Abstract
Free full text

The role of skeletal-muscle-based thermogenic mechanisms in vertebrate endothermy
Abstract
Thermogenesis is one of the most important homeostatic mechanisms that evolved during vertebrate evolution. Despite its importance for the survival of the organism, the mechanistic details behind various thermogenic processes remain incompletely understood. Although heat production from muscle has long been recognized as a thermogenic mechanism, whether muscle can produce heat independently of contraction remains controversial. Studies in birds and mammals suggest that skeletal muscle can be an important site of non-shivering thermogenesis (NST) and can be recruited during cold adaptation, although unequivocal evidence is lacking. Much research on thermogenesis during the last two decades has been focused on brown adipose tissue (BAT). These studies clearly implicate BAT as an important site of NST in mammals, in particular in newborns and rodents. However, BAT is either absent, as in birds and pigs, or is only a minor component, as in adult large mammals including humans, bringing into question the BAT-centric view of thermogenesis. This review focuses on the evolution and emergence of various thermogenic mechanisms in vertebrates from fish to man. A careful analysis of the existing data reveals that muscle was the earliest facultative thermogenic organ to emerge in vertebrates, long before the appearance of BAT in eutherian mammals. Additionally, these studies suggest that muscle-based thermogenesis is the dominant mechanism of heat production in many species including birds, marsupials, and certain mammals where BAT-mediated thermogenesis is absent or limited. We discuss the relevance of our recent findings showing that uncoupling of sarco(endo)plasmic reticulum Ca2+-ATPase (SERCA) by sarcolipin (SLN), resulting in futile cycling and increased heat production, could be the basis for NST in skeletal muscle. The overall goal of this review is to highlight the role of skeletal muscle as a thermogenic organ and provide a balanced view of thermogenesis in vertebrates.
I. INTRODUCTION
The maintenance of a constant core body temperature Tc (Mitchell et al., 1992) is one of the most exquisite homeostatic mechanisms of modern-day birds and mammals. Present-day endotherms have both the ability to produce heat (thermogenesis) as well as dissipate heat, which enables them to maintain Tc in hot and/or cold environments. Importantly, the evolution of thermogenic mechanisms in vertebrates has enabled species not only to survive in fluctuating environments but also to invade new territories from the Arctic to the Antarctic. However, the origins and evolution of thermogenic mechanisms in vertebrates remain poorly understood. Current knowledge of thermogenesis is largely derived from studies performed in present-day mammals (mostly rodents) and to a lesser extent in birds. These studies suggest that during cold exposure both shivering and non-shivering thermogenic mechanisms are activated in mammals and birds (Chaffee & Roberts, 1971; Block, 1994; Silva, 2011). It is well known that shivering, a repetitive process of muscle contraction, is an important mechanism of heat production and is a first line of defence during acute cold exposure. Studies on various genetically modified mouse models show that shivering alone is insufficient for the maintenance of Tc and non-shivering mechanisms must be activated to sustain heat production (Thomas & Palmiter, 1997; Guerra et al., 1998; Bachman et al., 2002; Ukropec et al., 2006; Arruda et al., 2008; Aydin et al., 2008; Anunciado-Koza et al., 2011; Bal et al., 2012).
Pioneering studies performed in newborn mammals and adult rodents have identified brown adipose tissue (BAT) as an important site of heat production via non-shivering thermogenesis (NST) (Dawkins & Scopes, 1965; Heim & Hull, 1966; Heim, Kellermayer & Dani, 1968; Knight & Myant, 1970; Alexander, Bennett & Gemmell, 1975; Lean & James, 1983; Lean et al., 1986; Casteilla et al., 1989; Giralt et al., 1990; Houstek et al., 1993; Zancanaro et al., 1995; Cambon, Reyne & Nougues, 1998; Rose et al., 1999; Cannon & Nedergaard, 2004). Rodents (the most common animal model used in research), like neonates, rely heavily on BAT for thermogenesis; as a result, much of the research on NST during the last two decades has been focused on BAT. There is no doubt that these studies have contributed to a greater understanding of BAT-dependent thermogenesis in mammals, but at the same time, these studies have overlooked the existence of other equally important NST mechanisms, especially the role of skeletal muscle. While BAT function is important in many mammals, it is most often restricted to neonatal stages and is downregulated in adult large non-hibernating mammals, including humans. In addition, there are several endotherms such as birds, marsupials, and wild boars that can maintain constant Tc and thrive in cold climates without BAT. Several studies have suggested that skeletal muscle could be an important site of NST (Davis, 1967; Barre et al., 1989; Bourhim et al., 1990; Duchamp et al., 1991; Duchamp & Barre, 1993; Eldershaw et al., 1997); however, the mechanistic details of muscle-based NST are not completely understood.
In addition to cold-induced thermogenesis, NST mechanisms have been shown to be recruited in diet-induced thermogenesis (Duchamp et al., 1993) as a way to increase energy expenditure during diet overload (Lowell & Spiegelman, 2000; Feldmann et al., 2009; Tseng, Cypess & Kahn, 2010). This area of research has received considerable attention because it can be targeted to increase energy expenditure, thereby providing a therapeutic strategy against obesity (Lowell & Spiegelman, 2000; Tseng et al., 2010). Indeed, in recent years, there has been renewed interest in finding ways of increasing BAT activity in adult humans as a strategy to increase energy expenditure. However, to tackle the recent obesity epidemic, a detailed understanding of other NST mechanisms in humans is of increasing significance.
The purpose of this review is (i) to trace the origins of various thermogenic processes throughout vertebrate evolution, (ii) to highlight the role of skeletal muscle as the original thermogenic organ, and lastly, (iii) to show that skeletal muscle is an important site of NST. In addition, we will discuss the relative contributions of muscle versus BAT-dependent thermogenic mechanisms in extant endothermic homeotherms. A major emphasis is placed on illustrating how muscle-based thermogenesis might be critical in animals where BAT is absent or limited in adult life. In addition, we discuss recent data from our laboratory that implicates uncoupling of the sarco(endo)plasmic reticulum Ca2+-ATPase (SERCA) pump by sarcolipin (SLN) as a mechanism for skeletal-muscle-based NST. This finding suggests that skeletal muscle has the capacity to activate futile Ca2+ cycling as a mechanism to generate heat. Thus, the overall goal of this review is to highlight the importance of skeletal muscle to thermogenesis throughout vertebrate evolution.
In addition to thermogenesis, many other adaptive features contributed to the evolution of endothermic homeothermy among vertebrates. These include: behavioural adaptations such as parental care and nesting/herding, heat conservation (by fur, feather, integument modifications), heterothermic adaptations (that reduce the energy cost of rigid homeothermy) such as torpor and hibernation, and predation and predator evasion, as these adaptations include locomotion thereby energy expenditure. Moreover, neurohormonal control of the activation and maintenance of thermogenesis will also have played a key role in the evolution of homeothermy. Such adaptations are beyond the scope of this review.
II. EVOLUTION OF SKELETAL MUSCLE AS A THERMOGENIC ORGAN
(1) Muscle is intrinsically capable of producing heat
Muscle contractions from physical activity generate considerable amounts of heat, and contraction-based heat production is exploited by shivering during cold exposure (Kosaka, Simon & Thauer,1967a; Kosaka, Takagi & Koyama, 1967b; Kosaka & Simon, 1968; Minaire & Chatonnet, 1968; Tkachenko, 1968; Nikki, 1969; Tanche & Therminarias, 1969; Chaffee & Roberts, 1971; Vybiral & Jansky, 1974; Lefaucheur et al., 2001; van Marken Lichtenbelt & Daanen, 2003; Silva, 2011). Indeed, when maximally recruited, as during exercise or an intense bout of shivering, muscle can account for up to 90% of whole-body oxygen uptake, an indirect measure of heat production (Stainsby & Lambert, 1979; Zurlo et al., 1990). During muscle contraction, heat is generated by the hydrolysis of ATP from three different ATPases: myosin ATPase (Stewart et al., 2010; Cooke, 2011; Little & Seebacher, 2013), which performs the contractile work, and SERCA (Block, 1994; Dumonteil, Barre & Meissner, 1995; Simonides et al., 2001; Morrissette, Franck & Block, 2003; de Meis, Arruda & Carvalho, 2005; Arruda et al., 2007; Kjelstrup et al., 2008; Bal et al., 2012; Inesi & Tadini-Buoninsegni, 2013; Little & Seebacher, 2013; Sahoo et al., 2013) and Na+/K+ ATPase (Guernsey & Morishige, 1979; Muller & Seitz, 1984; Herpin, McBride & Bayley, 1987; Kelly & McBride, 1990; Rolfe & Brown, 1997; Karbowski, 2009), which reset resting ion gradients and membrane potential. To sustain these processes, ATP generation must be increased to match demand. These obligatory metabolic processes also generate heat, adding to total heat production.
(2) Muscle as the first organ to be recruited in heat production
Striated muscle can be considered the most primitive facultative thermogenic organ. Fish were among the earliest vertebrate species to evolve, and evidence for muscle-based heat production can be observed in certain fish species that show characteristics of endothermy. While the vast majority of fish are ectotherms, species from the families Lamnidae (sharks) and Scombridae (tunas) can achieve some level of whole-body homeothermy through a modified myotomal muscle (Dickson & Graham, 2004; Runcie et al., 2009). In these species, this slow-twitch, oxidative muscle is located in a medial location, in contrast to the myotomal muscle of ectothermic fish, permitting heat conservation (Carey & Teal, 1966; Dickson & Graham, 2004). The muscle is also perfused by counter-current heat exchangers, which minimize heat loss (Dickson & Graham, 2004). A known characteristic of these species is their continuous swimming behaviour; these sustained contractions combined with the heat-conservation mechanisms described above result in net heat production and represent the source of endothermy in these fishes (Dizon et al., 1978; Guppy, Hulbert & Hochachka, 1979; Hochachka & Mommsen, 1991; Dickson & Graham, 2004; Runcie et al., 2009).
Another fish species known to exhibit endothermy is the opah (Lampris guttatus). Rather than achieving whole-body homeothermy, opahs have developed regional endothermy, which utilizes localized heat production specifically to warm the cranial region (Runcie et al., 2009). Cranial endothermy is an important ecological adaptation that provides a selective advantage as it protects the central nervous system from rapid changes in ambient temperature and enhances vision and detection of prey. To achieve cranial endothermy, opahs employ contractions of the extraocular muscles in order to elevate cranial temperatures when water temperatures are low (Runcie et al., 2009).
Further evidence for heat production as a result of muscular work is found in the brooding python (Python molurus and Python spilotes spilotes), a reptile that exhibits “facultative endothermy.” Brooding pythons use shivering thermogenesis, i.e. repetitive muscle contractions, to keep their eggs warm during embryonic development but revert to heterothermy after brooding (Hutchiso, Dowling & Vinegar, 1966; Harlow & Grigg, 1984; Slip & Shine, 1988; Grigg, Beard & Augee, 2004). Collectively, the evidence thus shows that heat can be generated by muscle, and that muscle can be recruited, in non-homeothermic species; perhaps this represents the most primitive form of regulated heat production. In fact, it has been postulated that behavioural thermoregulation and facultative endothermy were factors involved in the attainment of homeothermy (Hochachka & Mommsen, 1991). Modifications of these thermogenic mechanisms, along with a sophisticated thermoregulatory system, likely contributed to the development of obligate endothermy (Hochachka & Mommsen, 1991; Grigg et al., 2004). Acquiring insulation, such as fur, feathers, and subcutaneous fat would additionally have contributed to this process.
(3) The role of skeletal muscle in the transition from ectothermy to endothermy
Out of all groups of vertebrates only birds and mammals achieved whole-body homeothermy. For centuries the specific mechanism(s) that led to the evolution of endothermy among vertebrates have remained elusive despite intense research effort. Comparative analyses of ectotherms and endotherms have provided clues to the heat-producing mechanisms that must have defined the path to the evolution of endothermy. When comparing similar-sized reptiles (ectotherms) to mammals (endotherms), skeletal muscle is found to be much more massive (30% greater) in mammals (Ruben, 1995). Based on such comparative analyses it has been proposed that the expansion of skeletal muscle mass was a key adaptation during the evolutionary transition of vertebrates from ectothermy to homeothermy (Newman, 2011). Studies also indicate that the surface area of the inner mitochondrial membranes in muscle is 220% greater in mammals than in reptiles and isolated mammalian mitochondrial membranes have twice the enzymatic activity (Else & Hulbert, 1985; Hulbert & Else, 1989; Ruben, 1995). Because the energetic requirements for thermogenesis are high, an increase in mitochondrial respiratory capacity suggests that muscle must have played a key role as a site for thermogenesis during the adaptive transition towards homeothermy. Therefore, increased skeletal muscle mass, along with the means to sustain higher metabolic activity are likely to have been critical in the acquisition of endothermy among vertebrates. However, due to the different genetic backgrounds of ectotherms and endotherms (i.e. reptiles versus mammals), direct comparisons cannot clearly define the mechanism(s) that mediated the transition from ectothermy to endothermy.
To circumvent this problem, researchers have utilized chicken embryos as an ideal model system to study the mechanisms underlying the transition from ectothermy to endothermy (Walter & Seebacher, 2007, 2009; Ijiri et al., 2009). Before hatching, chick embryos switch from an ectothermic state to an endothermic state; therefore, one can perform controlled experiments without potentially confounding species-dependent variations. Observations from chick embryos show that muscle acquires a more oxidative phenotype during the transition, and respiration in skeletal muscle increases after hatching, whereas respiration in the liver remains unaltered (Walter & Seebacher, 2007, 2009; Ijiri et al., 2009). Collectively, these data suggest that muscle is one of the earliest sites of thermogenesis and plays a key role in the transition from ectothermy to endothermy.
III. NON-SHIVERING THERMOGENIC ORIGINS IN SKELETAL MUSCLE
(1) Modified skeletal muscle as a site of NST
The above sections emphasized that muscle can be recruited for thermogenesis, even in non-homeothermic species, but in these cases, the heat-producing capability of muscle is dependent upon contraction. However, muscle can be modified to produce heat in a non-shivering manner without being coupled to contraction. Evidence for muscle-based NST is found in certain fishes with the capacity for regional endothermy. These species, including billfishes, tuna, and mackerel, utilize modified muscle tissue as a means of achieving endothermy (Carey, 1982; Block, 1986, 1994; Dickson & Graham, 2004). This specialized muscle tissue, termed the “heater organ”, is derived from eye muscle and is located in the cranial region. The heater organ is composed of cells that lack the typical myofibrillar structure and have very low expression of actin and myosin (Tullis, Block & Sidell, 1991). Heater organ cells are densely packed with mitochondria and sarcoplasmic reticulum (SR) networks (Hochachka & Mommsen, 1991; Block, 1994). The extensive SR of heater organs is organized into tightly packed stacks, which optimizes surface area for SERCA. Studies have indicated that, in addition to SERCA, the heater organ expresses a Ca2+ release channel (CRC) and calsequestrin (CASQ), which are distributed rather homogeneously throughout the SR (Block & Kim, 1992; Block, Obrien & Meissner, 1994). This is in contrast to skeletal muscle, where the CRC and CASQ are commonly located at specific sites in the SR, called triad junctions. Although the precise mechanism of heat production in heater organ cells has not been proven, it has been linked to Ca2+ transport across the SR membrane. The mechanism proposed is as follows: neuronal stimulation depolarizes the heater cell, which leads to Ca2+ release from the SR. The resulting increase in cytoplasmic Ca2+ stimulates SERCA to actively remove the Ca2+ by utilizing energy derived from ATP hydrolysis. Simultaneously, the free cytosolic Ca2+ can also enter through the mitochondrial uniporter and stimulate mitochondrial respiration (and heat generation) directly (Block, 1994). Thus, in the heater organ, heat is produced by SERCA-catalysed ATP hydrolysis and the stimulation of mitochondrial metabolism required to replenish ATP. Hence, by coupling SR Ca2+-transport with ATP production by mitochondria, and with the loss of myofilaments, a new cell type evolved to produce heat (Fig. 1). Interestingly, the heater organ originated independently in two lineages of fish [billfishes and butterfly mackerel (Gasterochisma melampus)], a fact that highlights the relative ease with which muscle can be adapted to fulfill a thermogenic role (Block, 1994).
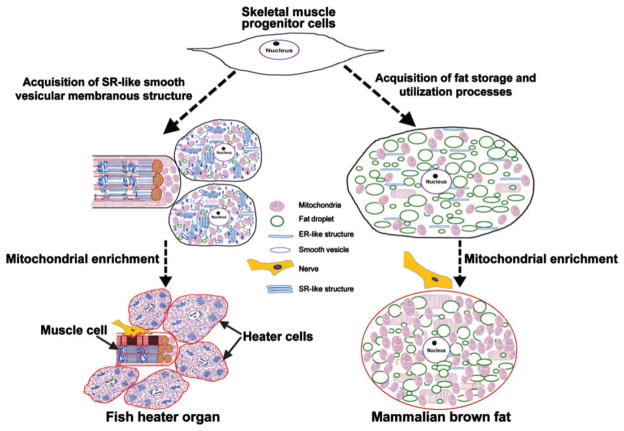
Modification of the skeletal muscle lineage during evolution to acquire a non-shivering thermogenic function. Electron microscopy of the heater organ from various fishes has shown that the sarcoplasmic reticulum (SR) network [with SR Ca2+-ATPase (SERCA), Ca2+ release channel (CRC) and Ca2+-buffering proteins] and mitochondria act in partnership to produce heat (Block & Franzini-Armstrong, 1988; O’Brien & Block, 1996; Londraville et al., 2000; Morrissette et al., 2003). This suggests that during evolution, skeletal muscle progenitor cells must have adapted by decreasing the expression of myofibrillar proteins to give rise to a new cell type that ultimately became heater cells of the fish heater organ. It can be speculated that among mammals, brown adipose tissue (BAT), which is functionally analogous to the fish heater organ, followed a similar evolutionary path. By acquiring more prominent fatty acid uptake, storage, and utilization processes and mitochondrial enrichment, a skeletal-muscle-like progenitor cell could have evolved into a BAT-progenitor cell type that would ultimately become modern (eutherian) BAT cells. Recent findings that skeletal muscle and BAT share a common cellular origin (see Fig. 4) provide support for this scenario. ER, endoplasmic reticulum.
(2) Muscle-based NST in birds
Perhaps the most convincing evidence for NST in skeletal muscle comes from avian species (Barre et al., 1985, 1989; Barre, Bailly & Rouanet, 1987; Duchamp et al., 1989; Dumonteil, Barre & Meissner, 1993, 1994; O’Brien, Meissner & Block, 1993; Dumonteil et al., 1995; Bicudo, Vianna & Chaui-Berlinck, 2001; Raimbault et al., 2001; Bicudo, Bianco & Vianna, 2002; Toyomizu et al., 2002; Ijiri et al., 2009; Walter & Seebacher, 2009). Birds occupy some of the most diverse climates yet maintain the highest body temperatures (38–42°C) among homeotherms (Butler & Woakes, 2001). Some birds including pigeons, geese, and starlings are able to maintain Tc at ~44°C during flight even at ambient temperatures below 0°C (Hart & Roy, 1967; Aulie, 1971; Miller, 1974; Torre-Bueno, 1976). A common feature of birds is that their skeletal muscle is more massive than in comparably sized reptiles and mammals (Else & Hulbert, 1981, 1985; Butler, 2000; Hutchinson, 2004; Newman, 2011; Newman, Mezentseva & Badyaev, 2013): the Japanese quail (Coturnix coturnix japonica) has a skeletal muscle mass that constitutes more than 70% of its body mass (Kawahara & Saito, 1976). Therefore it is speculated that the expansion of avian skeletal muscle, particularly the breast and thigh muscles, provided a unique survival advantage that permitted more efficient heat generation. These muscles are highly specialized for aerobic respiration with high levels of myoglobin and mitochondria, making them well suited for flight (Butler, 1991). Cold-adaptation experiments on birds have shown that, while shivering is the first line of defence used to maintain body temperature as in mammals, birds are also known to use NST mechanisms (Barre et al., 1989; Duchamp et al., 1989, 1991; Duchamp & Barre, 1993; Eldershaw et al., 1997). Hence, the same characteristics that enable flight very likely provide the proper physiological milieu for NST in muscle.
Although others had previously recognized cold-induced NST in birds, C. Duchamp and H. Barre were the first to identify skeletal muscle as the major site of NST (el-Halawani, Wilson & Burger, 1970; Duchamp et al., 1989; Duchamp & Barre, 1993). Using blood-flow measurements from thermoneutral (TN, 25°C) and cold-acclimated (CA, 4°C for 5 weeks) ducklings (Cairina moschata) exposed to 8°C, they were able to show that total muscle blood flow increased equally in the TN and CA ducklings, although the CA ducklings did not shiver. Both groups were also able to maintain Tc in the optimal range (Duchamp & Barre, 1993). Thus, skeletal muscles from CA ducklings were able to produce the same amount of heat as muscles from shivering TN ducklings, demonstrating the existence of NST in skeletal muscle. Similar results have also been found for sparrows (Passer domesticus), king penguin chicks (Aptenodytes patagonicus), and chickens (Gallus domesticus) (Barnett, 1970; el-Halawani et al., 1970; Duchamp et al., 1989; Eldershaw et al., 1997). Although this work defined muscle as the site of NST, it did not provide a detailed mechanism for the source of heat production. In a follow-up study, Dumonteil et al. (1995) performed timed cold exposures to determine how changes in gene expression patterns coincided with the activation of NST. In their experiment, the onset of NST correlated with the timing of increases in SERCA and CRC expression, whereas CASQ levels were unaffected (Dumonteil et al., 1995). Thus, these studies indicated that Ca2+ cycling could be responsible for muscle-based NST. Earlier studies substantiated corroborated these findings by showing increases in both SERCA and the CRC after 6 weeks of cold acclimation in ducklings (Dumonteil et al., 1993). Further support for Ca2+ cycling in NST is the finding that long-chain fatty acylcarnitines and related metabolites induce Ca2+ release from the avian CRC. During cold acclimation, long-chain fatty acids and their metabolites have been shown to be increased in muscle (Dumonteil et al., 1994). Therefore, fatty acyl-CoA/carnitine-induced Ca2+ release could be a mechanism by which Ca2+-cycling is activated in response to cold. Further research is required to define the exact mechanism of NST in avian skeletal muscle.
(3) NST in mammals: evolution of BAT and its role in mammalian thermogenesis
It appears that birds followed an evolutionary pathway that led to muscle hyperplasia along with enrichment in mitochondrial content (Ruben, 1995; Newman, 2011) but did not evolve BAT (Walter & Seebacher, 2007, 2009; Mezentseva, Kumaratilake & Newman, 2008; Teulier et al., 2010). Mammals did not follow the same route. The more recent mammals, the eutherians, invented a whole new tissue type, BAT, which prototherian mammals lack. This evolutionary bifurcation in NST mechanisms could have been due to the ecological and ecogeological constraints prevailing during the times of diversification of these groups from their respective ancestors. During this period, birds adopted an aerial mode of life that permitted muscle hyperplasia and the evolution of muscle-based NST. Mammals, however, remained predominantly terrestrial, and maintenance of Tc from basal metabolism alone became insufficient once cooler land masses began to appear. The appearance of BAT as a specialized thermogenic organ among mammals allowed mammalian habitation of colder climatic zones.
Among vertebrates, only mammals and birds developed whole-body homeothermy. The mammals and birds originated from different amniote lineages at very different time points in vertebrate evolutionary history and thus, must have undergone the transition to endothermic homeothermy independently (Mcnab, 1978; Ruben, 1995; Hillenius & Ruben, 2004; Geiser, 2008; Clarke & Portner, 2010). Synapsids (the lineage including modern mammals) diverged at the very beginning of the amniote radiation during the Late Carboniferous (Luo & Crompton, 1994; Sidor & Hopson, 1998; Luo, 2007; Geiser, 2008), while birds diversified from their sauropsid ancestors at least 80 million years later during the mid-to-late Jurassic (Ruben, 1995; Hillenius & Ruben, 2004; Luo, 2007; Nespolo et al., 2011; Ruta et al., 2013), as depicted in Fig. 2. By the Devonian Period of the Palaeozoic Era, the land plants had established and new ecological niches were available on land for animals to invade. In the Carboniferous, amphibians gave rise to the first amniotes, which made life on land possible. By the mid-Permian, when fauna on land became abundant, positive selection would have existed towards organisms that could remain active (i.e. better predators or escapers) even at low ambient temperatures. This evolutionarily advantageous feature was only possible via mechanisms of endothermic heat production (Clarke & Portner, 2010). Maintenance of elevated metabolic rate and muscular activity (including shivering) might have been the first two mechanisms of endothermic heat production; however, they are very costly and would be limited by nutritional availability in the environment.
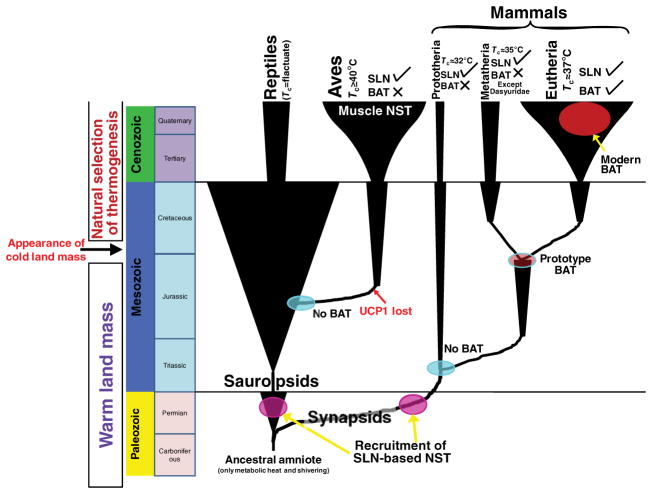
Proposed scheme illustrating the evolution of brown adipose tissue (BAT) and muscle-based thermogenesis that contributed to vertebrate endothermic homeothermy. The common ancestor of amniotes must have been bradymetabolic with metabolic heat (with or without the ability to shiver) as the only source of endothermic heat production. With the appearance of an erect posture (which appeared independently in many sauropsid and synapsid lineages in the mid-to-late Permian) among early amniotes, continuously recruited postural muscles would have provided a feasible molecular environment for sarcolipin (SLN)-based muscle non-shivering thermogenesis (NST) to be recruited. Many of the sauropsid lineages relied on muscle hyperplasia (birds and some dinosaurs) (Newman, 2011), which would have had a twofold benefit: bigger muscles that equated to faster locomotion and better chase/escape ability as well as greater cumulative heat production. An aerial mode of locomotion (i.e. flying) is accompanied by muscle hyperplasia, eliminating the need for additional thermogenic mechanisms. The synapsids took a different route to homeothermy that did not rely primarily on muscle hyperplasia. BAT-based NST has largely been attributed to a eutherian innovation that enabled utilization of uncoupling protein 1 (UCP1) for heat production. However, recent findings have identified the presence of a primitive BAT in a single metatherian species. According to palaeontological evidence, the divergence between Metatheria and Eutheria took place in the Late Jurassic, suggesting that UCP1 presumably was not recruited for thermogenesis until just before metatherian/eutherian divergence. Hence, SLN and UCP1 must have been recruited for thermogenesis at very different time frames in the evolutionary history of vertebrates. The evolution of BAT among mammals also overlaps the evolution of viviparity, which occurred between the prototherian–therian and metatherian–eutherian divergence events. It is interesting to note that birds and monotremes (that lack BAT) are completely oviparous, while only therian mammals (that have BAT) are viviparous. Therefore, BAT may have aided the survival of viviparous newborns, where skeletal muscle is often not fully developed at birth. However, the role of BAT (which can influence the energy trade-off between the neonate and parent) in the evolution of viviparity has not been studied.
The ectothermy to endothermy transition has a close association with the transition from brady- to tachy-metabolism (Bennett & Ruben, 1979; Clarke & Portner, 2010; Hayes, 2010). It seems likely that the ancestral amniotes, like their anamniote tetrapod ancestors, were brady-metabolic with very low basal metabolic rates and an inability to support maximal metabolic rate for long periods. As ancestral amniotes underwent adaptations for terrestrial life (early tetrapods were probably like modern amphibians, needing water to complete their life-cycle), the transition to an erect posture (elevated pelvic joint) must have aided in overcoming the physiological constraints imposed by a sprawling posture, including slow locomotion (friction with ground) and heat loss (ventral surface in close proximity to ground) (Kielan-Jaworowska & Hurum, 2006). Moreover, efficient terrestrial locomotion required animals to overcome “Carrier’s constraint”, the observation that postural and anatomical features precluded the ability to run and breathe at the same time, by adopting an elevated posture along with other skeletal modifications (Carrier, 1987; Brainerd & Owerkowicz, 2006). This sprawling-to-erect postural transition appeared among synapsid and sauropsid lineages independently in the Mid-Permian. In order to sustain the higher energetic demands of the newly evolved postural muscles and running ability, the development of skeletal muscles with both fast contractile properties and a more robust metabolic capacity (characterized by a high number of mitochondria), termed fast-oxidative muscles, must have been necessary. Thus, during the late Permian, it seems possible that skeletal-muscle-based NST must have been used for the first time for a transition to tachy-metabolic status with a rudimentary level of homoeothermy among both synapsid and sauropsid lineages. In order to recruit muscle-based thermogenesis more effectively, increased muscle mass (i.e. muscle hyperplasia) and increased mitochondrial number and activity were required. Palaeontological work on various dinosaur lineages during the last three decades, suggests that many of these lineages had achieved a basal level of homeothermy (Schweitzer & Marshall, 2001; Pontzer, Allen & Hutchinson, 2009; Clarke & Portner, 2010; Kohler et al., 2012; Clarke, 2013; Seymour, 2013), concomitant with muscle hyperplasia, ultimately giving rise to ancestral birds in the Jurassic. Muscle-based NST was further utilized by birds as the sole mechanism for their true endothermic homeothermy [as the uncoupling protein 1 (UCP1) gene was lost and no BAT tissue evolved], supplemented by the anatomical and physiological modifications required for an aerial mode of life, as shown in Fig. 3.
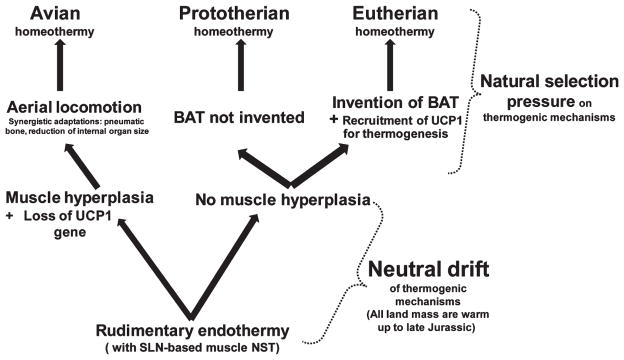
The evolution of two routes of vertebrate homeothermy in mammals. The common ancestor of synapsids and sauropsids must have recruited sarcolipin (SLN)-based muscle non-shivering thermogenesis (NST) achieving a rudimentary level of endothermy. However, for muscle-based NST to be used to achieve homeothermy, the muscle mass to body mass ratio would have to be increased, which only birds achieved. Evolution of thermogenic mechanisms might not have had a selective evolutionary advantage until the appearance of colder climatic niches in the Cretaceous; under this scenario most endothermic mechanisms would have been evolving more or less under neutral drift (because a minimal thermogenic need can be achieved by sustaining an elevated metabolic rate). Ancestral mammals (the common ancestor of Metatheria and Eutheria) evolved brown adipose tissue (BAT) possibly in the Jurassic; the Prototheria do not have this tissue. Thereafter, mammals possessing two adaptive thermogenic processes will have utilized and fine-tuned them according to the demands of their particular environmental/ecological niche.
Mammalian evolution remained shadowed during almost the entirety of the Mesozoic Era by the successful radiation of the dinosaurs. During the Triassic and Jurassic, mammals remained primarily nocturnal (most dinosaurs were diurnal) and small in size (less than 1 kg). Therefore, given these ecological constraints, the evolution of muscle hyperplasia as a means to achieve homeothermy during this period seems unlikely. Moreover, most of the land masses were warm during the Triassic and Jurassic, remaining so until the Early Cretaceous, when some land masses began to become cooler. In this scenario, the evolution of endothermic mechanisms would not have been under positive selection and could occur only by ‘neutral drift’ until conditions changed in the Early Cretaceous. Among mammals, BAT is not found in any prototherian, but recent data shows a primitive form of cold-activated BAT in a metatherian mammal (Sminthopsis crassicaudata; family Dasyuridae) (Jastroch et al., 2008). This prototype BAT could have provided UCP1 with the correct molecular milieu to perform a thermogenic function [elaborately discussed by Saito, Saito & Shingai (2008), Oelkrug et al. (2013) and Hughes et al. (2009)]. Based on these data it seems likely that BAT made its first appearance at or before the diversification of Metatheria and Eutheria, which occurred in the Late Jurassic, but subsequent to the split of Theria and Prototheria. Once colder climatic niches were available starting in the Cretaceous, natural selection would have positively selected animals with better endothermic capability. Further, with the elimination of ~85% of the large reptilian species from the earth during the late Cretaceous mass extinction, many ecological niches became available, allowing the expansion of mammalian species. Eutherians further evolved a sophisticated BAT-based mechanism and show a variety of NST strategies depending on their ecological and physiological requirements.
(4) NST in small versus large mammals: the complementary roles of BAT and skeletal muscle
BAT-dependent thermogenesis is highly efficient in newborn humans and rodents and a great deal of research has focused on this tissue leading to a clear understanding of its mechanism of heat production (Dawkins & Scopes, 1965; Heim & Hull, 1966; Heim et al., 1968; Jenkinson, Noble & Thompson, 1968; Knight & Myant, 1970; Alexander et al., 1975; Lean & James, 1983; Lean et al., 1986; Schubring, 1986; Slebodzinski, 1988; Houstek et al., 1993; Zancanaro et al., 1995; Enerback et al., 1997; Blumberg, Deaver & Kirby, 1999). Support for the existence of BAT-independent NST is provided by the following observations: (i) the transition from ectothermy to endothermy did not depend on the evolution of BAT, and (ii) secondly, BAT is not a characteristic trait of all endotherms (see above and Figs 2 and and3)3) (Grigg et al., 2004). Comparisons of large and small mammals and birds yield striking differences in apparent means of thermogenesis (Table 1). Studies in large mammals, including adult rabbits, dogs, and marsupials, where BAT is less prevalent, underscore the importance of skeletal muscle-based NST in facultative thermogenesis (Davis, 1967; Rose et al., 1999; Arruda et al., 2008); whereas, studies on small mammals, namely rodents, focus on BAT as the principal contributor to NST. These species-specific recruitment strategies are discussed in more detail below.
Table 1
Characteristics and compositions of tissues contributing to thermogenesis in extant endotherms
Characteristic | Eutherian mammals
| Birds | |
---|---|---|---|
Small | Large | ||
BAT (volume) | High (~16% body mass)* | Low (scant to <1%)* | None |
SLN expression | Low, restricted to slow-twitch muscles | High, present in most/all muscles | Unknown |
Muscle composition (fibre type and mitochondrial abundance) | Partitioned fibre types; restricted muscles with abundant mitochondria; preponderance of Type IIB fibres; lesser reliance on postural muscles | Muscles are mostly mixed with high mitochondrial number; considerable reliance on postural muscles; Type IIB fibres minimal or absent | Most muscles rich in mitochondria |
Body temperature | ~37°C | ~37–38°C | 38–42°C |
Surface area to volume ratio | High | Low | High |
Physical activity | Usually bursts of activity (occupy small ecological space) | High range of activity (occupy large ecological space) | Long range of activity (occupy large ecological space) |
BAT, brown adipose tissue; SLN, sarcolipin.
Rodents rely on the heat-generating capacity of BAT to maintain body temperature in response to temperatures below thermoneutrality (Enerback et al., 1997; Cannon & Nedergaard, 2004). BAT is located primarily in the subcutaneous interscapular region (comprising ~60% total BAT volume) of the mouse and rat, in addition to small depots dispersed throughout the body (Frontini & Cinti, 2010). BAT is defined by the presence of multilocular adipocytes, large numbers of mitochondria, and the expression of UCP1 (Heaton, 1972; Matthias et al., 2000). UCP1 is located in the inner mitochondrial membrane and generates heat by dissipating the proton gradient generated by the electron transport chain (Fedorenko, Lishko & Kirichok, 2012). Numerous studies have shown UCP1/BAT to be recruited and activated upon cold exposure. Furthermore, UCP1, and thus BAT, is required for the survival of mice challenged to an acute cold exposure (Enerback et al., 1997; Ukropec et al., 2006). However, UCP1-null mice are able to survive when gradually adapted to cold, indicating the presence of other adaptive thermogenic mechanisms (Golozoubova et al., 2001; Ukropec et al., 2006; Meyer et al., 2010; Shabalina et al., 2010). Unlike rodents, studies on thermogenesis in large mammals point to a much higher reliance on skeletal muscle. In large mammals such as rabbits, ruminants, and humans, BAT activity is downregulated after the neonatal period and is minimally present or not detectable in adulthood, suggesting that skeletal muscle is the primary site of heat production (Lean et al., 1986; Casteilla et al., 1989; Trayhurn, Thomas & Keith, 1993; Cambon et al., 1998; Rose et al., 1999; Lomax et al., 2007). Interestingly, some species even lack a functional Ucp1 gene; pigs lost a functional UCP1 protein ~20 million years ago due to a nonsense mutation (Berg, Gustafson & Andersson, 2006). Pigs maintain Tc at ~37°C, and interestingly, are very susceptible to a deadly condition called malignant hyperthermia, where sustained Ca2+ release by ryanodine receptor 1 in skeletal muscle results in excessive heat production (Fill et al., 1990; MacLennan & Phillips, 1992). This may suggest that pigs employ muscle-based thermogenesis and that its dysregulation can lead to hyperthermia.
Moreover, cold-exposure studies performed on large mammals provide evidence for skeletal-muscle-based NST. Cold-acclimation experiments performed on dogs in which the hindlimbs were denervated to prevent shivering showed that cold-acclimated dogs were able to significantly increase oxygen consumption of the hindlimbs, similar to levels before denervation. Thus, the authors concluded that the denervated skeletal muscle contributed to NST (Davis, 1967). Similar results were obtained for cold exposures on the Tasmanian bettong Bettongia gaimardi, a marsupial in which thermogenic BAT has not been identified (Rose et al., 1999). In addition, in bettongs adapted to cold, heat production and responsiveness to catecholamines are increased. While direct muscle respiration was not measured in this study, earlier experiments had shown that catecholamines stimulated muscle oxygen consumption (Ye et al., 1996; Rose, Kuswanti & Colquhoun, 1998); therefore, it was concluded that muscle contributes to NST in bettongs (Rose et al., 1999).
(5) The recruitment of BAT versus muscle-based NST mechanisms: a physioecological perspective
A clear explanation for the reduced volume of BAT in adult large mammals is not available. Using the concept of “thermal inertia (Hochachka & Mommsen, 1991),” one could hypothesize that the larger surface area to volume ratio of small mammals necessitates a more powerful and efficient NST organ to compensate for the greater heat loss. Larger mammals, with smaller surface area to volume ratios, do not suffer from similar rates of heat loss, and therefore, muscle-based thermogenic mechanisms would be sufficient to maintain T c.
Rodents (i.e. BAT-enriched mammals) possess an abundance of fast-type skeletal muscles, particularly Type IIB fibres (Hesse, Fischer & Schilling, 2010; Schiaffino & Reggiani, 2011). Because of their small size, rodent muscle must be able to contract quickly and more frequently than large mammals (Gasc, 2001; Hesse et al., 2010); in mammals, contraction velocity and stride frequency correlate inversely with body size (Strang & Steudel, 1990; Seow & Ford, 1991). In addition, the effects of gravity on small, lightweight mammals is less than for large mammals, reducing the need for postural muscles, which are typically composed of slow or intermediate oxidative fibres (Hesse et al., 2010). Fast-type muscles, especially Type IIB, rely on glycolytic pathways for ATP production, compared to slow or intermediate fibre types which rely predominantly on oxidative metabolism. Accordingly, the amount of oxidative fibres correlates positively with body mass; therefore, skeletal muscles with more oxidative features, where muscle-based NST would occur, are favoured in large mammals (Emmett & Hochachka, 1981; Hesse et al., 2010).
Muscle composition is also indicative of the ecological coverage and behavioural traits of an animal. Rodents, for instance, have small ecological (spatial) coverage so muscle activity is restricted mostly to small bursts of activity, such as sprinting, as when escaping from a predator. By contrast, most large mammals cover a greater ecological area, even for foraging and other routine activities, necessitating a muscle profile more closely resembling that of a marathon runner (Table 1). Based on these facts, the following explanation seems feasible: because skeletal muscle is used primarily for locomotion, the types and duration of movements a species is adapted for can define the thermogenic capability of the muscle. In simpler terms, animals adapted for extended muscle usage, including locomotion and posture maintenance, will have a greater likelihood of employing muscle-based thermogenesis than animals that require muscle activity for only short periods of time.
IV. SKELETAL MUSCLE AND BAT SHARE A COMMON ORIGIN
(1) BAT and skeletal muscle: divergence from a common cellular precursor
BAT and skeletal muscle are distinct organs with disparate thermogenic mechanisms. However, recent research has established that skeletal muscle and BAT develop from a common progenitor: myogenic factor 5 (Myf5)-expressing precursors, originally believed to give rise specifically to skeletal muscle (Seale et al., 2008). The cell fate switch from skeletal muscle to BAT is controlled by the PR domain-containing 16 (PRDM16) transcriptional complex (Seale et al., 2008; Kajimura et al., 2009; Ohno et al., 2013; Harms et al., 2014). Recent work has shown that the related protein, PRDM3, can also induce this switch in the absence of PRDM16 (Harms et al., 2014). Interestingly, expression of PRDM16 drives brown fat development in Myf5-expressing skeletal myoblasts, whereas loss of PRDM16 from primary brown fat preadipocytes is sufficient to induce differentiation into skeletal muscle. Intriguingly, white adipocytes do not develop from these predecessor cells, thus BAT and white adipose tissue (WAT) have distinct developmental origins (Seale et al., 2008). Moreover, gene expression profiles of BAT, WAT, and skeletal muscle indicate disparities between BAT and WAT, and similarities between muscle and BAT (Timmons et al., 2007). Further, comparisons of mitochondrial proteomic signatures show more similarities between BAT and skeletal muscle than BAT and WAT (Forner et al., 2009). Therefore, BAT, in spite of having some adipocytic features, shares a closer lineage with skeletal muscle (Fig. 4).
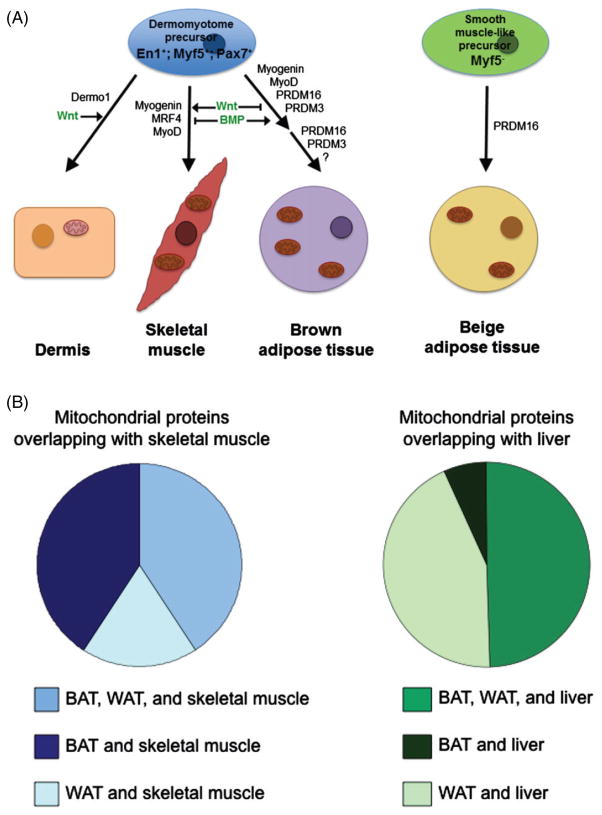
Skeletal muscle and brown adipose tissue share a common cellular lineage. (A) Lineage tracing analyses show that En1, Pax7, and Myf5-expressing cells of the dermomyotome of the paraxial mesoderm are tripotent, giving rise to dermis, skeletal muscle, and brown adipose tissue. Dermis develops from the dermomyotome near the surface ectoderm, and its differentiation is dependent on the expression of Dermo1 and Wnt signalling from the ectoderm (Atit et al., 2006; Gensch et al., 2008; Lepper & Fan, 2010). Skeletal muscle and brown adipose tissue develop from the medial dermomyotome. Skeletal muscle differentiation is initiated by the sequential expression of myogenin, myogenic regulatory factor (MRF4), and myogenic determination factor (MyoD) and by spatiotemporal Wnt signalling from the ectoderm and neural tube (Francetic & Li, 2011; von Maltzahn et al., 2012). Early brown adipose tissue precursors express the myogenic transcription factors myogenin and MyoD but downregulate them upon terminal differentiation (Timmons et al., 2007). Brown fat specification is regulated by expression of PR domain-containing 16 (PRDM16), or the functionally redundant PRDM3 (Harms et al., 2014), and BMP (4 and 7) signalling further directs brown fat differentiation by inhibiting MyoD and Myf5 expression and promoting adipogenesis (Kajimura, Seale & Spiegelman, 2010; Francetic & Li, 2011). Furthermore, Wnt signalling inhibits adipogenesis, promoting skeletal muscle specification (von Maltzahn et al., 2012). Due to common transcription factors (myogenin and MyoD) found in early brown fat and skeletal muscle precursors, it seems possible that the dermomyotome initially gives rise to two cell types rather than three: dermal precursors and a common skeletal muscle/brown fat precursor, which further differentiates into skeletal muscle and brown fat. Details of this process have not been elucidated. The development of beige adipose and white adipose tissue is much less clear. Beige adipose tissue develops from a separate lineage to skeletal muscle and classical brown adipose tissue. Beige fat arises from Myf5-negative precursors of the smooth muscle lineage, and its differentiation is directed by PRDM16 (Cohen et al., 2014; Long et al., 2014). Because smooth muscle develops from heterogeneous cellular lineages (i.e. neural crest, proepicardium, mesothelium, etc.), beige fat may have multiple developmental origins that are depot-specific (Majesky, 2007; Long et al., 2014). White adipose tissue also has diverse cellular origins, developing from ectoderm and mesoderm, including neural crest (ectoderm), mesenchyme (lateral mesoderm), dermis, etc. (Billon & Dani, 2012; Wojciechowicz et al., 2013). (B) Mitochondria are the energetic workhorses of cells; therefore, their properties match the metabolic requirements and/or demand of the cell type. The mitochondrial proteomes of brown adipose tissue (BAT) and white adipose tissue (WAT) were compared to the mitochondrial proteomes of skeletal muscle and liver. A large portion of the proteomes of WAT and BAT overlapped, which mostly included proteins integral to basic mitochondrial structure and function. The mitochondrial proteome of BAT closely resembles that of skeletal muscle. Both are enriched in proteins involved in catabolic processes such as lipolysis, fatty acid metabolism, citric acid cycle, etc. On the other hand, WAT mitochondria share a similar mitochondrial protein profile with liver. WAT mitochondria, in contrast to BAT mitochondria, are specialized for anabolic functions including lipogenesis and detoxification processes. Adapted from Forner et al. (2009)
These new findings might provide some clue to why the two organs share a common function of NST. Both are endowed with characteristics that could support NST, including a high capacity for lipid oxidation, dense mitochondria, neural innervation, and the ability to respond to circulating adrenergic factors through beta-adrenoreceptors (Farmer, 2008; Forner et al., 2009). It can be speculated that skeletal muscle became the first thermogenic organ in ancestral endotherms, but evolutionary pressure resulted in the development of a more efficient organ devoted entirely to heat production, so as not to interfere with muscle function, when the thermogenic demand became greater. This new organ evolved from one already possessing the capacity to meet the demand. All organisms with skeletal muscle have an inherent ability to generate heat. However, small mammals, especially rodents with prevalent BAT, may be less reliant on muscle NST, while others, including birds and large mammals, actively utilize muscle thermogenesis. Barbara Block, a pioneer in muscle-based thermogenesis, similarly hypothesized that “a source of heat is readily available in all skeletal muscle fibers that cycle Ca2+ for continuous contraction for sustained periods of time” (Block, 1994).
(2) Beige or brite fat: an analog of classical BAT
More recent work in the field of thermogenesis has been focused particularly on the inducible brown fat within the WAT depot, termed “beige” or “brite” fat. Rodent beige fat possesses the thermogenic properties of classical BAT; i.e. expression of UCP1 and increased mitochondrial enzymes, while retaining many of the properties of WAT (Wu et al., 2012; Harms & Seale, 2013; Wu, Cohen & Spiegelman, 2013). At thermoneutrality beige fat is minimally present; however, its differentiation/proliferation can be induced by the same stimuli that induce BAT activity and proliferation, i.e. cold exposure or beta 3-adrenoreceptor agonism (Young, Arch & Ashwell, 1984; Loncar, Afzelius & Cannon, 1988a,1988b; Cousin et al., 1992). In fact, “beiging” can compensate for decreased brown fat activity or in other conditions where thermogenic needs are not met (Xue et al., 2007; Schulz et al., 2013). Excitement has been generated in this field, not only because of its recruitable nature, but because human brown fat has a molecular signature more closely resembling rodent beige fat than classical brown fat, making it an attractive therapeutic target (Wu et al., 2012).
Compared to brown fat, the developmental origin(s) of beige fat are less well defined. Though brown fat and beige fat are functionally similar, they do not originate from the same lineage; beige fat arises from a Myf5-negative lineage (Fig. 4A) (Sanchez-Gurmaches et al., 2012). Interestingly, Long et al. (2014) recently showed that beige fat also develops from a muscle origin, that is, from smooth-muscle-(like) precursors. The precise cellular lineage(s) and developmental factors regulating beige fat specification, however, are yet to be elucidated.
V. THE RECRUITMENT OF CA2+-CYCLING IN MUSCLE AS A NST MECHANISM
For muscle to be an effective site of NST, one or more of the processes present in muscle must be recruitable and adaptable to heat generation in the absence of a muscle contraction. However, it would be disadvantageous to muscle function and energetics to evolve an additional machinery devoted entirely to NST. There are published accounts that indicate a role for all three muscle ATPases as well as metabolic/mitochondrial heat in muscle-based NST (Else & Hulbert, 1987; Hulbert & Else, 1989, 1999; Kelly & McBride, 1990; Couture & Hulbert, 1995; Cadenas et al., 2000; Toyomizu et al., 2002; Fernstrom, Tonkonogi & Sahlin, 2004; Shabalina et al., 2006; Cooke, 2011). However, the evidence for SERCA in NST is much more convincing than that for the myosin ATPase, Na+/K+ ATPase, or mitochondria alone. In fact, SERCA has three unique properties that would permit NST without affecting muscle function: (i) SERCA is abundant in muscle (Vangheluwe et al., 2005; de Jonge et al., 2006; Babu et al., 2007; Periasamy & Kalyanasundaram, 2007; Kinnunen & Manttari, 2012; Fajardo et al., 2013; Lamboley et al., 2013), therefore, a portion of the SERCA population can be recruited without affecting muscle function, (ii) the energy liberated as heat from SERCA can be increased or decreased depending on the cellular conditions (de Meis, 2001, 2002; de Meis et al., 2005; Arruda et al., 2008,2007; Kjelstrup et al., 2008; Mahmmoud, 2008; Mahmmoud & Gaster, 2012), and (iii) the Ca2+-uptake function of SERCA can be uncoupled from ATP hydrolysis by its regulatory partner, sarcolipin (SLN) (Smith et al., 2002; Mall et al., 2006; Bal et al., 2012; Bombardier et al., 2013b; Gorski et al., 2013; Inesi & Tadini-Buoninsegni, 2013; Sahoo et al., 2013; Toyoshima & Cornelius, 2013; Toyoshima et al., 2013; Winther et al., 2013).
(1) SERCA function and heat production
A considerable amount of data has been published concerning the molecular and biochemical basis of NST in rabbits. Most work hinges on the unifying theme that Ca2+ cycling in muscle is the major thermogenic mechanism in rabbits, and by extrapolation, other large mammals where BAT is not a major thermogenic organ. In particular, ATP hydrolysis by SERCA is considered to be the dominant heat producer (de Meis et al., 2005; Arruda et al., 2007; Kjelstrup et al., 2008). When activated by high cytosolic Ca2+ levels, as during excitation–contraction coupling, SERCA pumps Ca2+ into the SR, using the energy derived from ATP hydrolysis to restore the SR load. In the optimal “coupled” state, two Ca2+ ions are transported per ATP hydrolysed (Lytton et al., 1992; Lee & East, 2001; Lee, 2002; Periasamy & Kalyanasundaram, 2007; Periasamy, Bhupathy & Babu, 2008). However, SERCA has a unique ability to become “uncoupled,” a state in which fewer (than two) Ca2+ ions are transported per ATP and the remaining energy from ATP hydrolysis is transformed into heat (Yu & Inesi, 1995; de Meis, 2001). Interestingly, when rabbits are acclimatized to cold, SERCA1 expression is increased in red muscle (SERCA2 levels are unaffected) but not in white muscle (Arruda et al., 2008). Furthermore, in vitro preparations of these cold-acclimatized muscles show that cold exposure increased the heat released during ATP hydrolysis twofold in red muscle, where oxidative (mitochondrial) capacity is at least twofold greater than white muscle. Thus, cold exposure increased the heat-generating capacity of rabbit red muscle (Arruda et al., 2008). These results are intriguingly in agreement with those obtained on cold-exposed birds.
(2) Sarcolipin (SLN)-mediated thermogenesis is important for cold- and diet-induced thermogenesis
Despite suggestions that skeletal muscle could be recruited in NST, the mechanistic basis for muscle-based NST has been poorly understood. Recent studies have identified SLN, a regulator of SERCA, as an important player in muscle-based thermogenesis (Tupling et al., 2011; Bal et al., 2012; Yuan et al., 2012; Bombardier et al., 2013a,b; Gorski et al., 2013; Sahoo et al., 2013). SLN is a 31 amino acid single transmembrane protein that co-localizes with the SERCA pump in the SR of skeletal and cardiac muscles. When bound to SERCA, SLN can uncouple SERCA from Ca2+ transport, and as a result can promote futile cycling of SERCA, increasing ATP hydrolysis and heat production (Smith et al., 2002; Mall et al., 2006). This concept was examined using genetically altered mouse models lacking SLN (Bal et al., 2012). This study demonstrated that loss of SLN renders mice cold-sensitive (when interscapular BAT is ablated), but this cold sensitivity can be rescued when SLN is re-expressed. Interestingly, when fed on a high-fat diet, SLN-null mice developed obesity, while control wild-type mice were less obese but upregulated SLN expression in muscle, which suggested that SLN-based thermogenesis is also important for diet-induced thermogenesis (Bal et al., 2012). In addition, recent structure/function studies suggest that SLN interacts with SERCA in a Ca2+-dependent manner. The binding of SLN to SERCA is unique in that SLN remains bound to SERCA throughout the Ca2+ transport cycle and thus can result in uncoupling of SERCA (Sahoo et al., 2013). These data collectively suggest that the SLN/SERCA interaction could be the basis for increased heat production in muscle.
VI. SARCOLIPIN: THE MISSING LINK FOR SKELETAL-MUSCLE-BASED NST
In rodents, SLN is expressed in a developmental and tissue-specific manner. Protein expression is high in skeletal muscles during late gestation and at birth, then becomes gradually restricted to oxidative muscle fibres in adulthood (Babu et al., 2007). UCP1 expression follows the opposite pattern, with expression beginning at birth and remaining prominent in adulthood (Giralt et al., 1990). In adult rodents, SLN expression is predominant in slow-twitch, oxidative muscles, including the soleus and diaphragm, and in small amounts in the tibialis anterior, extensor digitorum longus, and red portion of the gastrocnemius and quadriceps (Vangheluwe et al., 2005; Babu et al., 2007). Given its putative role in thermogenesis, it is not surprising that SLN is restricted to slow, oxidative muscles in rodents where thermogenesis would most likely occur. In striking contrast is the expression profile of SLN in adult large mammals, where oxidative muscle predominates, coinciding with the reduction in BAT content in adulthood. In rabbits, SLN expression is relatively high in all skeletal muscles thus far examined (Fig. 5B); similar results were found in dog and human skeletal muscle (Fig. 5C). These data suggest that SLN plays a more dominant role in large mammals compared to rodents. In support of this, recent studies showed increased uncoupling of SERCA activity in rabbit red muscle after cold adaptation, which was associated with the induction of SERCA1 (de Meis, 2001; Arruda et al., 2003, 2008). In rabbits, SLN expression correlates highly with SERCA1a expression (Fig. 5B). Therefore, it is very likely that SLN was also upregulated after cold exposure and is responsible for this increased uncoupling. However, because this study was performed before SLN was implicated in NST, SLN expression or function was not determined.
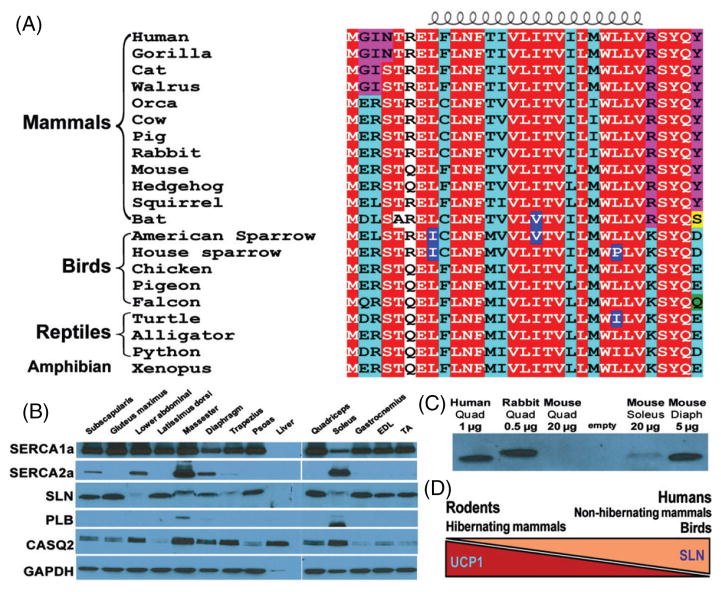
Sarcolipin (SLN) is evolutionarily conserved and is abundant in large mammals. (A) Alignment of SLN protein sequences from various vertebrates. SLN is highly conserved among vertebrate species. Importantly, the transmembrane and C-terminal domains, responsible for inhibition of sarco(endo)plasmic reticulum Ca2+ ATPase (SERCA), are remarkably conserved from amphibians to humans. The N-terminal region has unique conservation among different vertebrate groups; however, the physiological relevance of these unique residues has yet to be studied. All sequences were obtained from GenBank. The house sparrow (Passer domesticus) sequence was obtained, by our laboratory (L.A. Rowland and N.C. Bal), by DNA cloning from the pectoralis muscle and is highly homologous to the published SLN sequence of the American sparrow (Zonotrichia albicollis). The function of SLN in avian species is yet to be investigated. (B) Analyses of SLN and SERCA expression in rabbit skeletal muscle. SLN is abundantly expressed in most skeletal muscle tissues, whereas the homologous protein, phospholamban (PLB), is only found in slow muscles. CASQ2, calsequestrin; EDL, extensor digitorum longus; TA, tibialis anterior. (C) Expression of SLN in large mammals, including humans and dogs (Vangheluwe et al., 2005; Babu et al., 2007), is significantly greater than in rodents. SLN protein is detectable in 0.5 and 1.0 μg of rabbit and human quadriceps muscles, respectively, but is not detectable in 20 μg mouse quadriceps. In mouse muscles where SLN protein is detectable (soleus and diaphragm), total SLN content is still significantly lower than in rabbit and human muscle. Quad, quadriceps; Diaph, diaphragm. (D) Proposed model showing relative contributions of uncoupling protein 1 (UCP1) and SLN to thermogenesis in birds and mammals. We propose that in adult humans, birds, and other non-hibernating mammals, SLN-based muscle thermogenesis constitutes the largest component of thermogenesis, whereas in rodents and hibernating mammals, UCP1 (BAT) is the dominant heat producer. Tissues used to generate (B) and (C) were approved by the institutional animal care committee and institutional review board.
Evidence for SLN-mediated thermogenesis in muscle throughout vertebrates can be found by analysing SLN gene/protein structure; SLN sequences are highly conserved across species from fish to mammals (Fig. 5A). Such high conservation among species indicates that SLN may have played a critical role in the evolution of thermogenesis and remains conserved in animals that rely heavily on skeletal muscle thermogenesis. However, to support the energetic requirements of SLN-based thermogenesis, substantial skeletal muscle oxidative capacity is necessary. Therefore, SLN-based thermogenesis must have evolved in concert with the evolution of fast muscles with high oxidative capacity, a trait that probably evolved in the ancestral lineages that gave rise to homeothermic vertebrates (birds and mammals, see Fig. 2).
Based on these new observations and careful re-analysis of the existing data, we now propose a new model for the major thermogenic components of endotherms and their relative contributions (Fig. 5D). In adult non-hibernating mammals, including humans, where UCP1 and BAT are limited, SLN is the dominant source of thermogenesis. By contrast, in species where UCP1 and BAT are abundant, the contribution of SLN to thermogenesis is secondary to that of BAT.
VII. CONCLUSIONS
We have attempted to provide a critical analysis of the origin and evolution of thermogenic mechanisms in vertebrates, in particular, the contributions of BAT and muscle to NST. Our analyses suggest that skeletal-muscle-based thermogenesis preceded BAT during evolution, with BAT not evolving until the appearance of placental mammals. Since the divergence of placental mammals occurred after the appearance of endothermy in vertebrate evolution, this suggests that another thermogenic process must have been present prior to this diversification. Here, we provide a thorough analysis of the literature to show that skeletal muscle was likely this thermogenic mechanism. We provide data to show that even when BAT is present muscle is still a dominant thermogenic organ.
However, there remain important gaps in our knowledge with regard to the role of each of these mechanisms in different mammalian species. Although existing data suggest that both these mechanisms are recruited, the unique roles of each of these mechanisms in acute cold exposure versus gradual cold adaptation remain unclear. Why certain species evolved a preferential utilization of BAT versus muscle or vice versa, why BAT evolved in some species but not others (birds, marsupials, etc.) and whether either mechanism can compensate for the loss of the other remains unknown.
Our sequence comparison reveals that SLN is highly conserved from fish to man, suggesting that SLN could potentially be involved in thermogenesis in non-mammalian endotherms. However, it remains to be determined if SLN has the same function in all vertebrates. In addition, relatively little information is available concerning thermogenic mechanisms in avian species, especially regarding the role of SLN in avian skeletal muscle. Although putative sequences have been identified in many bird and fish species, expression of SLN mRNA or protein has not been investigated. The most promising evidence, so far, has been the identification of SLN-like mRNA sequences in a chicken expressed sequence tag (EST) (BX935884.1) and catfish EST (AF227818.1), both from muscle, although experimental confirmation is lacking.
Unfortunately, the answers to many of these questions regarding the contribution of muscle to the evolution of endothermy lie within extinct species, particularly those that were present at different diversification events. The challenge now relies on the ability of researchers to integrate studies from diverse extant species and fossil records to begin to understand some of these answers. An ongoing challenge for researchers studying skeletal muscle thermogenesis has been the delineation of heat production originating from contraction versus NST processes. Therefore, new methodologies to localize and capture thermogenesis more precisely in vivo, particularly skeletal muscle-based NST, must be developed.
With an increase in obesity worldwide, there is a significant interest in thermogenic mechanisms as a strategy to increase energy expenditure in humans. With skeletal muscle being a major consumer of metabolites, activation of NST could be an effective strategy to increase energy expenditure. However, the mechanism(s) by which muscle-based NST is activated and recruited is not known, including whether this is under the regulation of hormonal and/or neuronal circuits. Because skeletal muscle contractile activity is primarily activated through nervous stimulation, whether muscle-based NST could be recruited through this mechanism remains to be understood. Some studies suggest a potential role for hormonal regulation of NST in muscle: observations that dogs with denervated skeletal muscle can maintain NST suggests that hormonal factors are involved in the recruitment of NST (Davis, 1967). Other studies have also pointed to various neurohormonal factors in the regulation of NST, including catecholamines, thyroid hormones, glucagon, etc. (Barre, Cohen-Adad & Rouanet, 1987; Eldershaw et al., 1997; Marmonier et al., 1997; Thomas & Palmiter, 1997; Rose et al., 1999; Arruda et al., 2003, 2008; Silva, 2006).
The detailed molecular mechanisms of the SLN–SERCA interaction, including uncoupling of SERCA leading to heat production is not well understood, nor are the signalling mechanisms, including selective activation of Ca2+ cycling, that promote SLN-based heat production. We believe that understanding the detailed molecular basis of thermogenesis has important clinical implications in regulating whole-body metabolism and obesity.
Acknowledgments
We thank John P. Hunter and Christine M. Janis for critical reading and comments on the manuscript. We thank Joseph B. Williams for sharing bird muscle samples. N.C.B. thanks Colleen G. Farmer, Fritz Geiser, Stewart Nicol, and Elias T. Polymeropoulos for informative discussions.
IX. REFERENCES
- Alexander G, Bennett JW, Gemmell RT. Brown adipose tissue in the new-born calf (Bos taurus) Journal of Physiology. 1975;244:223–234. [Abstract] [Google Scholar]
- Anunciado-Koza RP, Zhang J, Ukropec J, Bajpeyi S, Koza RA, Rogers RC, Cefalu WT, Mynatt RL, Kozak LP. Inactivation of the mitochondrial carrier SLC25A25 (ATP-Mg2+/Pi transporter) reduces physical endurance and metabolic efficiency in mice. Journal of Biological Chemistry. 2011;286:11659–11671. [Europe PMC free article] [Abstract] [Google Scholar]
- Arruda AP, Da-Silva WS, Carvalho DP, De Meis L. Hyperthyroidism increases the uncoupled ATPase activity and heat production by the sarcoplasmic reticulum Ca2+ —ATPase. Biochemical Journal. 2003;375:753–760. [Europe PMC free article] [Abstract] [Google Scholar]
- Arruda AP, Ketzer LA, Nigro M, Galina A, Carvalho DP, de Meis L. Cold tolerance in hypothyroid rabbits: role of skeletal muscle mitochondria and sarcoplasmic reticulum Ca2+ ATPase isoform 1 heat production. Endocrinology. 2008;149:6262–6271. [Abstract] [Google Scholar]
- Arruda AP, Nigro M, Oliveira GM, de Meis L. Thermogenic activity of Ca2+ —ATPase from skeletal muscle heavy sarcoplasmic reticulum: the role of ryanodine Ca2+ channel. Biochimica et Biophysica Acta. 2007;1768:1498–1505. [Abstract] [Google Scholar]
- Atit R, Sgaier SK, Mohamed OA, Taketo MM, Dufort D, Joyner AL, Niswander L, Conlon RA. Beta-catenin activation is necessary and sufficient to specify the dorsal dermal fate in the mouse. Developmental Biology. 2006;296:164–176. [Abstract] [Google Scholar]
- Aulie A. Body temperatures in pigeons and budgerigars during sustained flight. Comparative Biochemistry and Physiology. 1971;39:173–176. [Abstract] [Google Scholar]
- Aydin J, Shabalina IG, Place N, Reiken S, Zhang SJ, Bellinger AM, Nedergaard J, Cannon B, Marks AR, Bruton JD, Westerblad H. Nonshivering thermogenesis protects against defective calcium handling in muscle. FASEB Journal. 2008;22:3919–3924. [Europe PMC free article] [Abstract] [Google Scholar]
- Babu GJ, Bhupathy P, Carnes CA, Billman GE, Periasamy M. Differential expression of sarcolipin protein during muscle development and cardiac pathophysiology. Journal of Molecular and Cellular Cardiology. 2007;43:215–222. [Europe PMC free article] [Abstract] [Google Scholar]
- Bachman ES, Dhillon H, Zhang CY, Cinti S, Bianco AC, Kobilka BK, Lowell BB. beta AR signaling required for diet-induced thermogenesis and obesity resistance. Science. 2002;297:843–845. [Abstract] [Google Scholar]
- Bal NC, Maurya SK, Sopariwala DH, Sahoo SK, Gupta SC, Shaikh SA, Pant M, Rowland LA, Bombardier E, Goonasekera SA, Tupling AR, Molkentin JD, Periasamy M. Sarcolipin is a newly identified regulator of muscle-based thermogenesis in mammals. Nature Medicine. 2012;18:1575–1579. [Europe PMC free article] [Abstract] [Google Scholar]
- Barnett LB. Seasonal changes in temperature acclimatization of house sparrow, passer-domesticus. Comparative Biochemistry and Physiology. 1970;33:559–578. [Google Scholar]
- Barre H, Bailly L, Rouanet JL. Increased oxidative capacity in skeletal muscles from cold-acclimated ducklings: a comparison with rats. Comparative Biochemistry and Physiology Part B: Biochemistry and Molecular Biology. 1987;88:519–522. [Abstract] [Google Scholar]
- Barre H, Cohen-Adad F, Duchamp C, Rouanet JL. Muscular nonshivering thermogenesis in birds. Thermal Physiology. 1989;871:667–672. [Google Scholar]
- Barre H, Cohen-Adad F, Rouanet JL. Two daily glucagon injections induce nonshivering thermogenesis in Muscovy ducklings. American Journal of Physiology. 1987;252:E616–E620. [Abstract] [Google Scholar]
- Barre H, Geloen A, Chatonnet J, Dittmar A, Rouanet JL. Potentiated muscular thermogenesis in cold-acclimated muscovy duckling. American Journal of Physiology. 1985;249:R533–R538. [Abstract] [Google Scholar]
- Bennett AF, Ruben JA. Endothermy and activity in vertebrates. Science. 1979;206:649–654. [Abstract] [Google Scholar]
- Berg F, Gustafson U, Andersson L. The uncoupling protein 1 gene (UCP1) is disrupted in the pig lineage: a genetic explanation for poor thermoregulation in piglets. PLoS Genetics. 2006;2:e129. [Europe PMC free article] [Abstract] [Google Scholar]
- Bicudo JE, Bianco AC, Vianna CR. Adaptive thermogenesis in hummingbirds. Journal of Experimental Biology. 2002;205:2267–2273. [Abstract] [Google Scholar]
- Bicudo JE, Vianna CR, Chaui-Berlinck JG. Thermogenesis in birds. Bioscience Reports. 2001;21:181–188. [Abstract] [Google Scholar]
- Billon N, Dani C. Developmental origins of the adipocyte lineage: new insights from genetics and genomics studies. Stem Cell Reviews. 2012;8:55–66. [Abstract] [Google Scholar]
- Block BA. Structure of the brain and eye heater tissue in marlins, sailfish, and spearfishes. Journal of Morphology. 1986;190:169–189. [Abstract] [Google Scholar]
- Block BA. Thermogenesis in muscle. Annual Review of Physiology. 1994;56:535–577. [Abstract] [Google Scholar]
- Block BA, Franzini-Armstrong C. The structure of the membrane systems in a novel muscle cell modified for heat production. Journal of Cell Biology. 1988;107:1099–1112. [Europe PMC free article] [Abstract] [Google Scholar]
- Block BA, Kim SY. Localization of junctional Sr proteins in the thermogenic heater cells of billfish. FASEB Journal. 1992;6:A429. [Google Scholar]
- Block BA, Obrien J, Meissner G. Characterization of the sarcoplasmic-reticulum proteins in the thermogenic muscles of fish. Journal of Cell Biology. 1994;127:1275–1287. [Europe PMC free article] [Abstract] [Google Scholar]
- Blumberg MS, Deaver K, Kirby RF. Leptin disinhibits nonshivering thermogenesis in infants after maternal separation. American Journal of Physiology. 1999;276:R606–R610. [Abstract] [Google Scholar]
- Bombardier E, Smith IC, Gamu D, Fajardo VA, Vigna C, Sayer RA, Gupta SC, Bal NC, Periasamy M, Tupling AR. Sarcolipin trumps beta-adrenergic receptor signaling as the favored mechanism for muscle-based diet-induced thermogenesis. FASEB Journal. 2013a;27:3871–3878. [Europe PMC free article] [Abstract] [Google Scholar]
- Bombardier E, Smith IC, Vigna C, Fajardo VA, Tupling AR. Ablation of sarcolipin decreases the energy requirements for Ca2+ transport by sarco(endo)plasmic reticulum Ca2+ —ATPases in resting skeletal muscle. FEBS Letters. 2013b;587:1687–1692. [Abstract] [Google Scholar]
- Bourhim M, Barre H, Oufara S, Minaire Y, Chatonnet J, Cohen-Adad F, Rouanet JL. Increase in cytochrome oxidase capacity of BAT and other tissues in cold-acclimated gerbils. American Journal of Physiology. 1990;258:R1291–R1298. [Abstract] [Google Scholar]
- Brainerd EL, Owerkowicz T. Functional morphology and evolution of aspiration breathing in tetrapods. Respiratory Physiology and Neurobiology. 2006;154:73–88. [Abstract] [Google Scholar]
- Butler PJ. Exercise in birds. Journal of Experimental Biology. 1991;160:233–262. [Google Scholar]
- Butler PJ. Energetic costs of surface swimming and diving of birds. Physiological and Biochemical Zoology. 2000;73:699–705. [Abstract] [Google Scholar]
- Butler PJ, Woakes AJ. Seasonal hypothermia in a large migrating bird: saving energy for fat deposition? Journal of Experimental Biology. 2001;204:1361–1367. [Abstract] [Google Scholar]
- Cadenas S, Buckingham JA, St-Pierre J, Dickinson K, Jones RB, Brand MD. AMP decreases the efficiency of skeletal-muscle mitochondria. Biochemical Journal. 2000;351(2):307–311. [Europe PMC free article] [Abstract] [Google Scholar]
- Cambon B, Reyne Y, Nougues J. In vitro induction of UCP1 mRNA in preadipocytes from rabbit considered as a model of large mammals brown adipose tissue development: importance of PPARgamma agonists for cells isolated in the postnatal period. Molecular and Cellular Endocrinology. 1998;146:49–58. [Abstract] [Google Scholar]
- Cannon B, Nedergaard J. Brown adipose tissue: function and physiological significance. Physiological Reviews. 2004;84:277–359. [Abstract] [Google Scholar]
- Carey FG. A brain heater in the swordfish. Science. 1982;216:1327–1329. [Abstract] [Google Scholar]
- Carey FG, Teal JM. Heat conservation in tuna fish muscle. Proceedings of the National academy of Sciences of the United States of America. 1966;56:1464–1469. [Europe PMC free article] [Abstract] [Google Scholar]
- Carrier DR. The evolution of locomotor stamina in tetrapods - circumventing a mechanical constraint. Paleobiology. 1987;13:326–341. [Google Scholar]
- Casteilla L, Champigny O, Bouillaud F, Robelin J, Ricquier D. Sequential changes in the expression of mitochondrial protein mRNA during the development of brown adipose tissue in bovine and ovine species. Sudden occurrence of uncoupling protein mRNA during embryogenesis and its disappearance after birth. Biochemical Journal. 1989;257:665–671. [Europe PMC free article] [Abstract] [Google Scholar]
- Chaffee RR, Roberts JC. Temperature acclimation in birds and mammals. Annual Review of Physiology. 1971;33:155–202. [Abstract] [Google Scholar]
- Clarke A. Dinosaur energetics: setting the bounds on feasible physiologies and ecologies. American Naturalist. 2013;182:283–297. [Abstract] [Google Scholar]
- Clarke A, Portner HO. Temperature, metabolic power and the evolution of endothermy. Biological Reviews of the Cambridge Philosophical Society. 2010;85:703–727. [Abstract] [Google Scholar]
- Cohen P, Levy JD, Zhang Y, Frontini A, Kolodin DP, Svensson KJ, Lo JC, Zeng X, Ye L, Khandekar MJ, Wu J, Gunawardana SC, Banks AS, Camporez JP, Jurczak MJ, Kajimura S, Piston DW, Mathis D, Cinti S, Shulman GI, Seale P, Spiegelman BM. Ablation of PRDM16 and beige adipose causes metabolic dysfunction and a subcutaneous to visceral fat switch. Cell. 2014;156:304–316. [Europe PMC free article] [Abstract] [Google Scholar]
- Cooke R. The role of the myosin ATPase activity in adaptive thermogenesis by skeletal muscle. Biophysical Reviews. 2011;3:33–45. [Europe PMC free article] [Abstract] [Google Scholar]
- Cousin B, Cinti S, Morroni M, Raimbault S, Ricquier D, Penicaud L, Casteilla L. Occurrence of brown adipocytes in rat white adipose tissue: molecular and morphological characterization. Journal of Cell Science. 1992;103(Pt 4):931–942. [Abstract] [Google Scholar]
- Couture P, Hulbert AJ. Relationship between body-mass, tissue metabolic-rate, and sodium-pump activity in mammalian liver and kidney. American Journal of Physiology-Regulatory Integrative and Comparative Physiology. 1995;268:R641–R650. [Abstract] [Google Scholar]
- Davis TR. Contribution of skeletal muscle to nonshivering thermogenesis in the dog. American Journal of Physiology. 1967;213:1423–1426. [Abstract] [Google Scholar]
- Dawkins MJ, Scopes JW. Non-shivering thermogenesis and brown adipose tissue in the human new-born infant. Nature. 1965;206:201–202. [Abstract] [Google Scholar]
- Dickson KA, Graham JB. Evolution and consequences of endothermy in fishes. Physiological and Biochemical Zoology. 2004;77:998–1018. [Abstract] [Google Scholar]
- Dizon AE, Sharp GD & Inter-American Tropical Tuna Commission, Hubbs-Sea World Research Institute & Southwest Fisheries Center (U.S.) The Physiological Ecology of Tunas. Academic Press; New York: 1978. [Google Scholar]
- Duchamp C, Barre H. Skeletal muscle as the major site of nonshivering thermogenesis in cold-acclimated ducklings. American Journal of Physiology. 1993;265:R1076–R1083. [Abstract] [Google Scholar]
- Duchamp C, Barre H, Delage D, Rouanet JL, Cohen-Adad F, Minaire Y. Nonshivering thermogenesis and adaptation to fasting in king penguin chicks. American Journal of Physiology. 1989;257:R744–R751. [Abstract] [Google Scholar]
- Duchamp C, Barre H, Rouanet JL, Lanni A, Cohen-Adad F, Berne G, Brebion P. Nonshivering thermogenesis in king penguin chicks. I. Role of skeletal muscle. American Journal of Physiology. 1991;261:R1438–R1445. [Abstract] [Google Scholar]
- Duchamp C, Chatonnet J, Dittmar A, Barre H. Increased role of skeletal muscle in the calorigenic response to glucagon of cold-acclimated ducklings. American Journal of Physiology. 1993;265:R1084–R1091. [Abstract] [Google Scholar]
- Dumonteil E, Barre H, Meissner G. Sarcoplasmic reticulum Ca(2+)-ATPase and ryanodine receptor in cold-acclimated ducklings and thermogenesis. American Journal of Physiology. 1993;265:C507–C513. [Abstract] [Google Scholar]
- Dumonteil E, Barre H, Meissner G. Effects of palmitoyl carnitine and related metabolites on the avian Ca(2+)-ATPase and Ca2+ release channel. Journal of Physiology. 1994;479(1):29–39. [Abstract] [Google Scholar]
- Dumonteil E, Barre H, Meissner G. Expression of sarcoplasmic reticulum Ca2+ transport proteins in cold-acclimating ducklings. American Journal of Physiology. 1995;269:C955–C960. [Abstract] [Google Scholar]
- Eldershaw TPD, Duchamp C, Ye JM, Clark MG, Colquhoun EQ. Potential for non-shivering thermogenesis in perfused chicken (Gallus domesticus) muscle. Comparative Biochemistry and Physiology Part A: Molecular & Integrative Physiology. 1997;117:545–554. [Abstract] [Google Scholar]
- Else PL, Hulbert AJ. Comparison of the mammal machine and the reptile machine - energy-production. American Journal of Physiology. 1981;240:R3–R9. [Abstract] [Google Scholar]
- Else PL, Hulbert AJ. An allometric comparison of the mitochondria of mammalian and reptilian tissues: the implications for the evolution of endothermy. Journal of Comparative Physiology B. 1985;156:3–11. [Abstract] [Google Scholar]
- Else PL, Hulbert AJ. Evolution of mammalian endothermic metabolism: “leaky” membranes as a source of heat. American Journal of Physiology. 1987;253:R1–R7. [Abstract] [Google Scholar]
- Emmett B, Hochachka PW. Scaling of oxidative and glycolytic-enzymes in mammals. Respiration Physiology. 1981;45:261–272. [Abstract] [Google Scholar]
- Enerback S, Jacobsson A, Simpson EM, Guerra C, Yamashita H, Harper ME, Kozak LP. Mice lacking mitochondrial uncoupling protein are cold-sensitive but not obese. Nature. 1997;387:90–94. [Abstract] [Google Scholar]
- Fajardo VA, Bombardier E, Vigna C, Devji T, Bloemberg D, Gamu D, Gramolini AO, Quadrilatero J, Tupling AR. Co-expression of SERCA Isoforms, phospholamban and sarcolipin in human skeletal muscle fibers. PLoS ONE. 2013;8:e84304. [Europe PMC free article] [Abstract] [Google Scholar]
- Farmer SR. Brown fat and skeletal muscle: unlikely cousins? Cell. 2008;134:726–727. [Abstract] [Google Scholar]
- Fedorenko A, Lishko PV, Kirichok Y. Mechanism of fatty-acid-dependent UCP1 uncoupling in brown fat mitochondria. Cell. 2012;151:400–413. [Europe PMC free article] [Abstract] [Google Scholar]
- Feldmann HM, Golozoubova V, Cannon B, Nedergaard J. UCP1 ablation induces obesity and abolishes diet-induced thermogenesis in mice exempt from thermal stress by living at thermoneutrality. Cell Metabolism. 2009;9:203–209. [Abstract] [Google Scholar]
- Fernstrom M, Tonkonogi M, Sahlin K. Effects of acute and chronic endurance exercise on mitochondrial uncoupling in human skeletal muscle. Journal of Physiology. 2004;554:755–763. [Abstract] [Google Scholar]
- Fill M, Coronado R, Mickelson JR, Vilven J, Ma JJ, Jacobson BA, Louis CF. Abnormal ryanodine receptor channels in malignant hyperthermia. Biophysical Journal. 1990;57:471–475. [Europe PMC free article] [Abstract] [Google Scholar]
- Forner F, Kumar C, Luber CA, Fromme T, Klingenspor M, Mann M. Proteome differences between brown and white fat mitochondria reveal specialized metabolic functions. Cell Metabolism. 2009;10:324–335. [Abstract] [Google Scholar]
- Francetic T, Li Q. Skeletal myogenesis and Myf5 activation. Transcription. 2011;2:109–114. [Europe PMC free article] [Abstract] [Google Scholar]
- Frontini A, Cinti S. Distribution and development of brown adipocytes in the murine and human adipose organ. Cell Metabolism. 2010;11:253–256. [Abstract] [Google Scholar]
- Gasc JP. Comparative aspects of gait, scaling and mechanics in mammals. Comparative Biochemistry and Physiology Part A:Molecular&Integrative Physiology. 2001;131:121–133. [Abstract] [Google Scholar]
- Geiser F. Ontogeny and phylogeny of endothermy and torpor in mammals and birds. Comparative Biochemistry and Physiology Part A: Molecular & Integrative Physiology. 2008;150:176–180. [Abstract] [Google Scholar]
- Gensch N, Borchardt T, Schneider A, Riethmacher D, Braun T. Different autonomous myogenic cell populations revealed by ablation of Myf5-expressing cells during mouse embryogenesis. Development. 2008;135:1597–1604. [Abstract] [Google Scholar]
- Giralt M, Martin I, Iglesias R, Vinas O, Villarroya F, Mampel T. Ontogeny and perinatal modulation of gene expression in rat brown adipose tissue. Unaltered iodothyronine 5′-deiodinase activity is necessary for the response to environmental temperature at birth. European Journal of Biochemistry. 1990;193:297–302. [Abstract] [Google Scholar]
- Golozoubova V, Hohtola E, Matthias A, Jacobsson A, Cannon B, Nedergaard J. Only UCP1 can mediate adaptive nonshivering thermogenesis in the cold. FASEB Journal. 2001;15:2048–2050. [Abstract] [Google Scholar]
- Gorski PA, Glaves JP, Vangheluwe P, Young HS. Sarco(endo)plasmic reticulum calcium ATPase (SERCA) inhibition by sarcolipin is encoded in its luminal tail. Journal of Biological Chemistry. 2013;288:8456–8467. [Europe PMC free article] [Abstract] [Google Scholar]
- Grigg GC, Beard LA, Augee ML. The evolution of endothermy and its diversity in mammals and birds. Physiological and Biochemical Zoology. 2004;77:982–997. [Abstract] [Google Scholar]
- Guernsey DL, Morishige WK. Na+ pump activity and nuclear T3 receptors in tissues of genetically obese (ob/ob) mice. Metabolism. 1979;28:629–632. [Abstract] [Google Scholar]
- Guerra C, Koza RA, Walsh K, Kurtz DM, Wood PA, Kozak LP. Abnormal nonshivering thermogenesis in mice with inherited defects of fatty acid oxidation. Journal of Clinical Investigation. 1998;102:1724–1731. [Europe PMC free article] [Abstract] [Google Scholar]
- Guppy M, Hulbert WC, Hochachka PW. Metabolic sources of heat and power in tuna muscles. II. Enzyme and metabolite profiles. Journal of Experimental Biology. 1979;82:303–320. [Abstract] [Google Scholar]
- el-Halawani Me-S, Wilson WO, Burger RE. Cold-acclimation and the role of catecholamines in body temperature regulation in male Leghorns. Poultry Science. 1970;49:621–632. [Abstract] [Google Scholar]
- Harlow P, Grigg G. Shivering thermogenesis in a brooding diamond python, Python spilotes spilotes. Copeia. 1984;4:959–965. [Google Scholar]
- Harms M, Seale P. Brown and beige fat: development, function and therapeutic potential. Nature Medicine. 2013;19:1252–1263. [Abstract] [Google Scholar]
- Harms MJ, Ishibashi J, Wang W, Lim HW, Goyama S, Sato T, Kurokawa M, Won KJ, Seale P. Prdm16 is required for the maintenance of brown adipocyte identity and function in adult mice. Cell Metabolism. 2014;19:593–604. [Europe PMC free article] [Abstract] [Google Scholar]
- Hart JS, Roy OZ. Temperature regulation during flight in pigeons. The American Journal of Physiology. 1967;213:1311–1316. [Abstract] [Google Scholar]
- Hayes JP. Metabolic rates, genetic constraints, and the evolution of endothermy. Journal of Evolutionary Biology. 2010;23:1868–1877. [Abstract] [Google Scholar]
- Heaton JM. The distribution of brown adipose tissue in the human. Journal of Anatomy. 1972;112:35–39. [Europe PMC free article] [Abstract] [Google Scholar]
- Heim T, Hull D. The blood flow and oxygen consumption of brown adipose tissue in the new-born rabbit. Journal of Physiology. 1966;186:42–55. [Abstract] [Google Scholar]
- Heim T, Kellermayer M, Dani M. Thermal conditions and the mobilization of lipids from brown and white adipose tissue in the human neonate. Acta Paediatrica Academiae Scientiarum Hungaricae. 1968;9:109–120. [Abstract] [Google Scholar]
- Herpin PR, McBride BW, Bayley HS. Effect of cold exposure on energy metabolism in the young pig. Canadian Journal of Physiology and Pharmacology. 1987;65:236–245. [Abstract] [Google Scholar]
- Hesse B, Fischer MS, Schilling N. Distribution pattern of muscle fiber types in the perivertebral musculature of two different sized species of mice. Anatomical Record (Hoboken) 2010;293:446–463. [Abstract] [Google Scholar]
- Hillenius WJ, Ruben JA. The evolution of endothermy in terrestrial vertebrates: Who? When? Why? Physiological and Biochemical Zoology. 2004;77:1019–1042. [Abstract] [Google Scholar]
- Hochachka PW, Mommsen TP. Biochemistry and Molecular biology of Fishes. Elsevier; Amsterdam, New York: 1991. [Google Scholar]
- Houstek J, Vizek K, Pavelka S, Kopecky J, Krejcova E, Hermanska J, Cermakova M. Type II iodothyronine 5′-deiodinase and uncoupling protein in brown adipose tissue of human newborns. Journal of Clinical Endocrinology and Metabolism. 1993;77:382–387. [Abstract] [Google Scholar]
- Hughes DA, Jastroch M, Stoneking M, Klingenspor M. Molecular evolution of UCP1 and the evolutionary history of mammalian non-shivering thermogenesis. BMC Evol Biol. 2009;9:4. [Europe PMC free article] [Abstract] [Google Scholar]
- Hulbert AJ, Else PL. Evolution of mammalian endothermic metabolism: mitochondrial activity and cell composition. American Journal of Physiology. 1989;256:R63–R69. [Abstract] [Google Scholar]
- Hulbert AJ, Else PL. Membranes as possible pacemakers of metabolism. Journal of Theoretical Biology. 1999;199:257–274. [Abstract] [Google Scholar]
- Hutchinson JR. Biomechanical modeling and sensitivity analysis of bipedal running ability. I. Extant taxa. Journal of Morphology. 2004;262:421–440. [Abstract] [Google Scholar]
- Hutchiso V, Dowling HG, Vinegar A. Thermoregulation in a brooding female Indian python Python molurus bivittatus. Science. 1966;151:694–696. [Abstract] [Google Scholar]
- Ijiri D, Miura M, Kanai Y, Hirabayashi M. Increased mass of slow-type skeletal muscles and depressed myostatin gene expression in cold-tolerant chicks. Zoological Science. 2009;26:277–283. [Abstract] [Google Scholar]
- Inesi G, Tadini-Buoninsegni F. Ca/H exchange, lumenal Ca release and Ca /ATP coupling ratios in the sarcoplasmic reticulum ATPase. Journal of Cell Communication and Signalling. 2013;8:5–11. [Europe PMC free article] [Abstract] [Google Scholar]
- Jastroch M, Withers KW, Taudien S, Frappell PB, Helwig M, Fromme T, Hirschberg V, Heldmaier G, McAllan BM, Firth BT, Burmester T, Platzer M, Klingenspor M. Marsupial uncoupling protein 1 sheds light on the evolution of mammalian nonshivering thermogenesis. Physiological Genomics. 2008;32:161–169. [Abstract] [Google Scholar]
- Jenkinson DM, Noble RC, Thompson GE. Adipose tissue and heat production in the new-born ox (Bos taurus) Journal of Physiology. 1968;195:639–646. [Abstract] [Google Scholar]
- de Jonge HW, van der Wiel CW, Eizema K, Weijs WA, Everts ME. Presence of SERCA and calcineurin during fetal development of porcine skeletal muscle. Journal of Histochemistry & Cytochemistry. 2006;54:641–648. [Abstract] [Google Scholar]
- Kajimura S, Seale P, Kubota K, Lunsford E, Frangioni JV, Gygi SP, Spiegelman BM. Initiation of myoblast to brown fat switch by a PRDM16-C/EBP-beta transcriptional complex. Nature. 2009;460:1154–1158. [Europe PMC free article] [Abstract] [Google Scholar]
- Kajimura S, Seale P, Spiegelman BM. Transcriptional control of brown fat development. Cell Metabolism. 2010;11:257–262. [Europe PMC free article] [Abstract] [Google Scholar]
- Karbowski J. Thermodynamic constraints on neural dimensions, firing rates, brain temperature and size. Journal of Computational Neuroscience. 2009;27:415–436. [Abstract] [Google Scholar]
- Kawahara T, Saito K. Genetic parameters of organ and body weights in the Japanese quail. Poultry Science. 1976;55:1247–1252. [Abstract] [Google Scholar]
- Kelly JM, McBride BW. The sodium pump and other mechanisms of thermogenesis in selected tissues. Procedings of the Nutrition Society. 1990;49:185–202. [Abstract] [Google Scholar]
- Kielan-Jaworowska Z, Hurum JH. Limb posture in early mammals: Sprawling or parasagittal. Acta Palaeontologica Polonica. 2006;51:393–406. [Google Scholar]
- Kinnunen S, Manttari S. Specific effects of endurance and sprint training on protein expression of calsequestrin and SERCA in mouse skeletal muscle. Journal of Muscle Research and Cell Motility. 2012;33:123–130. [Abstract] [Google Scholar]
- Kjelstrup S, de Meis L, Bedeaux D, Simon JM. Is the Ca2+ —ATPase from sarcoplasmic reticulum also a heat pump? European Biophysical Journal. 2008;38:59–67. [Abstract] [Google Scholar]
- Knight BL, Myant NB. A comparison between the effects of cold exposure in vivo and of noradrenaline in vitro on the metabolism of the brown fat of new-born rabbits. Biochemical Journal. 1970;119:103–111. [Europe PMC free article] [Abstract] [Google Scholar]
- Kohler M, Marin-Moratalla N, Jordana X, Aanes R. Seasonal bone growth and physiology in endotherms shed light on dinosaur physiology. Nature. 2012;487:358–361. [Abstract] [Google Scholar]
- Kosaka M, Simon E. Central nervous spinal mechanism of cold shivering. Pflügers Archiv. 1968;302:357–373. [Abstract] [Google Scholar]
- Kosaka M, Simon E, Thauer R. Shivering in intact and spinal rabbits during spinal cord cooling. Experientia. 1967a;23:385–387. [Abstract] [Google Scholar]
- Kosaka M, Takagi K, Koyama Y. Reflex inhibition of cold shivering by pressure to the skin and the histological investigation of its afferent spinal pathway. Experientia. 1967b;23:453–455. [Abstract] [Google Scholar]
- Lamboley CR, Murphy RM, McKenna MJ, Lamb GD. Endogenous and maximal sarcoplasmic reticulum calcium content and calsequestrin expression in type I and type II human skeletal muscle fibres. Journal of Physiology. 2013;591:6053–6068. [Abstract] [Google Scholar]
- Lean ME, James WP. Uncoupling protein in human brown adipose tissue mitochondria. Isolation and detection by specific antiserum. FEBS Letters. 1983;163:235–240. [Abstract] [Google Scholar]
- Lean ME, James WP, Jennings G, Trayhurn P. Brown adipose tissue uncoupling protein content in human infants, children and adults. Clinical Science (London) 1986;71:291–297. [Abstract] [Google Scholar]
- Lee AG. A calcium pump made visible. Current Opinion in Structural Biology. 2002;12:547–554. [Abstract] [Google Scholar]
- Lee AG, East JM. What the structure of a calcium pump tells us about its mechanism. Biochemical Journal. 2001;356:665–683. [Europe PMC free article] [Abstract] [Google Scholar]
- Lefaucheur L, Ecolan P, Lossec G, Gabillard JC, Butler-Browne GS, Herpin P. Influence of early postnatal cold exposure on myofiber maturation in pig skeletal muscle. Journal of Muscle Research and Cell Motility. 2001;22:439–452. [Abstract] [Google Scholar]
- Lepper C, Fan CM. Inducible lineage tracing of Pax7-descendant cells reveals embryonic origin of adult satellite cells. Genesis. 2010;48:424–436. [Europe PMC free article] [Abstract] [Google Scholar]
- Little AG, Seebacher F. Thyroid hormone regulates muscle function during cold acclimation in zebrafish (Danio rerio) Journal of Experimental Biology. 2013;216:3514–3521. [Abstract] [Google Scholar]
- Lomax MA, Sadiq F, Karamanlidis G, Karamitri A, Trayhurn P, Hazlerigg DG. Ontogenic loss of brown adipose tissue sensitivity to beta-adrenergic stimulation in the ovine. Endocrinology. 2007;148:461–468. [Abstract] [Google Scholar]
- Loncar D, Afzelius BA, Cannon B. Epididymal white adipose tissue after cold stress in rats. I. Nonmitochondrial changes. Journal of Ultrastructure and Molecular Structure Research. 1988a;101:109–122. [Abstract] [Google Scholar]
- Loncar D, Afzelius BA, Cannon B. Epididymal white adipose tissue after cold stress in rats. II. Mitochondrial changes. Journal of Ultrastructure and Molecular Structure Research. 1988b;101:199–209. [Abstract] [Google Scholar]
- Londraville RL, Cramer TD, Franck JP, Tullis A, Block BA. Cloning of a neonatal calcium atpase isoform (SERCA 1B) from extraocular muscle of adult blue marlin (Makaira nigricans) Comparative Biochemistry and Physiology B. 2000;127:223–233. [Abstract] [Google Scholar]
- Long JZ, Svensson KJ, Tsai L, Zeng X, Roh HC, Kong X, Rao RR, Lou J, Lokurkar I, Baur W, Castellot JJ, Jr, Rosen ED, Spiegelman BM. A smooth muscle-like origin for beige adipocytes. Cell Metabolism. 2014;19:810–820. [Europe PMC free article] [Abstract] [Google Scholar]
- Lowell BB, Spiegelman BM. Towards a molecular understanding of adaptive thermogenesis. Nature. 2000;404:652–660. [Abstract] [Google Scholar]
- Luo ZX. Transformation and diversification in early mammal evolution. Nature. 2007;450:1011–1019. [Abstract] [Google Scholar]
- Luo ZX, Crompton AW. Transformation of the quadrate (incus) through the transition from nonmammalian cynodonts to mammals. Journal of Vertebrate Paleontology. 1994;14:341–374. [Google Scholar]
- Lytton J, Westlin M, Burk SE, Shull GE, Maclennan DH. Functional comparisons between isoforms of the sarcoplasmic or endoplasmic-reticulum family of calcium pumps. Journal of Biological Chemistry. 1992;267:14483–14489. [Abstract] [Google Scholar]
- MacLennan DH, Phillips MS. Malignant hyperthermia. Science. 1992;256:789–794. [Abstract] [Google Scholar]
- Mahmmoud YA. Capsaicin stimulates uncoupled ATP hydrolysis by the sarcoplasmic reticulum calcium pump. Journal of Biological Chemistry. 2008;283:21418–21426. [Abstract] [Google Scholar]
- Mahmmoud YA, Gaster M. Uncoupling of sarcoplasmic reticulum Ca2+ —ATPase by N-arachidonoyl dopamine. Members of the endocannabinoid family as thermogenic drugs. British Journal of Pharmacology. 2012;166:2060–2069. [Europe PMC free article] [Abstract] [Google Scholar]
- Majesky MW. Developmental basis of vascular smooth muscle diversity. Arteriosclerosis, Thrombosis, and Vascular Biology. 2007;27:1248–1258. [Abstract] [Google Scholar]
- Mall S, Broadbridge R, Harrison SL, Gore MG, Lee AG, East JM. The presence of sarcolipin results in increased heat production by Ca(2+)-ATPase. Journal of Biological Chemistry. 2006;281:36597–36602. [Abstract] [Google Scholar]
- von Maltzahn J, Chang NC, Bentzinger CF, Rudnicki MA. Wnt signaling in myogenesis. Trends in Cell Biology. 2012;22:602–609. [Europe PMC free article] [Abstract] [Google Scholar]
- van Marken Lichtenbelt WD, Daanen HA. Cold-induced metabolism. Current Opinion in Clinical Nutrition and Metabolic Care. 2003;6:469–475. [Abstract] [Google Scholar]
- Marmonier F, Duchamp C, Cohen-Adad F, Eldershaw TP, Barre H. Hormonal control of thermogenesis in perfused muscle of Muscovy ducklings. American Journal of Physiology. 1997;273:R1638–R1648. [Abstract] [Google Scholar]
- Matthias A, Ohlson KB, Fredriksson JM, Jacobsson A, Nedergaard J, Cannon B. Thermogenic responses in brown fat cells are fully UCP1-dependent. UCP2 or UCP3 do not substitute for UCP1 in adrenergically or fatty scid-induced thermogenesis. Journal of Biological Chemistry. 2000;275:25073–25081. [Abstract] [Google Scholar]
- Mcnab BK. Evolution of endothermy in phylogeny of mammals. American Naturalist. 1978;112:1–21. [Google Scholar]
- de Meis L. Uncoupled ATPase activity and heat production by the sarcoplasmic reticulum Ca2+ —ATPase. Regulation by ADP. Journal of Biological Chemistry. 2001;276:25078–25087. [Abstract] [Google Scholar]
- de Meis L. Ca2+ —ATPases (SERCA): energy transduction and heat production in transport ATPases. Journal of Membrane Biology. 2002;188:1–9. [Abstract] [Google Scholar]
- de Meis L, Arruda AP, Carvalho DP. Role of sarco/endoplasmic reticulum Ca(2+)-ATPase in thermogenesis. Bioscience Reports. 2005;25:181–190. [Abstract] [Google Scholar]
- Meyer CW, Willershauser M, Jastroch M, Rourke BC, Fromme T, Oelkrug R, Heldmaier G, Klingenspor M. Adaptive thermogenesis and thermal conductance in wild-type and UCP1-KO mice. American Journal of Physiology. 2010;299:R1396–R1406. [Europe PMC free article] [Abstract] [Google Scholar]
- Mezentseva NV, Kumaratilake JS, Newman SA. The brown adipocyte differentiation pathway in birds: an evolutionary road not taken. BMC Biology. 2008;6:17. [Europe PMC free article] [Abstract] [Google Scholar]
- Miller WJ. Body temperature of adult pigeons. American Pigeon Journal. 1974 Jul;:397. [Google Scholar]
- Minaire Y, Chatonnet J. Influence of the muscular activities of shivering and work on metabolic kinetics of lactate and glucose. Journal of Physiology, Paris. 1968;60(Suppl 2):500–501. [Abstract] [Google Scholar]
- Mitchell JR, Jacobsson A, Kirchgessner TG, Schotz MC, Cannon B, Nedergaard J. Regulation of expression of the lipoprotein lipase gene in brown adipose tissue. American Journal of Physiology. 1992;263:E500–E506. [Abstract] [Google Scholar]
- Morrissette JM, Franck JP, Block BA. Characterization of ryanodine receptor and Ca2+ —ATPase isoforms in the thermogenic heater organ of blue marlin (Makaira nigricans) Journal of Experimental Biology. 2003;206:805–812. [Abstract] [Google Scholar]
- Muller MJ, Seitz HJ. Thyroid hormone action on intermediary metabolism. Part I: respiration, thermogenesis and carbohydrate metabolism. Klinische Wochenschrift. 1984;62:11–18. [Abstract] [Google Scholar]
- Nespolo RF, Bacigalupe LD, Figueroa CC, Koteja P, Opazo JC. Using new tools to solve an old problem: the evolution of endothermy in vertebrates. Trends in Ecology and Evolution. 2011;26:414–423. [Abstract] [Google Scholar]
- Newman SA. Thermogenesis, muscle hyperplasia, and the origin of birds. Bioessays. 2011;33:653–656. [Abstract] [Google Scholar]
- Newman SA, Mezentseva NV, Badyaev AV. Gene loss, thermogenesis, and the origin of birds. Annals of the New York Academy of Sciences. 2013;1289:36–47. [Abstract] [Google Scholar]
- Nikki P. Biogenic amines, cold-adaptation and halothane shivering in mice. Annales Medicinae Experimentalis et Biologiae Fenniae. 1969;47:129–140. [Abstract] [Google Scholar]
- O’Brien J, Block BA. Effects of Ca2+ on oxidative phosphorylation in mitochondria from the thermogenic organ of marlin. Journal of Experimental Biology. 1996;199:2679–2687. [Abstract] [Google Scholar]
- O’Brien J, Meissner G, Block BA. The fastest contracting muscles of nonmammalian vertebrates express only one isoform of the ryanodine receptor. Biophysical Journal. 1993;65:2418–2427. [Europe PMC free article] [Abstract] [Google Scholar]
- Oelkrug R, Goetze N, Exner C, Lee Y, Ganjam GK, Kutschke M, Muller S, Stohr S, Tschop MH, Crichton PG, Heldmaier G, Jastroch M, Meyer CW. Brown fat in a protoendothermic mammal fuels eutherian evolution. Nat Commun. 2013;4:2140. [Europe PMC free article] [Abstract] [Google Scholar]
- Ohno H, Shinoda K, Ohyama K, Sharp LZ, Kajimura S. EHMT1 controls brown adipose cell fate and thermogenesis through the PRDM16 complex. Nature. 2013;504:163–167. [Europe PMC free article] [Abstract] [Google Scholar]
- Periasamy M, Bhupathy P, Babu GJ. Regulation of sarcoplasmic reticulum Ca2+ ATPase pump expression and its relevance to cardiac muscle physiology and pathology. Cardiovascular Research. 2008;77:265–273. [Abstract] [Google Scholar]
- Periasamy M, Kalyanasundaram A. SERCA pump isoforms: their role in calcium transport and disease. Muscle & Nerve. 2007;35:430–442. [Abstract] [Google Scholar]
- Pontzer H, Allen V, Hutchinson JR. Biomechanics of running indicates endothermy in bipedal dinosaurs. PLoS ONE. 2009;4:e7783. [Europe PMC free article] [Abstract] [Google Scholar]
- Raimbault S, Dridi S, Denjean F, Lachuer J, Couplan E, Bouillaud F, Bordas A, Duchamp C, Taouis M, Ricquier D. An uncoupling protein homologue putatively involved in facultative muscle thermogenesis in birds. Biochemical Journal. 2001;353:441–444. [Europe PMC free article] [Abstract] [Google Scholar]
- Rolfe DF, Brown GC. Cellular energy utilization and molecular origin of standard metabolic rate in mammals. Physiological Reviews. 1997;77:731–758. [Abstract] [Google Scholar]
- Rose RW, Kuswanti N, Colquhoun EQ. Development of endothermy in a Tasmanian marsupial, Bettongia gaimardi and its response to cold and noradrenaline. Journal of Comparative Physiology B. 1998;168:359–363. [Abstract] [Google Scholar]
- Rose RW, West AK, Ye JM, McCormick GH, Colquhoun EQ. Nonshivering thermogenesis in a marsupial (the tasmanian bettong Bettongia gaimardi) is not attributable to brown adipose tissue. Physiological and Biochemical Zoology. 1999;72:699–704. [Abstract] [Google Scholar]
- Ruben J. The evolution of endothermy in mammals and birds: from physiology to fossils. Annual Review of Physiology. 1995;57:69–95. [Abstract] [Google Scholar]
- Runcie RM, Dewar H, Hawn DR, Frank LR, Dickson KA. Evidence for cranial endothermy in the opah (Lampris guttatus) Journal of Experimental Biology. 2009;212:461–470. [Europe PMC free article] [Abstract] [Google Scholar]
- Ruta M, Botha-Brink J, Mitchell SA, Benton MJ. The radiation of cynodonts and the ground plan of mammalian morphological diversity. Proceedings of the Royal Society B: Biological Sciences. 2013;280:20131865. [Europe PMC free article] [Abstract] [Google Scholar]
- Sahoo SK, Shaikh SA, Sopariwala DH, Bal NC, Periasamy M. Sarcolipin protein interaction with sarco(endo)plasmic reticulum Ca2+ ATPase (SERCA) is distinct from phospholamban protein, and only sarcolipin can promote uncoupling of the SERCA pump. Journal of Biological Chemistry. 2013;288:6881–6889. [Europe PMC free article] [Abstract] [Google Scholar]
- Saito S, Saito CT, Shingai R. Adaptive evolution of the uncoupling protein 1 gene contributed to the acquisition of novel nonshivering thermogenesis in ancestral eutherian mammals. Gene. 2008;408:37–44. [Abstract] [Google Scholar]
- Sanchez-Gurmaches J, Hung CM, Sparks CA, Tang Y, Li H, Guertin DA. PTEN loss in the Myf5 lineage redistributes body fat and reveals subsets of white adipocytes that arise from Myf5 precursors. Cell Metabolism. 2012;16:348–362. [Europe PMC free article] [Abstract] [Google Scholar]
- Schiaffino S, Reggiani C. Fiber types in mammalian skeletal muscles. Physiological Reviews. 2011;91:1447–1531. [Abstract] [Google Scholar]
- Schubring C. Temperature regulation in healthy and resuscitated newborns immediately after birth. Journal of Perinatal Medicine. 1986;14:27–33. [Abstract] [Google Scholar]
- Schulz TJ, Huang P, Huang TL, Xue R, McDougall LE, Townsend KL, Cypess AM, Mishina Y, Gussoni E, Tseng YH. Brown-fat paucity due to impaired BMP signalling induces compensatory browning of white fat. Nature. 2013;495:379–383. [Europe PMC free article] [Abstract] [Google Scholar]
- Schweitzer MH, Marshall CL. A molecular model for the evolution of endothermy in the theropod-bird lineage. Journal of Experimental Zoology. 2001;291:317–338. [Abstract] [Google Scholar]
- Seale P, Bjork B, Yang W, Kajimura S, Chin S, Kuang S, Scime A, Devarakonda S, Conroe HM, Erdjument-Bromage H, Tempst P, Rudnicki MA, Beier DR, Spiegelman BM. PRDM16 controls a brown fat/skeletal muscle switch. Nature. 2008;454:961–967. [Europe PMC free article] [Abstract] [Google Scholar]
- Seow CY, Ford LE. Shortening velocity and power output of skinned muscle-fibers from mammals having a 25,000-fold range of body-mass. Journal of General Physiology. 1991;97:541–560. [Europe PMC free article] [Abstract] [Google Scholar]
- Seymour RS. Maximal aerobic and anaerobic power generation in large crocodiles versus mammals: implications for dinosaur gigantothermy. PLoS ONE. 2013;8:e69361. [Europe PMC free article] [Abstract] [Google Scholar]
- Shabalina IG, Hoeks J, Kramarova TV, Schrauwen P, Cannon B, Nedergaard J. Cold tolerance of UCP1-ablated mice: a skeletal muscle mitochondria switch toward lipid oxidation with marked UCP3 up-regulation not associated with increased basal, fatty acid- or ROS-induced uncoupling or enhanced GDP effects. Biochimica et Biophysica Acta. 2010;1797:968–980. [Abstract] [Google Scholar]
- Shabalina IG, Kramarova TV, Nedergaard J, Cannon B. Carboxyatractyloside effects on brown-fat mitochondria imply that the adenine nucleotide translocator isoforms ANT1 and ANT2 may be responsible for basal and fatty-acid-induced uncoupling respectively. Biochemical Journal. 2006;399:405–414. [Europe PMC free article] [Abstract] [Google Scholar]
- Sidor CA, Hopson JA. Ghost lineages and “mammalness”: assessing the temporal pattern of character acquisition in the Synapsida. Paleobiology. 1998;24:254–273. [Google Scholar]
- Silva JE. Thermogenic mechanisms and their hormonal regulation. Physiological Reviews. 2006;86:435–464. [Abstract] [Google Scholar]
- Silva JE. Physiological importance and control of non-shivering facultative thermogenesis. Frontiers in Bioscience. 2011;3:352–371. [Abstract] [Google Scholar]
- Simonides WS, Thelen MH, van der Linden CG, Muller A, van Hardeveld C. Mechanism of thyroid-hormone regulated expression of the SERCA genes in skeletal muscle: implications for thermogenesis. Bioscience Reports. 2001;21:139–154. [Abstract] [Google Scholar]
- Slebodzinski AB. Hyperiodothyroninaemia of neonates, its significance for thermogenesis. Acta Physiologica Polonica. 1988;39:364–379. [Abstract] [Google Scholar]
- Slip DJ, Shine R. Reptilian endothermy – a field-study of thermoregulation by brooding diamond pythons. Journal of Zoology. 1988;216:367–378. [Google Scholar]
- Smith WS, Broadbridge R, East JM, Lee AG. Sarcolipin uncouples hydrolysis of ATP from accumulation of Ca2+ by the Ca2+ —ATPase of skeletal-muscle sarcoplasmic reticulum. Biochemical Journal. 2002;361:277–286. [Europe PMC free article] [Abstract] [Google Scholar]
- Stainsby WN, Lambert CR. Determination of oxygen uptake in skeletal muscle. Exercise and Sports Sciences Review. 1979;7:125–151. [Abstract] [Google Scholar]
- Stewart MA, Franks-Skiba K, Chen S, Cooke R. Myosin ATP turnover rate is a mechanism involved in thermogenesis in resting skeletal muscle fibers. Proceedings of the National Academy of Sciences of the United States of America. 2010;107:430–435. [Europe PMC free article] [Abstract] [Google Scholar]
- Strang KT, Steudel K. Explaining the scaling of transport costs – the role of stride frequency and stride length. Journal of Zoology. 1990;221:343–358. [Google Scholar]
- Tanche M, Therminarias A. Thyroxine and catecholamines during cold exposure in dogs. Federation Procedings. 1969;28:1257–1261. [Abstract] [Google Scholar]
- Teulier L, Rouanet JL, Letexier D, Romestaing C, Belouze M, Rey B, Duchamp C, Roussel D. Cold-acclimation-induced non-shivering thermogenesis in birds is associated with upregulation of avian UCP but not with innate uncoupling or altered ATP efficiency. Journal of Experimental Biology. 2010;213:2476–2482. [Abstract] [Google Scholar]
- Thomas SA, Palmiter RD. Thermoregulatory and metabolic phenotypes of mice lacking noradrenaline and adrenaline. Nature. 1997;387:94–97. [Abstract] [Google Scholar]
- Timmons JA, Wennmalm K, Larsson O, Walden TB, Lassmann T, Petrovic N, Hamilton DL, Gimeno RE, Wahlestedt C, Baar K, Nedergaard J, Cannon B. Myogenic gene expression signature establishes that brown and white adipocytes originate from distinct cell lineages. Proceedings of the National academy of Sciences of the United States of America. 2007;104:4401–4406. [Europe PMC free article] [Abstract] [Google Scholar]
- Tkachenko E. Shivering and non-shivering thermogenesis in skeletal muscles. Fiziologicheskii zhuenal imeni IM Sechenova. 1968;54:1475–1480. [Abstract] [Google Scholar]
- Torre-Bueno JR. Temperature regulation and heat dissipation during flight in birds. Journal of Experimental Biology. 1976;65:471–482. [Abstract] [Google Scholar]
- Toyomizu M, Ueda M, Sato S, Seki Y, Sato K, Akiba Y. Cold-induced mitochondrial uncoupling and expression of chicken UCP and ANT mRNA in chicken skeletal muscle. FEBS Letters. 2002;529:313–318. [Abstract] [Google Scholar]
- Toyoshima C, Cornelius F. New crystal structures of PII-type ATPases: excitement continues. Current Opinion in Structural Biology. 2013;23:507–514. [Abstract] [Google Scholar]
- Toyoshima C, Iwasawa S, Ogawa H, Hirata A, Tsueda J, Inesi G. Crystal structures of the calcium pump and sarcolipin in the Mg2+ —bound E1 state. Nature. 2013;495:260–264. [Abstract] [Google Scholar]
- Trayhurn P, Thomas ME, Keith JS. Postnatal development of uncoupling protein, uncoupling protein mRNA, and GLUT4 in adipose tissues of goats. American Journal of Physiology. 1993;265:R676–R682. [Abstract] [Google Scholar]
- Tseng YH, Cypess AM, Kahn CR. Cellular bioenergetics as a target for obesity therapy. Nature Reviews Drug Discovery. 2010;9:465–482. [Europe PMC free article] [Abstract] [Google Scholar]
- Tullis A, Block BA, Sidell BD. Activities of key metabolic enzymes in the heater organs of scombroid fishes. Journal of Experimental Biology. 1991;161:383–403. [Abstract] [Google Scholar]
- Tupling AR, Bombardier E, Gupta SC, Hussain D, Vigna C, Bloemberg D, Quadrilatero J, Trivieri MG, Babu GJ, Backx PH, Periasamy M, MacLennan DH, Gramolini AO. Enhanced Ca2+ transport and muscle relaxation in skeletal muscle from sarcolipin-null mice. American Journal of Physiology. 2011;301:C841–C849. [Europe PMC free article] [Abstract] [Google Scholar]
- Ukropec J, Anunciado RP, Ravussin Y, Hulver MW, Kozak LP. UCP1-independent thermogenesis in white adipose tissue of cold-acclimated Ucp1−/− mice. Journal of Biological Chemistry. 2006;281:31894–31908. [Abstract] [Google Scholar]
- Vangheluwe P, Schuermans M, Zador E, Waelkens E, Raeymaekers L, Wuytack F. Sarcolipin and phospholamban mRNA and protein expression in cardiac and skeletal muscle of different species. Biochemical Journal. 2005;389:151–159. [Europe PMC free article] [Abstract] [Google Scholar]
- Virtanen KA, Nuutila P. Brown adipose tissue in humans. Current Opinion in Lipidology. 2011;22:49–54. [Abstract] [Google Scholar]
- Vybiral S, Jansky L. Non-shivering thermogenesis in the golden hamster. Physiologia Bohemoslovaca. 1974;23:235–243. [Abstract] [Google Scholar]
- Walter I, Seebacher F. Molecular mechanisms underlying the development of endothermy in birds (Gallus gallus): a new role of PGC-1 alpha? American Journal of Physiology. 2007;293:R2315–R2322. [Abstract] [Google Scholar]
- Walter I, Seebacher F. Endothermy in birds: underlying molecular mechanisms. Journal of Experimental Biology. 2009;212:2328–2336. [Abstract] [Google Scholar]
- Winther AM, Bublitz M, Karlsen JL, Moller JV, Hansen JB, Nissen P, Buch-Pedersen MJ. The sarcolipin-bound calcium pump stabilizes calcium sites exposed to the cytoplasm. Nature. 2013;495:265–269. [Abstract] [Google Scholar]
- Wojciechowicz K, Gledhill K, Ambler CA, Manning CB, Jahoda CA. Development of the mouse dermal adipose layer occurs independently of subcutaneous adipose tissue and is marked by restricted early expression of FABP4. PLoS ONE. 2013;8:e59811. [Europe PMC free article] [Abstract] [Google Scholar]
- Wu J, Bostrom P, Sparks LM, Ye L, Choi JH, Giang AH, Khandekar M, Virtanen KA, Nuutila P, Schaart G, Huang K, Tu H, van Marken Lichtenbelt WD, Hoeks J, Enerback S, Schrauwen P, Spiegelman BM. Beige adipocytes are a distinct type of thermogenic fat cell in mouse and human. Cell. 2012;150:366–376. [Europe PMC free article] [Abstract] [Google Scholar]
- Wu J, Cohen P, Spiegelman BM. Adaptive thermogenesis in adipocytes: is beige the new brown? Genes & Development. 2013;27:234–250. [Europe PMC free article] [Abstract] [Google Scholar]
- Xue B, Rim JS, Hogan JC, Coulter AA, Koza RA, Kozak LP. Genetic variability affects the development of brown adipocytes in white fat but not in interscapular brown fat. Journal of Lipid Research. 2007;48:41–51. [Abstract] [Google Scholar]
- Ye JM, Edwards SJ, Rose RW, Steen JT, Clark MG, Colquhoun EQ. Alpha-adrenergic stimulation of thermogenesis in a rat kangaroo (Marsupialia, Bettongia gaimardi) American Journal of Physiology. 1996;271:R586–R592. [Abstract] [Google Scholar]
- Young P, Arch JR, Ashwell M. Brown adipose tissue in the parametrial fat pad of the mouse. FEBS Letters. 1984;167:10–14. [Abstract] [Google Scholar]
- Yu X, Inesi G. Variable stoichiometric efficiency of Ca2+ and Sr2+ transport by the sarcoplasmic reticulum ATPase. Journal of Biological Chemistry. 1995;270:4361–4367. [Abstract] [Google Scholar]
- Yuan B, Lu R, Gu Y, Liao Y, Wei H. Research progress of sarcolipin-a new regulatory protein of sarcoplasmic reticulum Ca2+ ATPase. Zhong Nan Da Xue Xue Bao Yi Xue Ban. 2012;37:316–319. [Abstract] [Google Scholar]
- Zancanaro C, Carnielli VP, Moretti C, Benati D, Gamba P. An ultrastructural study of brown adipose tissue in pre-term human new-borns. Tissue and Cell. 1995;27:339–348. [Abstract] [Google Scholar]
- Zurlo F, Larson K, Bogardus C, Ravussin E. Skeletal muscle metabolism is a major determinant of resting energy expenditure. Journal of Clinical Investigation. 1990;86:1423–1427. [Europe PMC free article] [Abstract] [Google Scholar]
Full text links
Read article at publisher's site: https://doi.org/10.1111/brv.12157
Read article for free, from open access legal sources, via Unpaywall:
https://europepmc.org/articles/pmc4854186?pdf=render
Citations & impact
Impact metrics
Citations of article over time
Alternative metrics
Smart citations by scite.ai
Explore citation contexts and check if this article has been
supported or disputed.
https://scite.ai/reports/10.1111/brv.12157
Article citations
Sleep deprivation stimulates adaptive thermogenesis by activating AMPK pathway in mice.
J Comp Physiol B, 30 Oct 2024
Cited by: 0 articles | PMID: 39477902
We need to talk-how muscle stem cells communicate.
Front Cell Dev Biol, 12:1378548, 10 Jul 2024
Cited by: 0 articles | PMID: 39050890 | PMCID: PMC11266305
Review Free full text in Europe PMC
On the origins of endothermy in amniotes.
iScience, 27(4):109375, 02 Mar 2024
Cited by: 0 articles | PMID: 38544566 | PMCID: PMC10966186
YTHDF2 governs muscle size through a targeted modulation of proteostasis.
Nat Commun, 15(1):2176, 11 Mar 2024
Cited by: 0 articles | PMID: 38467649 | PMCID: PMC10928198
Rapid adaptation of cellular metabolic rate to the MicroRNA complements of mammals and its relevance to the evolution of endothermy.
iScience, 27(2):108740, 21 Dec 2023
Cited by: 0 articles | PMID: 38327773 | PMCID: PMC10847693
Go to all (105) article citations
Data
Data behind the article
This data has been text mined from the article, or deposited into data resources.
BioStudies: supplemental material and supporting data
Nucleotide Sequences (2)
- (1 citation) ENA - AF227818
- (1 citation) ENA - BX935884
Similar Articles
To arrive at the top five similar articles we use a word-weighted algorithm to compare words from the Title and Abstract of each citation.
Avian adjustments to cold and non-shivering thermogenesis: whats, wheres and hows.
Biol Rev Camb Philos Soc, 97(6):2106-2126, 28 Jul 2022
Cited by: 3 articles | PMID: 35899483
Uncoupling of sarcoendoplasmic reticulum calcium ATPase pump activity by sarcolipin as the basis for muscle non-shivering thermogenesis.
Philos Trans R Soc Lond B Biol Sci, 375(1793):20190135, 13 Jan 2020
Cited by: 45 articles | PMID: 31928193 | PMCID: PMC7017432
Review Free full text in Europe PMC
Whole-body endothermy: ancient, homologous and widespread among the ancestors of mammals, birds and crocodylians.
Biol Rev Camb Philos Soc, 97(2):766-801, 10 Dec 2021
Cited by: 19 articles | PMID: 34894040 | PMCID: PMC9300183
Muscle Non-shivering Thermogenesis and Its Role in the Evolution of Endothermy.
Front Physiol, 8:889, 09 Nov 2017
Cited by: 61 articles | PMID: 29170642 | PMCID: PMC5684175
Review Free full text in Europe PMC