H5N1 virus infections in humans have been reported from 15 countries (12). Although limited A/H5N1 virus transmission between persons in close contact has been reported, sustained human-to-human transmission of HPAI A/H5N1 virus has not been detected (13–15). Whether this virus may acquire the ability to be transmitted via aerosols or respiratory droplets among mammals, including humans, to trigger a future pandemic is a key question for pandemic preparedness. Although our knowledge of viral traits necessary for host switching and virulence has increased substantially in recent years (16, 17), the factors that determine airborne transmission of influenza viruses among mammals, a trait necessary for a virus to become pandemic, have remained largely unknown (18–21). Therefore, investigations of routes of influenza virus transmission between animals and on the determinants of airborne transmission are high on the influenza research agenda.
The viruses that caused the major pandemics of the past century emerged upon reassortment (that is, genetic mixing) of animal and human influenza viruses (22). However, given that viruses from only four pandemics are available for analyses, we cannot exclude the possibility that a future pandemic may be triggered by a wholly avian virus without the requirement of reassortment. Several studies have shown that reassortment events between A/H5N1 and seasonal human influenza viruses do not yield viruses that are readily transmitted between ferrets (18–20, 23). In our work, we investigated whether A/H5N1 virus could change its transmissibility characteristics without any requirement for reassortment.
We chose influenza virus A/Indonesia/5/2005 for our study because the incidence of human A/H5N1 virus infections and fatalities in Indonesia remains fairly high (12), and there are concerns that this virus could acquire molecular characteristics that would allow it to become more readily transmissible between humans and initiate a pandemic. Because no reassortants between A/H5N1 viruses and seasonal or pandemic human influenza viruses have been detected in nature and because our goal was to understand the biological properties needed for an influenza virus to become airborne transmissible in mammals, we decided to use the complete A/Indonesia/5/2005 virus that was isolated from a human case of HPAI A/H5N1 infection.
We chose the ferret (Mustela putorius furo) as the animal model for our studies. Ferrets have been used in influenza research since 1933 because they are susceptible to infection with human and avian influenza viruses (24). After infection with human influenza A virus, ferrets develop respiratory disease and lung pathology similar to that observed in humans. Ferrets can also transmit human influenza viruses to other ferrets that serve as sentinels with or without direct contact (fig. S1) (25–27).
Host restriction of replication and transmission of influenza A viruses is partly determined by specific SA receptors on the surface of susceptible cells. The affinity of influenza viruses for these receptors varies according to the species from which they are isolated. Influenza viruses of avian origin preferentially bind to α-2,3–linked SA receptors, whereas human influenza viruses recognize α-2,6–linked SA receptors. The receptor distribution in ferrets resembles that of humans in that the α-2,6–linked SA receptors are predominantly present in the upper respiratory tract (URT), and the α-2,3–linked SA receptors are mainly present in the lower respiratory tract. In chickens and other birds, α-2,3–linked SAs predominate, but both α-2,3–linked and α-2,6–linked SA are present throughout the respiratory and enteric tracts (fig. S2) (28). The differences in receptor distribution between humans and avian species are thought to determine the host restriction of influenza A viruses. A switch in receptor specificity from avian α-2,3–SA to human α-2,6–SA receptors, which can be acquired by specific mutations in the receptor binding site (RBS) of the HA, is expected to be necessary for an avian virus to become transmissible and, thus, gain the potential to become pandemic in humans.
Besides a switch in receptor specificity to facilitate infection of cells in the URT, increased virus production in the URT and efficient release of virus particles from the respiratory tract to yield airborne virus may also be required (22). Such traits are likely to be determined by the viral surface glycoproteins and the proteins that form the viral polymerase complex. Amino acid substitutions in the polymerase proteins have already been shown to be major determinants of host range and transmission, including for pandemic influenza viruses (29–31). Whereas avian viruses, in principle, replicate at temperatures around 41°C (the temperature in the intestinal tract of birds), for replication in humans the viruses need to adapt to 33°C (the temperature of the human URT). The amino acid substitution Glu627→Lys627 (E627K) in the polymerase complex protein PB2 has been associated with increased virus replication in mammalian cells at such lower temperatures (16, 17, 32).
In addition, when newly formed virus particles bud from the host cell membrane after virus replication, the NA present on the virus membrane facilitates the release of particles. For A/H5N1, this process is rather inefficient, and released particles tend to form virus aggregates (22). Therefore, a balance between the properties endowed by HA and NA may be required to generate single particles. These established effects were thus used as the basis for the initial substitutions chosen in the current study.
Human-to-human transmission of influenza viruses can occur through direct contact, indirect contact via fomites (contaminated environmental surfaces), and/or airborne transmission via small aerosols or large respiratory droplets. The pandemic and epidemic influenza viruses that have circulated in humans throughout the past century were all transmitted via the airborne route, in contrast to many other respiratory viruses that are exclusively transmitted via contact. There is no exact particle size cut-off at which transmission changes from exclusively large droplets to aerosols. However, it is generally accepted that for infectious particles with a diameter of 5 μm or less, transmission occurs via aerosols. Because we did not measure particle size during our experiments, we will use the term “airborne transmission” throughout this Report.
Biosafety and biosecurity concerns have remained foremost in our planning for this research program. The details are explained in the supplementary materials and are summarized here: The enhanced Animal Biosafety Laboratory level 3 (ABSL3+) facility at Erasmus Medical Center (MC) Rotterdam, the Netherlands, was constructed for the specific purpose of containing pathogenic and transmissible influenza viruses and other pathogens of concern. The facility consists of a negatively pressurized laboratory with an interlock room. All in vivo and in vitro experimental work is carried out in negatively pressurized class 3 isolators or class 3 biosafety cabinets, respectively. The facility is secured by procedures recognized as appropriate by the institutional biosafety officers and facility management at Erasmus MC, as well as Dutch and U.S. government inspectors.
Before and during the research, biosafety officers of Erasmus MC and inspectors from the Dutch government, as well as from the U.S. Centers for Disease Control and Prevention, approved the facilities and procedures. Explicit permits for research on genetically modified airborne-transmissible A/H5N1 virus were obtained from the Dutch government. The research was performed strictly in accordance with the Dutch Code of Conduct for Biosecurity (33). All personnel were instructed and trained extensively for working in the ABSL3+ facility, handling (highly pathogenic) influenza virus, and controlling incidents (such as spills). To further prevent occupational risks, research personnel used protective equipment and were offered seasonal and A/H5N1 influenza vaccines (25). For emergency purposes, Erasmus MC holds supplies of oseltamivir and has quarantine hospital rooms.
Using a combination of targeted mutagenesis followed by serial virus passage in ferrets, we investigated whether A/H5N1 virus can acquire mutations that would increase the risk of mammalian transmission (34). We have previously shown that several amino acid substitutions in the RBS of the HA surface glycoprotein of A/Indonesia/5/2005 change the binding preference from the avian α-2,3–linked SA receptors to the human α-2,6–linked SA receptors (35). A/Indonesia/5/2005 virus with amino acid substitutions N182K, Q222L/G224S, or N182K/Q222L/G224S (numbers refer to amino acid positions in the mature H5 HA protein; N, Asn; Q, Gln; L, Leu; G, Gly; S, Ser) in HA display attachment patterns similar to those of human viruses to cells of the respiratory tract of ferrets and humans (35). Of these changes, we know that together, Q222L and G224S switch the receptor binding specificity of H2 and H3 subtype influenza viruses, as this switch contributed to the emergence of the 1957 and 1968 pandemics (36). N182K has been found in a human case of A/H5N1 virus infection (37).
Our experimental rationale to obtain transmissible A/H5N1 viruses was to select a mutant A/H5N1 virus with receptor specificity for α-2,6–linked SA shed at high titers from the URT of ferrets. Therefore, we used the QuickChange multisite-directed mutagenesis kit (Agilent Technologies, Amstelveen, the Netherlands) to introduce amino acid substitutions N182K, Q222L/G224S, or N182K/Q222L/G224S in the HA of wild-type (WT) A/Indonesia/5/2005, resulting in A/H5N1HA N182K, A/H5N1HA Q222L,G224S, and A/H5N1HA N182K,Q222L,G224S. Experimental details for experiments 1 to 9 are provided in the supplementary materials (25). For experiment 1, we inoculated these mutant viruses and the A/H5N1wildtype virus intranasally into groups of six ferrets for each virus (fig. S3). Throat and nasal swabs were collected daily, and virus titers were determined by end-point dilution in Madin Darby canine kidney (MDCK) cells to quantify virus shedding from the ferret URT. Three animals were euthanized after day 3 to enable tissue sample collection. All remaining animals were euthanized by day 7 when the same tissue samples were taken. Virus titers were determined in the nasal turbinates, trachea, and lungs collected postmortem from the euthanized ferrets. Throughout the duration of experiment 1, ferrets inoculated intranasally with A/H5N1wildtype virus produced high titers in nose and throat swabs—up to 10 times more than A/H5N1HA Q222L,G224S, which yielded the highest virus titers of all three mutants during the 7-day period (Fig. 1). However, no significant difference was observed between the virus shedding of ferrets inoculated with A/H5N1HA Q222L, G224S or A/H5N1HA N182K during the first 3 days when six animals per group were present. Thus, of the viruses with specificity for α-2,6–linked SA, A/H5N1HA Q222L,G224S yielded the highest virus titers in the ferret URT (Fig. 1).
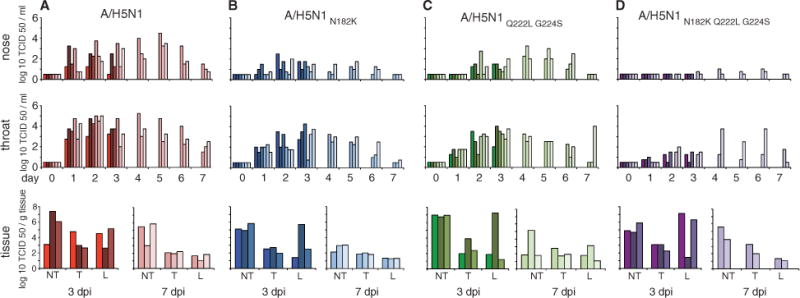
In experiment 1, we inoculated groups of six ferrets intranasally with 1 × 106 TCID50 of (A) influenza A/H5N1wildtype virus and the three mutants (B) A/H5N1HA N182K, (C) A/H5N1HA Q222L,G224S, and (D) A/H5N1HA N182K,Q222L,G224S. Three animals were euthanized at day 3 for tissue sampling and at day 7, when this experiment was stopped. Virus titers were measured daily in nose swabs (top) and throat swabs (middle) and also on 3 and 7 dpi in respiratory tract tissues (bottom) of individual ferrets. Virus titers in swabs and nasal turbinates (NT), trachea (T), and lungs (L) were determined by end-point titration in MDCK cells. [One animal inoculated with A/H5N1HA N182K,Q222L,G224S died at 1 dpi due to circumstances not related to the experiment (D).] (Top two rows) Virus shedding from the URT as determined by virus titers in nasal and throat swabs was highest in A/H5N1wildtype-inoculated animals. The mutant that yielded the highest virus titers during the 7-day period was A/H5N1HA Q222L,G224S, but titers were ~1 log lower than for the A/H5N1wildtype-inoculated animals. In the first 3 days, when six animals per group were present, no significant differences were observed between A/H5N1HA N182K- and A/H5N1HA Q222L,G224S-inoculated animals, as calculated by comparing the viral titer (Mann-Whitney test, P = 0.589 and 0.818 for nose and throat titers, respectively). (Bottom row) No marked differences in virus titers in respiratory tissues were observed between the four groups. Each bar color denotes a single animal.
As described above, amino acid substitution E627K in PB2 is one of the most consistent host-range determinants of influenza viruses (29–31). For experiment 2 (fig. S4), we introduced E627K into the PB2 gene of A/Indonesia/5/2005 by site-directed mutagenesis and produced the recombinant virus A/H5N1HA Q222L,G224S PB2 E627K. The introduction of E627K in PB2 did not significantly affect virus shedding in ferrets, because virus titers in the URT were similar to those seen in A/H5N1HA Q222L,G224S-inoculated animals [up to 1 × 104 50% tissue culture infectious doses (TCID50)] (Mann-Whitney U rank-sum test, P = 0.476) (Fig. 1 and fig. S5). When four naïve ferrets were housed in cages adjacent to those with four inoculated animals to test for airborne transmission as described previously (27), A/H5N1HA Q222L,G224S PB2 E627K was not transmitted (fig. S5).
Because the mutant virus harboring the E627K mutation in PB2 and Q222L and G224S in HA did not transmit in experiment 2, we designed an experiment to force the virus to adapt to replication in the mammalian respiratory tract and to select virus variants by repeated passage (10 passages in total) of the constructed A/H5N1HA Q222L,G224S PB2 E627K virus and A/H5N1wildtype virus in the ferret URT (Fig. 2 and fig. S6). In experiment 3, one ferret was inoculated intranasally with A/H5N1wildtype and one ferret with A/H5N1HA Q222L,G224S PB2 E627K. Throat and nose swabs were collected daily from live animals until 4 days postinoculation (dpi), at which time the animals were euthanized to collect samples from nasal turbinates and lungs. The nasal turbinates were homogenized in 3 ml of virus-transport medium, tissue debris was pelleted by centrifugation, and 0.5 ml of the supernatant was subsequently used to inoculate the next ferret intranasally (passage 2). This procedure was repeated until passage 6.
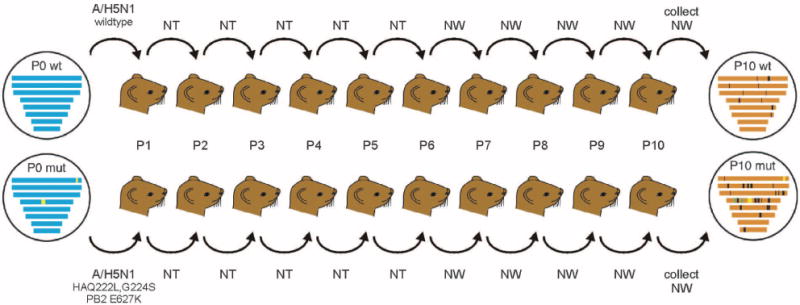
Experiment 3, virus passaging in ferrets (P1 to P10, passages 1 to 10). Because no airborne transmission was observed in experiment 2, A/H5N1wildtype and A/H5N1HA Q222L,G224S PB2 E627K were serially passaged in ferrets to allow adaptation for efficient replication in mammals. Each virus was inoculated intranasally with 1 × 106 TCID50 in one ferret (2 × 250 μl, divided over both nostrils). Nose and throat swabs were collected daily. Animals were euthanized at 4 dpi, and nasal turbinates and lungs were collected. Nasal turbinates were homogenized in virus-transport medium, and this homogenate was used to inoculate the next ferret, resulting in passage 2 (fig. S6). Subsequent passages 3 to 6 were performed in the same way. From passage six onward, nasal washes (NW) were collected at 3 dpi in addition to the nasal swabs. To this end, 1 ml of PBS was delivered drop wise into the nostrils of the ferrets, thereby inducing sneezing. Approximately 200 μl of the sneeze was collected in a Petri dish, and PBS was added to a final volume of 2 ml. For passages 7 through 10, the nasal-wash sample was used for the passages in ferrets. The passage-10 nasal washes were subsequently used for sequence analyses and transmission experiments to be described in experiment 4. For details, see the supplementary materials.
From passage 6 onward, in addition to the samples described above, a nasal wash was also collected at 3 dpi. To this end, 1 ml of phosphate-buffered saline (PBS) was delivered dropwise to the nostrils of the ferrets to induce sneezing. Approximately 200 μl of the “sneeze” was collected in a Petri dish, and PBS was added to a final volume of 2 ml. The nasal-wash samples were used for intranasal inoculation of the ferrets for the subsequent passages 7 through 10. We changed the source of inoculum during the course of the experiment, because passaging nasal washes may facilitate the selection of viruses that were secreted from the URT. Because influenza viruses mutate rapidly, we anticipated that 10 passages would be sufficient for the virus to adapt to efficient replication in mammals.
Virus titers in the nasal turbinates of ferrets inoculated with A/H5N1wildtype ranged from ~1 × 105 to 1 × 107 TCID50/gram tissue throughout 10 serial passages (Fig. 3A and fig. S7). In ferrets inoculated with A/H5N1HA Q222L,G224S PB2 E627K virus, a moderate increase in virus titers in the nasal turbinates was observed as the passage number increased. These titers ranged from 1 × 104 TCID50/gram tissue at the start of the experiment to 3.2 × 105 to 1 × 106 TCID50/gram tissue in the final passages (Fig. 3A and fig. S7). Notably, virus titers in the nose swabs of animals inoculated with A/H5N1HA Q222L,G224S PB2 E627K also increased during the successive passages, with peak virus shedding of 1 × 105 TCID50 at 2 dpi after 10 passages (Fig. 3B). These data indicate that A/H5N1HA Q222L,G224S PB2 E627K was developing greater capacity to replicate in the ferret URT after repeated passage, with evidence for such adaptation becoming apparent by passage number 4. In contrast, virus titers in the nose swabs of the ferrets collected at 1 to 4 dpi throughout 10 serial passages with A/H5N1wildtype revealed no changes in patterns of virus shedding.
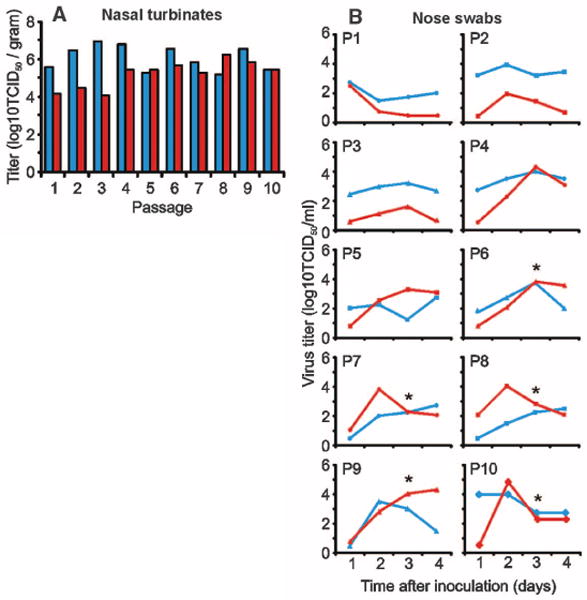
Virus titers in (A) the nasal turbinates collected at day 4 and (B) nose swabs collected daily until day 4, from ferrets inoculated with A/H5N1wildtype (blue) and A/H5N1HAQ222L,G224S PB2 E627K (red) throughout the 10 serial passages described in Fig. 2. Virus titers were determined by end-point titration in MDCK cells. After inoculation with A/H5N1wildtype, virus titers in the nasal turbinates were variable but high, ranging from 1.6 × 105 to 7.9 × 106 TCID50/gram tissue (A), with no further increase observed with repeated passage. After inoculation with A/H5N1HA Q222L,G224S PB2 E627K, virus titers in nasal turbinates averaged 1.6 × 104 in the first three passages, 2.5 × 105 in passages four to seven, and 6.3 × 105 TCID50/gram tissue in the last three passages, suggestive of improved replication and virus adaptation. A similar pattern of adaptation was observed in the virus titers in the nose swabs of animals inoculated with A/H5N1HA Q222L,G224S PB2 E627K (B). These titers also increased during the successive passages, with peak virus shedding of 1 × 105 TCID50 at 2 dpi after 10 passages. Altogether, these data indicate that A/H5N1HA Q222L,G224S PB2 E627K adapted to more efficient replication in the ferret URT upon repeated passage, with evidence for such adaptation by passage number 4. In contrast, analyses of the virus titers in the nose swabs of the ferrets collected at 1 to 4 dpi throughout the 10 serial passages with A/H5N1wildtype revealed no changes in patterns of virus shedding. Asterisks indicate that a nose wash was collected before the nose swab was taken, which may influence the virus titer that was detected.
Passaging of influenza viruses in ferrets should result in the natural selection of heterogeneous mixtures of viruses in each animal with a variety of mutations: so-called viral quasispecies (38). The genetic composition of the viral quasi-species present in the nasal washes of ferrets after 10 passages of A/H5N1wildtype and A/H5N1HA Q222L,G224S PB2 E627K was determined by sequence analysis using the 454/Roche GS-FLX sequencing platform (Roche, Woerden, the Netherlands) (tables S1 and S2). The mutations introduced in A/H5N1HA Q222L,G224S PB2 E627K by reverse genetics remained present in the virus population after 10 consecutive passages at a frequency >99.5% (Fig. 4 and table S1). Numerous additional nucleotide substitutions were detected in all viral gene segments of A/H5N1wildtype and A/H5N1HA Q222L,G224S PB2 E627K after passaging, except in segment 7 (tables S1 and S2). Of the 30 nucleotide substitutions selected during serial passage, 53% resulted in amino acid substitutions. The only amino acid substitution detected upon repeated passage of both A/H5N1wildtype and A/H5N1HA Q222L,G224S PB2 E627K was T156A (T, Thr; A, Ala) in HA. This substitution removes a potential N-linked glycosylation site (Asn-X-Thr/Ser; X, any amino acid) in HA and was detected in 99.6% of the A/H5N1wildtype sequences after 10 passages. T156Awas detected in 89% of the A/H5N1HA Q222L,G224S PB2 E627K sequences after 10 passages, and the other 11% of sequences possessed the substitution N154K, which removes the same potential N-linked glycosylation site in HA.
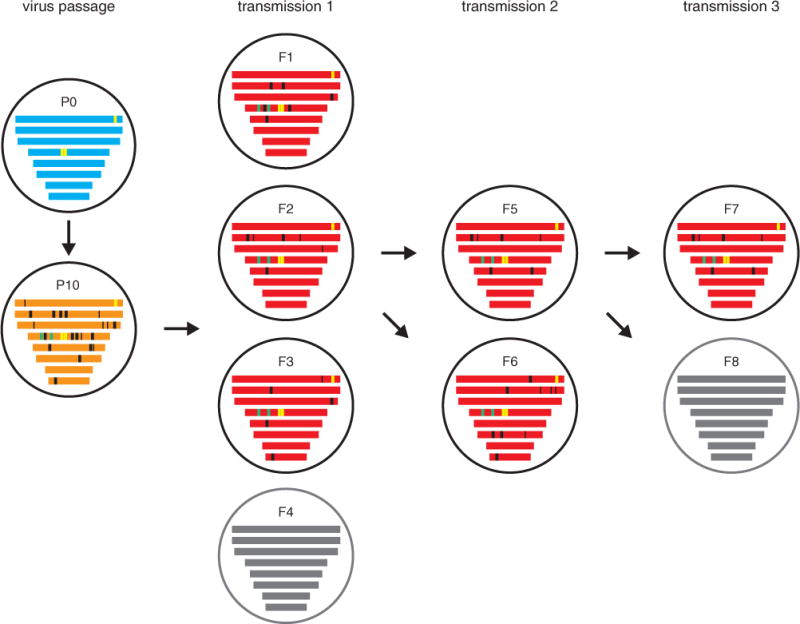
Summary of the substitutions detected upon serial passage and airborne transmission of A/H5N1HA Q222L,G224S PB2 E627K virus in ferrets. The eight influenza virus gene segments and substitutions are drawn approximately to scale (top to bottom: PB2, PB1, PA, HA, NP, NA, M, NS). Viruses shown in blue, orange, and red represent the initial recombinant A/H5N1HA Q222L,G224S PB2 E627K virus (P0), ferret passage-10 virus (P10), and P10 virus after airborne transmission to recipient ferrets, respectively. Viruses shown in gray indicate that virus was not transmitted to the recipient ferret. First, we tested whether airborne-transmissible viruses were present in the heterogeneous virus population of ferret P10. We inoculated four donor ferrets intranasally, which were then housed in transmission cages and paired with four recipient ferrets. Transmissible viruses were isolated from three out of four recipient ferrets (F1 to F3). Next, we took a throat-swab sample from F2 (this sample contained the highest virus titer among the positive recipient ferrets), and this sample was used to inoculate two more donor ferrets intranasally. In a transmission experiment, these donors infected two recipient ferrets via airborne transmission (F5 and F6). Virus isolated from F5 was passaged once in MDCK cells and was subsequently used in a third transmission experiment in which two intranasally inoculated donor ferrets transmitted the virus to one of two recipient ferrets (F7). The genetic composition of the viral quasi-species present in the nasal wash of ferret P10 was determined by sequence analysis using the 454/Roche GS-FLX sequencing platform. Conventional Sanger sequencing was used to determine the consensus sequence in one high-titer nasal- or throat-swab sample for each ferret. Thick and thin black vertical bars indicate amino acid and nucleotide substitutions, respectively; substitutions introduced by reverse genetics are shown in yellow; substitutions detected in passage 10 and all subsequent transmissions are shown in green.
In experiment 4 (see supplementary materials), we investigated whether airborne-transmissible viruses were present in the heterogeneous virus population generated during virus passaging in ferrets (fig. S4). Nasal-wash samples, collected at 3 dpi from ferrets at passage 10, were used in transmission experiments to test whether airborne-transmissible virus was present in the virus quasi-species. For this purpose, nasal-wash samples were diluted 1:2 in PBS and subsequently used to inoculate six naïve ferrets intranasally: two for passage 10 A/H5N1wildtype and four for passage 10 A/H5N1HA Q222L,G224S PB2 E627K virus.
The following day, a naïve recipient ferret was placed in a cage adjacent to each inoculated donor ferret. These cages are designed to prevent direct contact between animals but allow airflow from a donor ferret to a neighboring recipient ferret (fig. S1) (27). Although mutations had accumulated in the viral genome after passaging of A/H5N1wildtype in ferrets, we did not detect replicating virus upon inoculation of MDCK cells with swabs collected from naïve recipient ferrets after they were paired with donor ferrets inoculated with passage 10 A/H5N1wildtype virus (Fig. 5, A and B). In contrast, we did detect virus in recipient ferrets paired with those inoculated with passage 10 A/H5N1HA Q222L,G224S PB2 E627K virus. Three (F1 to F3) out of four (F1 to F4) naïve recipient ferrets became infected as confirmed by the presence of replicating virus in the collected nasal and throat swabs (Fig. 5, C and D). A throat-swab sample obtained from recipient ferret F2, which contained the highest virus titer among the ferrets in the first transmission experiment, was subsequently used for intranasal inoculation of two additional donor ferrets. Both of these animals, when placed in the transmission cage setup (fig. S1), again transmitted the virus to the recipient ferrets (F5 and F6) (Fig. 6, A and B). A virus isolate was obtained after inoculation of MDCK cells with a nose swab collected from ferret F5 at 7 dpi. The virus from F5 was inoculated intranasally into two more donor ferrets. One day later, these animals were paired with two recipient ferrets (F7 and F8) in transmission cages, one of which (F7) subsequently became infected (Fig. 6, C and D).
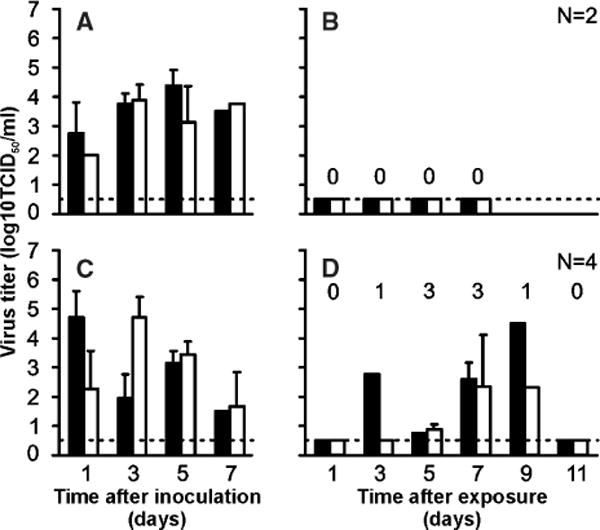
Airborne transmission of A/H5N1 viruses in ferrets. Transmission experiments are shown for A/H5N1wildtype (A and B) and A/H5N1HA Q222L,G224S PB2 E627K (C and D) after 10 passages (P10) in ferrets. Two or four ferrets were inoculated intranasally with nasal-wash samples collected from P10 virus of A/H5N1wildtype and A/H5N1HA Q222L,G224S PB2 E627K, respectively, and housed individually in transmission cages (A and C). A naïve recipient ferret was added to each transmission cage adjacent to a donor ferret at 1dpi (B and D). Virus titers in throat (black bars) and nose swabs (white bars) were determined by end-point titration in MDCK cells. Geometric mean titers and SDs (error bars) of positive samples are shown. The number of animals infected via airborne transmission is indicated in (D) for each time point after exposure; the drop from three animals infected at day 7 to one animal at day 9 and no animals at day 11 is explained by the fact that the animals that became infected via airborne transmission had cleared the virus by the end of the experiment and, therefore, detectable amounts of virus were no longer present. The dotted lines indicate the lower limit of virus detection.
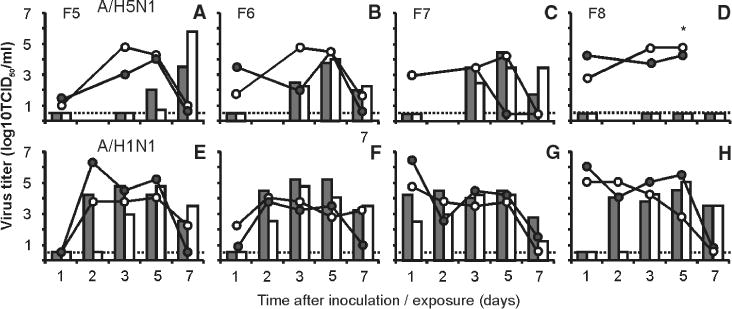
Comparison of airborne transmission of experimental passaged A/H5N1 and 2009 pandemic A/H1N1 viruses in individual ferrets. A throat-swab sample from ferret F2 at 7 days postexposure (dpe) (Fig. 5D) was used for the transmission experiments shown in (A) and (B), and a virus isolate obtained from a nose swab collected from ferret F5 at 7 dpi (Fig. 6A) was used for the experiments in (C) and (D). For comparison, published data on transmission of 2009 pandemic A/H1N1 virus between ferrets is shown in (E) to (H) (27). Data for individual transmission experiments is shown in each panel, with virus shedding in inoculated and airborne virus–exposed animals shown as lines and bars, respectively. For the transmission experiments with airborne-transmissible A/H5N1 (A to D), nose or throat swabs were not collected at 2 dpi and 2 dpe. White circles and bars represent shedding from the nose; black circles and bars represent shedding from the throat. The asterisk indicates the inoculated animal that died 6 days after intranasal inoculation.
We used conventional Sanger sequencing to determine the consensus genome sequences of viruses recovered from the six ferrets (F1 to F3 and F5 to F7) that acquired virus via airborne transmission (Fig. 4 and table S3). All six samples still harbored substitutions Q222L, G224S, and E627K that had been introduced by reverse genetics. Surprisingly, only two additional amino acid substitutions, both in HA, were consistently detected in all six airborne-transmissible viruses: (i) H103Y (H, His; Y, Tyr), which forms part of the HA trimer interface, and (ii) T156A, which is proximal but not immediately adjacent to the RBS (fig. S8). Although we observed several other mutations, their occurrence was not consistent among the airborne viruses, indicating that of the heterogeneous virus populations generated by passaging in ferrets, viruses with different genotypes were transmissible. In addition, a single transmission experiment is not sufficient to select for clonal airborne-transmissible viruses because, for example, the consensus sequence of virus isolated from F6 differed from the sequence of parental virus isolated from F2.
Together, these results suggest that as few as five amino acid substitutions (four in HA and one in PB2) may be sufficient to confer airborne transmission of HPAI A/H5N1 virus between mammals. The airborne-transmissible virus isolate with the least number of amino acid substitutions, compared with the A/H5N1wildtype, was recovered from ferret F5. This virus isolate had a total of nine amino acid substitutions; in addition to the three mutations that we introduced (Q222L and G224S in HA and E627K in PB2), this virus harbored H103Y and T156A in HA, H99Y and I368V (I, Ile; V, Val) in PB1, and R99K (R, Arg) and S345N in NP (table S3). Reverse genetics will be needed to identify which of the five to nine amino acid substitutions in this virus are essential to confer airborne transmission.
During the course of the transmission experiments with the airborne-transmissible viruses, ferrets displayed lethargy, loss of appetite, and ruffled fur after intranasal inoculation. One of eight inoculated animals died upon intranasal inoculation (Table 1). In previously published experiments, ferrets inoculated intranasally with WTA/Indonesia/5/2005 virus at a dose of 1 × 106 TCID50 showed neurological disease and/or death (39, 40). It should be noted that inoculation of immunologically naïve ferrets with a dose of 1 × 106 TCID50 of A/H5N1 virus and the subsequent course of disease is not representative of the natural situation in humans. Importantly, although the six ferrets that became infected via respiratory droplets or aerosol also displayed lethargy, loss of appetite, and ruffled fur, none of these animals died within the course of the experiment. Moreover, previous infections of humans with seasonal influenza viruses are likely to induce heterosubtypic immunity that would offer some protection against the development of severe disease (41, 42). It has been shown that mice and ferrets previously infected with an A/H3N2 virus are clinically protected against intranasal challenge infection with an A/H5N1 virus (43, 44).
Table 1
Lethality of WT and airborne-transmissible A/H5N1 virus in ferrets upon inoculation via different routes. n, number of animals; N.A., not applicable.
Inoculation route | Virus | Dead or moribund (no. dead/no. tested) | Day of death postinoculation (no.) |
---|---|---|---|
Intratracheal | A/H5N1wildtype | 6/6* | 2 (n = 2), 3 (n = 4) |
A/H5N1/F5 | 6/6 | 3 (n = 6) | |
Intranasal | A/H5N1wildtype/P10 | 2/2† | 6 (n = 2) |
A/H5N1HA Q222L,G224S PB2 E627K/P10 | 0/4 | N.A. | |
A/H5N1/F2 | 0/2 | N.A. | |
A/H5N1/F5 | 1/2 | 6 (n = 1) | |
Airborne | A/H5N1wildtype | N.A. | N.A. |
A/H5N1HA Q222L,G224S PB2 E627K/P10 | 0/3 | N.A. | |
A/H5N1/F2 | 0/2 | N.A. | |
A/H5N1/F5 | 0/1 | N.A. |
After intratracheal inoculation (experiment 5; fig. S9), six ferrets inoculated with 1 × 106 TCID50 of airborne-transmissible virus F5 in a 3-ml volume of PBS died or were moribund at day 3. Intratracheal inoculations at such high doses do not represent the natural route of infection and are generally used only to test the ability of viruses to cause pneumonia (45), as is done for vaccination-challenge studies. At necropsy, the six ferrets revealed macroscopic lesions affecting 80 to 100% of the lung parenchyma with average virus titers of 7.9 × 106 TCID50/gram lung (fig. S10). These data are similar to those described previously for A/H5N1wildtype in ferrets (Table 1). Thus, although the airborne-transmissible virus is lethal to ferrets upon intratracheal inoculation at high doses, the virus was not lethal after airborne transmission.
To test the effect of the mutations in HA in the airborne-transmissible virus on its sensitivity to antiviral drugs, we used virus isolated from F5 (experiment 6). This airborne-transmissible virus with nine amino acid substitutions displayed a sensitivity to the antiviral drug oseltamivir similar to that of A/H5N1wildtype (table S4).
In experiment 7, we evaluated the recognition of the airborne-transmissible virus by antisera raised against potential A/H5N1 vaccine strains. Because only HA recognition by antibodies is evaluated in this assay, chimeric viruses were generated based on six gene segments of the mouse-adapted A/Puerto Rico/8/34 (PR8) virus with the HA and PB2 genes of the transmissible virus harboring amino acid substitutions H103Y, T156A, Q222L, and G224S in HA and E627K in PB2. We replaced the MBCS of the HA by a monobasic cleavage site, allowing us to do these experiments under BSL2 conditions. The chimeric PR8/H5 virus reacted well with ferret antisera raised against A/Indonesia/5/2005 and several other prepandemic vaccine strains (table S5). In fact, the presence of the four HA mutations increased the reactivity with H5 antisera by twofold or more.
We subsequently used the same PR8/H5 chimeric virus in experiment 8 to evaluate the presence of existing immunity against the airborne-transmissible virus in sera obtained from human volunteers more than 70 years of age. The introduction of receptor-binding site mutations Q222L/G224S and the mutations H103Y and T156A in HA, acquired during ferret passage, did not result in increased cross-reactivity with human antisera (table S6), indicating that humans do not have antibodies against the HA of the airborne-transmissible A/H5N1 virus that was selected in our experiments.
Substitutions Q222L and G224S have previously been shown to be sufficient to switch receptor-binding specificity of avian influenza strains (i.e., α-2,3–linked SA) to that of human strains (i.e., α-2,6–linked SA) (20, 35, 46, 47). Amino acid position 103 is distal from the RBS, forms part of the trimer interface, and is unlikely to affect receptor specificity (fig. S8). T156 is part of a N-glycosylation sequon, and T156A (as well as N154K) would delete this potential glycosylation site (fig. S8); amino acid T156 is proximal but not immediately adjacent to the RBS. Loss of N-glycosylation sites at the tip of HA has been shown to affect receptor binding of A/H1 (48, 49) and the virulence of A/H5 virus (50). We evaluated the impact of the HA mutations that emerged during passaging in ferrets in a modified turkey red blood cell (TRBC) assay (Table 2). In this assay, the binding of influenza viruses, with a mutated HA, to normal TRBCs (expressing both α-2,3–linked SA and α-2,6–linked SA) and modified TRBCs with either α-2,3–linked SA or α-2,6–linked SA on the cell surface was evaluated and compared to two reference viruses with known receptor binding preference: avian A/H5N1 and human A/H3N2 viruses. As expected and shown before, introduction of the Q222L and G224S mutations in the HA of A/H5N1 changed the receptor binding preference from α-2,3–linked SA to α-2,6–linked SA (35). Furthermore, in our hands, the introduction of substitutions H103Yand T156A not only enhanced binding of A/H5N1HA Q222L,G224S PB2 E627K to α-2,6–linked SA, as expected from glycan array studies (51), but also increased the affinity for α-2,3–linked SA. When these two mutations were introduced in the A/H5N1wildtype HA, the affinity for α-2,3–linked SA also increased.
Table 2
Receptor specificity of the different mutant A/H5N1 viruses, as determined by a modified TRBC hemagglutination assay. Introduction of Q222L and G224S in the A/H5N1 HA resulted in a receptor binding preference switch from the avian α-2,3– to the human α-2,6–linked SA receptor. Subsequent substitution of H103Y and T156A resulted in an increased affinity for α-2,3– and α-2,6–linked SA, in agreement with glycan array studies (51). For details, see supplementary experiment 9. HAU, hemagglutination units.
Virus | Subtype | HA titer (HAU/50 μl)
| ||
---|---|---|---|---|
TRBC | α-2,3–linked TRBC | α-2,6–linked TRBC | ||
A/Netherlands/213/03 | H3N2 | 64 | 0 | 64 |
A/Vietnam/1194/04 | H5N1 | 64 | 64 | 0 |
A/H5N1PB2 E627K | H5N1 | 64 | 16 | 0 |
A/H5N1HA H103Y,T156A PB2 E627K | H5N1 | 64 | 48 | 0 |
A/H5N1HA Q222L,G224S PB2 E627K | H5N1 | 64 | 0 | 24 |
A/H5N1HA H103Y,T156A,Q222L,G224S PB2 E627K | H5N1 | 64 | 4 | 32 |
Substitutions Q222L and G224S have previously emerged in avian A/H2 and A/H3 viruses in nature (36, 52), and mutations associated with similar changes in receptor binding specificity have been detected repeatedly in A/H5 viruses—for instance, substitution N182K has been reported nine times (37, 51), which is why we initially selected it for our investigations. The other three substitutions we found consistently in airborne-transmissible viruses have all previously been detected in HPAI A/H5N1 viruses circulating in the field (53). Only a minor fraction of the A/H5N1 viruses that have circulated in outbreaks have been sequenced (estimated to be <0.001%) (53, 54). Yet the individual substitutions we obtained, as well as combinations of T156A and H103Yor T156A and E627K, have already been reported in public sequence databases (53); thus, we conclude that these mutations do not appear to have a detrimental effect on virus fitness. Substitution H103Y has only been found once, in combination with T156A in a duck in China (53). Substitution E627K in PB2 has been found in ~27% of avian A/H5N1 virus sequences and in ~29% of human A/H5N1 viruses (53). Substitution T156A in HA has been reported in >50% of the viruses sequenced and was detected in 100% of the viruses from human cases in Egypt (53).
Investigations of viral quasi-species during a massive avian influenza A/H7N7 virus outbreak in the Netherlands indicated that viruses with human adaptation markers, including HA mutations that alter receptor specificity and mutations in polymerase proteins that increase polymerase activity like E627K in PB2, emerged rapidly in poultry (55–57). Given the large numbers of HPAI A/H5N1 virus-infected hosts globally, the high viral mutation rate, and the apparent lack of detrimental effects on fitness of the mutations that confer airborne transmission, it may simply be a matter of chance and time before a human-to-human transmissible A/H5N1 virus emerges.
The specific mutations we identified in these experiments that are associated with airborne transmission represent biological traits that may be determined by a set of different amino acid substitutions. For example, amino acid substitutions D701N (D, Asp) or S590G/R591Q in PB2 yield a similar phenotype to E627K (29). N182K and other substitutions in the RBS of HA may yield a similar phenotype to Q222L/G224S (35). Such mutations should be considered for A/H5N1 surveillance studies in outbreak areas. Imai et al. recently identified different RBS changes (N220K, Q222L) along with N154D (affecting the same N-glycosylation sequon as T156A) and T314I in HA as determinants of airborne transmission of an A/H5 virus (58). This airborne virus contained seven genes of the 2009 pandemic A/H1N1 virus (which has S590G/R591Q in PB2 rather than E627K), with the HA of A/H5N1 virus A/Vietnam/1203/2004 (58). These data indicate that different lineages of A/H5N1 virus and different amino acid substitutions that affect particular biological traits (receptor binding, glycosylation, replication) can yield airborne-transmissible A/H5N1 viruses.
Although our experiments showed that A/H5N1 virus can acquire a capacity for airborne transmission, the efficiency of this mode remains unclear. Previous data have indicated that the 2009 pandemic A/H1N1 virus transmits efficiently among ferrets and that naïve animals shed high amounts of virus as early as 1 or 2 days after exposure (27). When we compare the A/H5N1 transmission data with that of reference (27), keeping in mind that our experimental design for studying transmission is not quantitative, the data shown in Figs. 5 and and66 suggest that A/H5N1 airborne transmission was less robust, with less and delayed virus shedding compared with pandemic A/H1N1 virus.
Airborne transmission could be tested in a second mammalian model system such as guinea pigs (59), but this would still not provide conclusive evidence that transmission among humans would occur. The mutations we identified need to be tested for their effect on transmission in other A/H5N1 virus lineages (60), and experiments are needed to quantify how they affect viral fitness and virulence in birds and mammals. For pandemic preparedness, antiviral drugs and vaccine candidates against airborne-transmissible virus should be evaluated in depth. Mechanistic studies on the phenotypic traits associated with each of the identified amino acid substitutions should provide insights into the key determinants of airborne virus transmission. Our findings indicate that HPAI A/H5N1 viruses have the potential to evolve directly to transmit by aerosol or respiratory droplets between mammals, without reassortment in any intermediate host, and thus pose a risk of becoming pandemic in humans. Identification of the minimal requirements for virus transmission between mammals may have prognostic and diagnostic value for improving pandemic preparedness (34).
Acknowledgments
We thank D. de Meulder, G. van Amerongen, and D. Akkermans for technical assistance. M. Peiris, Univ. of Hong Kong, provided A/Indonesia/5/2005 with permission from I. Kandun of the Indonesian government. This work was financed through NIAID-NIH contract HHSN266200700010C. D.J.S. and D.F.B. were supported in part by NIH Director’s Pioneer Award DP1-OD000490-01. We acknowledge a Nederlandse Organisatie voor Wetenschappelijk Onderzoek VICI grant, European Union FP7 program EMPERIE (223498), and Human Frontier Science Program grant P0050/2008. D.F.B. and D.J.S. acknowledge the use of the CamGrid distributed computing resource. Sequence data generated from this study were deposited in GenBank with accession numbers CY116643 to CY116698. Special arrangements are in place with the NIH and the contractor at Mount Sinai School of Medicine, New York, for sharing the viruses (and plasmids) in the present paper; please contact R.A.M.F. A.D.M. E.O. and G.F.R. are CSO and part-time employee of ViroClinics Biosciences BV. A.D.M.E.O. has advisory affiliations on behalf of Viroclinics Biosciences BV with GlaxoSmithKline, Novartis, and Roche. A.D.M.E.O. and R.A.M.F. are holders of certificates of shares in ViroClinics Biosciences B.V.
Footnotes
To avoid any possible conflict of interests, Erasmus MC policy dictates that the shares as such are held by the Stichting Administratiekantoor Erasmus Personeelsparticipaties. The board of this foundation is appointed by the Board of Governors of the Erasmus MC and exercises all voting rights with regard to these shares.
Supplementary Materials
www.sciencemag.org/cgi/content/full/336/6088/1534/DC1
Materials and Methods
References (61–72)
References and Notes
Full text links
Read article at publisher's site: https://doi.org/10.1126/science.1213362
Read article for free, from open access legal sources, via Unpaywall:
https://europepmc.org/articles/pmc4810786?pdf=render
Citations & impact
Impact metrics
Citations of article over time
Alternative metrics
Article citations
The host tropism of current zoonotic H7N9 viruses depends mainly on an acid-labile hemagglutinin with a single amino acid mutation in the stalk region.
PLoS Pathog, 20(10):e1012427, 22 Oct 2024
Cited by: 0 articles | PMID: 39436936 | PMCID: PMC11495601
Interdisciplinary Animal Research Ethics-Challenges, Opportunities, and Perspectives.
Animals (Basel), 14(19):2896, 08 Oct 2024
Cited by: 0 articles | PMID: 39409845 | PMCID: PMC11475729
Leveraging Synthetic Virology for the Rapid Engineering of Vesicular Stomatitis Virus (VSV).
Viruses, 16(10):1641, 21 Oct 2024
Cited by: 0 articles | PMID: 39459973 | PMCID: PMC11512388
Rapid evolution leads to extensive genetic diversification of cattle flu Influenza D virus.
Commun Biol, 7(1):1276, 07 Oct 2024
Cited by: 0 articles | PMID: 39375524 | PMCID: PMC11458855
Is bird flu spreading among people? Data gaps leave researchers in the dark.
Nature, 19 Sep 2024
Cited by: 0 articles | PMID: 39300268
Go to all (1,002) article citations
Other citations
Data
Data behind the article
This data has been text mined from the article, or deposited into data resources.
BioStudies: supplemental material and supporting data
Nucleotide Sequences (2)
- (1 citation) ENA - CY116643
- (1 citation) ENA - CY116698
Similar Articles
To arrive at the top five similar articles we use a word-weighted algorithm to compare words from the Title and Abstract of each citation.
Experimental adaptation of an influenza H5 HA confers respiratory droplet transmission to a reassortant H5 HA/H1N1 virus in ferrets.
Nature, 486(7403):420-428, 02 May 2012
Cited by: 917 articles | PMID: 22722205 | PMCID: PMC3388103
H5N1 hybrid viruses bearing 2009/H1N1 virus genes transmit in guinea pigs by respiratory droplet.
Science, 340(6139):1459-1463, 02 May 2013
Cited by: 153 articles | PMID: 23641061
The potential for respiratory droplet-transmissible A/H5N1 influenza virus to evolve in a mammalian host.
Science, 336(6088):1541-1547, 01 Jun 2012
Cited by: 211 articles | PMID: 22723414 | PMCID: PMC3426314
The Pandemic Threat of Emerging H5 and H7 Avian Influenza Viruses.
Viruses, 10(9):E461, 28 Aug 2018
Cited by: 85 articles | PMID: 30154345 | PMCID: PMC6164301
Review Free full text in Europe PMC
Funding
Funders who supported this work.
European Commission FP7 (1)
Grant ID: FP7_223498
Intramural NIH HHS (1)
Grant ID: ZIA AI001179-01
NIAID NIH HHS (1)
Grant ID: HHSN266200700010C
NIH HHS (2)
Grant ID: DP1 OD000490
Grant ID: DP1-OD000490-01