Abstract
Free full text

Genotype tunes pancreatic ductal adenocarcinoma tissue tension to induce matricellular-fibrosis and tumor progression
Abstract
Fibrosis compromises pancreatic ductal carcinoma (PDAC) treatment and contributes to patient mortality yet anti-stromal therapies are controversial. We found that human PDACs with impaired epithelial transforming growth factor β (TGF-β) signaling have elevated epithelial Stat3 activity and develop a stiffer, matricellular-enriched fibrosis associated with high epithelial tension and shorter patient survival. In several Kras-driven mouse models, both the loss of TGF-β signaling and elevated β1-integrin mechanosignaling engaged a positive feedback loop whereby Stat3 signaling promotes tumor progression by increasing matricellular fibrosis and tissue tension. In contrast, epithelial Stat3 ablation attenuated tumor progression by reducing the stromal stiffening and epithelial contractility induced by loss of TGF-β signaling. In PDAC patient biopsies, higher matricellular protein and activated Stat3 associated with SMAD4 mutation and shorter survival. The findings implicate epithelial tension and matricellular fibrosis in the aggressiveness of SMAD4 mutant pancreatic tumors, and highlight Stat3 and mechanics as key drivers of this phenotype.
Introduction
PDAC fibrosis compromises drug delivery, impedes immune cell accessibility and promotes disease aggression and therapy resistance1–4. To this end, inhibition of stromal sonic hedgehog (SHH) signaling in a mouse model significantly reduced fibrosis and increased drug update that, at least transiently, stabilized the disease5. Similarly, reducing the abundance of mouse tumor hyaluronan, using hyaluronidase, or treating xenografted human pancreatic tumors with an angiotensin inhibitor to reduce tissue tension, decreased facilitated chemotherapy delivery1,6. Yet, phase II clinical trials targeting fibrosis failed to increase patient survival7. Experiments in mouse models of PDAC revealed that, while depletion of proliferating α-smooth muscle actin (α-SMA) positive stromal cells reduced fibrosis, the vasculature remained abnormal and the tumors were hypoxic and less differentiated, resulting in accelerated mortality8. Additionally, despite a reduction in fibrosis and enhancement of tissue vascularity, genetic ablation of SHH or treatment with a smoothened inhibitor resulted in mouse PDACs that were less differentiated and more aggressive9.
At first glance, these data imply that the stroma can both promote and restrain tumor progression and suggest stromal dependency may be context dependent. Nevertheless, underlying assumptions informing these experimental and clinical manipulations were similarities in tissue across all PDACs regardless of tumor genotype, the mediation of stromal remodeling in PDACs by a uniform population of stromal fibroblasts, and a comparable physical impact of fibrosis including the restraint of the tumor cells, the impedance of tumor-associated vasculature and the prevention of immune infiltratation10–12. Yet the extent, nature and tumor cell response to the of tumor-associated fibrosis varies widely across cancers, tumor subtypes and even within the same tumor and the stromal population that contributes to ECM deposition and remodeling in tumors is heterogeneous13–16. Importantly, oncogenic transformation itself increases tumor cell tension that can critically contribute to ECM remodeling and the malignant phenotype as was demonstrated by a ras-transformed epithelium whose ROCK-dependent contractility and fibrosis induction was essential for Wnt-dependent squamous carcinoma progression17–19. Clearly sorting out the contribution of stromal and epithelial elements to PDAC fibrosis and pathology would go far to guide the development and application of anti-stromal therapies.
Patients whose tumors harbor Mothers Against DPP Homolog 4 (SMAD4) mutations die more frequently from disseminated disease20, and reduced levels of epithelial Smad4 enhanced the aggressiveness and increased dissemination of tumor cells from the PDACs that developed in an experimental mouse model21. Interestingly, experimental tumors with combined Kras mutations and Tgfbr2 deletion are highly fibrotic and exhibit a pronounced mesenchymal-like phenotype following stromal ablation8,22. Clinically, patient PDACs with a quasi-mesenchymal phenotype are more aggressive and treatment-resistant as compared to PDACs with a classical, more-differentiated histophenotype23,24. Moreover, the mesenchymal-like patient PDACs often associate with aberrant TGF-β signaling in the epithelium25. These findings suggest that PDACs in which TGF-β signaling is compromised may have a unique stromal-epithelial interaction phenotype that could influence their response to anti-stromal therapies. As such, we investigated the interplay between tumor genotype and fibrotic phenotype in PDAC progression.
Results
Tissue tension and collagen thickness linked to PDAC prognosis
Fibrillar collagen has been implicated in PDAC aggression and treatment resistance26, yet recent findings suggest collagen abundance in PDAC may associate with better, not worse, patient prognosis and with a more differentiated PDAC phenotype27–29. We constructed a gene list of major fibrillar collagens (Supplementary Table 1) and used this signature to stratify publically-available NCBI GEO data. We found no significant association between the levels of the major fibrillar collagens and patient survival. However, when we stratified patient data for high versus low Col1α2, Col2α1 and Col4α1 expression, patients expressing high levels of these specific collagens had a reduced overall survival (log rank p ≤ 0.04; Supplementary Fig. 1a).
To investigate the relationship between fibrillar collagen and PDAC phenotype, we assessed total and fibrillar collagen in PDAC tissue arrays with well (n = 19), moderately (n = 23) and poorly differentiated (n = 26) tumors. Although total and fibrillar collagens were similar in all tumor biopsies regardless of differentiation status (Fig. 1a;Supplementary Fig. 1b, c), we noted a reduction in the number of alpha smooth muscle actin α-SMA) positive cells in the poorly differentiated PDAC tumor biopsies, as compared to the well and moderately differentiated tumors (Fig. 1a; p ≤ 0.05)8,30. Moreover, the diameter of the collagen fibers adjacent to the epithelial lesions was significantly thicker in the poorly differentiated PDACs (Fig. 1; p ≤ 0.01–0.001). We also detected a progressive increase in level of phosphorylated myosin light chain 2 (pMLC2) in the epithelium that was adjacent to the thicker collagen fibers in the moderately and poorly differentiated tumors, consistent with increased epithelial actomyosin tension (Fig. 1a; p ≤ 0.05–0.001) suggesting an association may exist between tumor cell tension and the architecture of the adjacent collagen bundles19,31.
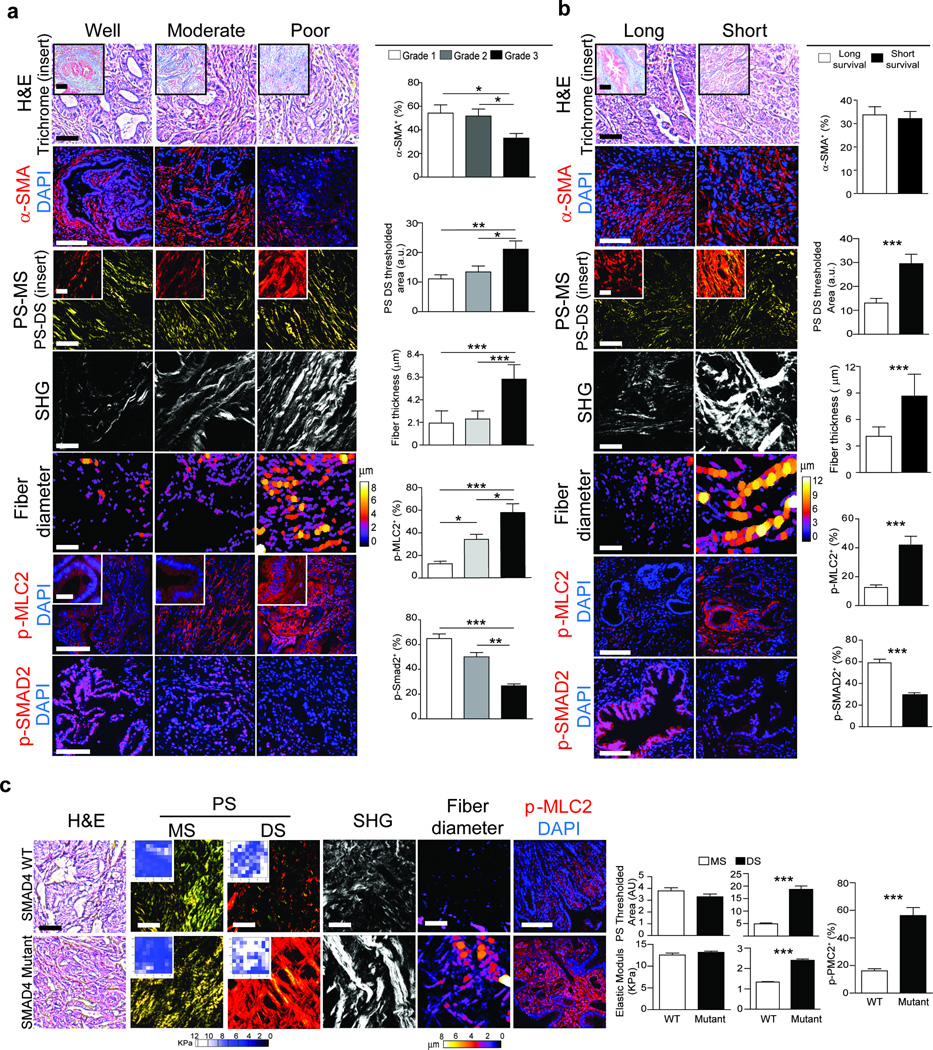
(a) H&E and Trichrome (top insert) staining of PDAC tissue arrays for well (n = 19), moderately (n = 23) or poorly differentiated (n = 26) tumors; Scale bar, 100 µm (main) and 50 µm (insert). Immunofluorescence images and quantifications of α-SMA, p-MLC2, p-SMAD2 and DAPI; Scale bar, 75 µm (main) and 40 µm (insert). Polarized light images of picrosirius red (PR) within the main stroma (MS) (3rd) and periductal stroma (DS) (3rd insert); Scale bar, 50 µm (main) and 40 µm (insert). Second harmonic generation (SHG) of periductal collagen and diameter color-coded SHG; Scale bar, 75 µm. (b) H&E and Trichrome (top insert) staining of tissue from PDAC patients with a median short survival of 11–289 days (n = 29) and median long survival of 1090–3298 days (n = 28); Scale bar, 100 µm. Immunofluorescence and quantification of tissue stained for α-SMA, p-MLC2, p-SMAD2 and DAPI; Scale bar, 75 µm. PR staining within the main stroma (MS) and periductal stroma (DS) (insert); Scale bar, 50µm. Periductal SHG and diameter color-coded SHG; Scale bar, 75 µm. (c) H&E staining of PDAC tissue from wild type SMAD4 (n = 10) and mutant SMAD4 (n = 10) patients. Scale bar, 100 µm. PR staining and quantification within the main stroma (MS) and periductal stroma (DS); Scale bar, 50 µm. Force maps and quantification of the main stroma (MS) (insert) and periductal stroma (DS) (insert). SHG of periductal collagen and diameter color-coded SHG; Scale bar, 75 µm. Immunofluorescence staining and quantification for p-MLC2 and DAPI; Scale bar, 75 µm. Results are presented as the mean +/− SEM. Subsequent statistical analysis was performed with either unpaired two-sided student t-tests, one-way ANOVA with Tukey’s method for multiple comparisons. (*P < 0.05; **P < 0.01, ***P < 0.001, ****P < 0.0001, “ns” not significant).
To investigate the relationship between fibrillar collagen, epithelial tension and patient survival, we stratified PDAC tumors from a cohort of patients32 by survival (median survival <10 months versus median survival >24 months). Again, we found no association between total and fibrillar collagen or α-SMA-positive cells to patient survival (Fig. 1b, Supplementary Fig. 1d,e). However, the diameter of the collagen fibers adjacent to the pancreatic lesions was significantly thicker in patients with the shortest survival (Fig. 1b; p ≤ 0.001). We also observed elevated pMLC2 in the PDAC tissues from patients with the shortest survival, indicating increased actomyosin tension and a relationship between the contractile PDAC epithelium and the reorganized collagen bundles (Fig. 1b; p ≤ 0.001).
TGF-β signaling is often attenuated in patient PDACs33, thus we assessed phosphorylation of SMAD2 as a primary mediator of canonical TGF-β signaling. The least differentiated PDAC lesions (Fig. 1a; p ≤ 0.001) and the PDACs derived from the patients with the shortest survival (Fig. 1b; p ≤ 0.001) had less detectable pSMAD2, suggesting they had compromised TGF-β signaling34,35. Next, we compared the stromal phenotype of patient PDACs with genetically altered SMAD4 to PDACs with wild type SMAD436. While total collagen and α-SMA levels were similar between both cohorts (Supplementary Fig. 1f), we observed elevated epithelial pMLC2 (Fig. 1c; p ≤ 0.001) with increased adjacent collagen fibers in PDACs lacking functional SMAD4 (Fig. 1c; p ≤ 0.001). Atomic Force Microscopy (AFM) mechanical testing revealed that the thickened, epithelial-adjacent collagen fibers were stiffer in the PDACs from patients whose lesions lacked a functional SMAD4 (Fig. 1c; p ≤ 0.001). These findings indicate that the architecture and mechanics of collagen fibers adjacent to the epithelial lesion, and not bulk collagen abundance, may be an indicator of PDAC aggression and that a reduction in TGF-β signaling in the PDAC epithelium may influence the nature of the stromal response.
PDAC genotype tunes epithelial tension to regulate fibrosis
To explore the relationship between TGF-β signaling, collagen organization and tissue mechanics, we exploited available PDAC genetically-engineered mouse models (GEMMs). By 20 weeks KC (KrasLSL-G12D/+Ptf1α-Cre)37 mice had progressed to PanIN lesions, whereas the KPC (KC with mutant Tp53+/−; KrasLSL-G12D/+Tp53R172H/+Pdx1-Cre) and KTC (KC with Tgfbr2flox/wt; KrasLSL-G12D/+Tgfbr2flox/wtPtf1a-Cre) mice developed PDAC lesions22,38. Coincident with tumor formation, the pancreatic tissues of both the KPC and KTC mice were highly fibrotic, indicated by abundant quantities of total and fibrillar collagen (not shown). Targeted proteomics confirmed that many of the fibrillar collagens in the KTC and KPC mice were present at similar levels (Supplementary Table 2). Immunofluorescence staining showed that KTC and KPC tumors had similar stromal levels of collagen III, α-Sma and fibroblast activated protein (Fap) (Supplementary Fig. 2a). GLI family zinc finger 1 (Gli1), which regulates PDAC fibrosis, was abundantly and uniformly expressed in the KPC and KTC tumor stroma (Supplementary Fig. 2a). Nevertheless, and in agreement with our clinical findings, KTC PDAC lesions had thicker collagen bundles and a stiffer ECM in the periductal region (Fig. 2a–c; p ≤ 0.001). Mass spectrometry and immunofluorescence analyses of the tumor ECM further revealed that the altered fibrillar collagen phenotype and the elevated ECM stiffness in the KTC PDAC lesions was accompanied by a significant increase in tenascin C, fibronectin and collagen, type XII, alpha 1 (Supplementary Fig. 3b, p ≤ 0.05; Table 2; Fig 2a–b, p ≤ 0.01–0.001). The KTC PDAC epithelium was also more contractile as indicated by more pMLC2 and myosin phosphatase target subunit 1 (p-MyPT1; Fig 2a, Supplementary Fig 3a; p ≤ 0.01–0,001) and higher mechanosignaling indicated by activated β1-integrin, phosphorylated focal adhesion kinase (p397-Ptk2; Fig. 2a, Supplementary Fig. 3a; p ≤ 0.05–0.01) and nuclear Yorkie activated protein (YAP1; Fig. 2a, Supplementary Fig. 3a; p ≤ 0.01–0.001;39). These data suggest that a reduction in TGF-β signaling increases actomyosin tension and mechanosignaling in a transformed pancreatic epithelium to induce a stiffer, periductal, matricellular-enriched fibrosis.
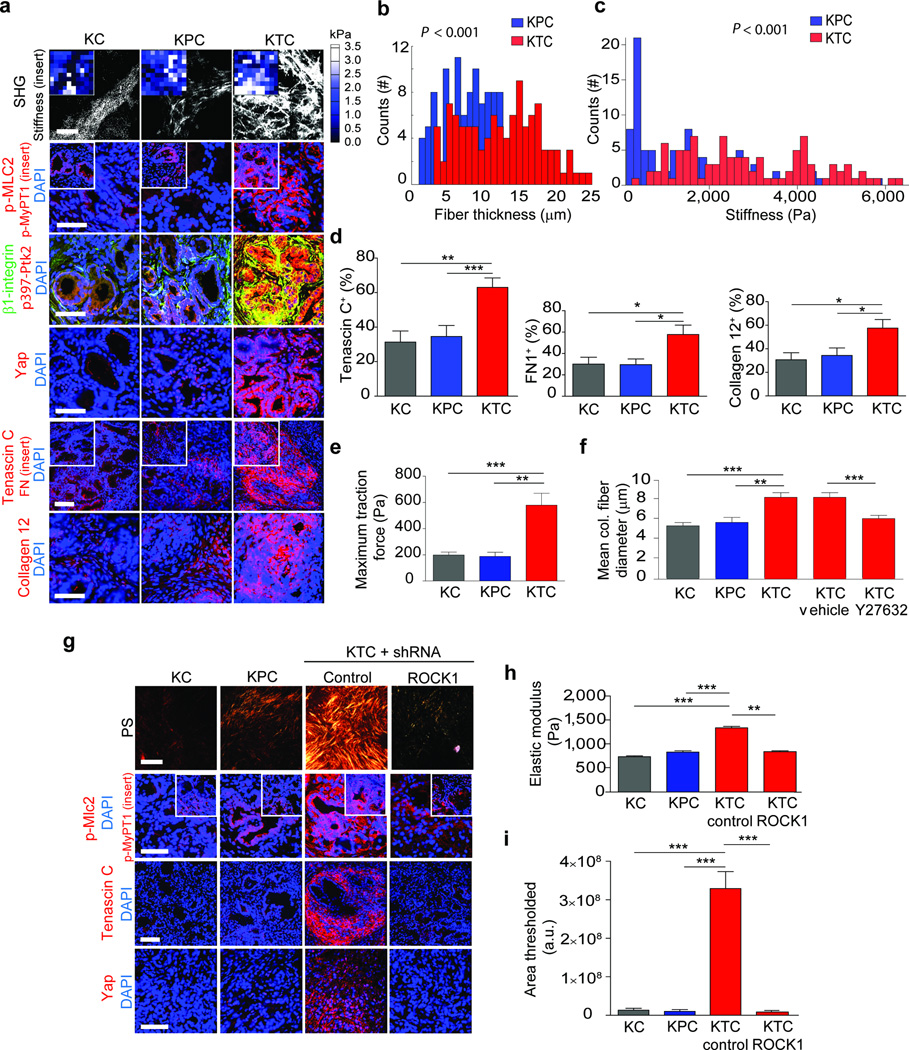
(a) SHG images from 20 week KC, KPC or KTC transgenic pancreatic tissues (top); Scale bar, 75 µm. Force maps from AFM PDAC ECM (top insert). Immunofluorescence images of p-Mlc2, p-MyPT1 (insert), β1-integrin and p-Ptk2, Yap1 and DAPI; Scale bar, 50 µm. Tenascin C, Fibronectin (insert), Collagen type XII alpha1 and DAPI; Scale bar, 75 µm. (b) Quantification of SHG fibril thickness and distribution around PDAC lesions. (c) Distribution of PDAC ECM stiffness measured by AFM. (d) Quantification of Tenascin C, Fibronectin and Collagen type XII alpha 1 images shown in (a). (e) Quantification of traction force KC, KPC and KTC cells on 2300 Pa polyacrylamide gels. (f) Quantification of mean collagen fiber diameter in three-dimensional collagen gels with KC, KPC or KTC cells or with KTC cells treated with vehicle or ROCK inhibitor Y27632 at 24 hours. (g) PR staining of tissue excised from nude mice 3 weeks after injection with KC, KPC, or KTC cells expressing either a control shRNA or an shRNA to Rock1 (top); Scale bar, 75 µm. Immunofluorescence of p-Mlc2, p-MyPT1 (insert), Tenascin C, Yap1 and DAPI; Scale bar, 50 µm. (h) Quantification of stiffness of tissue in (e). (i) Quantification of total levels of fibrillar collagen. For in vitro bar graphs, 3 technical replicates were performed and results are the mean +/− SEM of 3 independent experiments. For in vivo experiments, n = 5 mice per group. Subsequent statistical analysis was performed with either unpaired two-sided student t-tests, one-way ANOVA with Tukey’s method for multiple comparisons. For survival analysis, the log-rank (Mantel-Cox) test was used. (*P < 0.05; **P < 0.01, ***P < 0.001, ****P < 0.0001, “ns” not significant).
We next assessed the contractility phenotype of individual PDAC epithelial cells derived from KC, KTC and KPC mice using traction force microscopy (TFM). KTC tumor cells were more than the KPC or KC cells (Supplementary Fig. 3c). The KTC tumor cells promoted more Rho kinase 1 (Rock1) and not Rock 2-dependent 3D collagen gel contraction than KPC or KC mouse tumor cancer cells (Supplementary Fig. 4a; p ≤ 0.01–0.001). (Fig. 2d; p ≤ 0.01–0.001; Supplementary Fig. 5a–d). The KTC tumor cells exhibited higher Rock1 dependent tumor growth (Fig. 2g, Supplementary Fig. 4d). Myosin tension, as revealed by elevated traction force as well as pMLC2 (Fig 2e, Supplementary Fig. 4c), induced more nuclear Yap1 and YAP activity as indicated by higher Ctgf (Fig. 2e, Supplementary Fig. 4c) and promoted a greater fiber diameter (Fig. 2f; p ≤ 0.001) that was stiffer and enriched for the matricellular protein tenascin C (Fig. 2g–i; p ≤ 0.01–0.001) upon orthotopic injection into the pancreas of immune-compromised mice. These data demonstrate that a reduction of TGF-β signaling in the pancreatic epithelium increases tumor cell tension to induce a stiffer, matricellular fibrosis.
KTC tumor cells also showed a significant increase in anchorage-independent growth and colony formation (Supplementary Fig. 4f; p ≤ 0.05–0.001) which is consistent with a reduced dependence on ECM ligation for tumor cell survival and the enhanced metastasis incidence observed in patients with mutant SMAD440,41. However, the size of the KTC tumors was significantly smaller than those formed by KPC cells (Supplementary Fig. 4d–e). Thus, although pancreatic transformation is universally accompanied by a progressive fibrosis and stiffening of the ECM, the nature of the fibrotic response and the mechano-phenotype of the cancer can be modified by the genotype of the tumor.
JAK-Stat3 signaling drives ECM remodeling and stiffening
We next sought to clarify how reduced TGF-β signaling could increase tumor cell tension. G protein coupled receptor (GPCR)-mediated Janus kinase (Jak) activation stimulates activation of both signal transducer and activator of transcription 3 (Stat3) and ROCK, and induces actomyosin-mediated cell contractility and Stat3 has been implicated in PDACs42,43–46. We noted that pStat3 staining was significantly higher in the pancreatic epithelium of the KTC mice compared to KPC or KC mice (Fig. 3a; p ≤ 0.001), even in the eight week old PanINs where the amount of infiltrating immune cells is quite low (Supplementary Fig. 6a; p ≤ 0.001). Although both the stroma and the PDACs in the KPC and KTC mice stained positively for pStat3, coincident with an abundant immune infiltrate46, pStat3 was significantly higher only in the tumor pancreatic epithelium in KTC mice (Fig. 3a; p ≤ 0.001). We also detected abundant pStat3 in vitro in non-stimulated KTC, but not KPC or KC, cells (Fig. 3b, Supplementary Fig 5a). KTC tumor cell contraction and remodeling of collagen gels was blocked by treatment with the JAK inhibitor Ruxolitinib (Fig. 3c,d, Supplementary Fig. 6b). We also noted that the 48 hour conditioned media from KTC tumor cells activated pStat3 in cultured KC cells (Fig. 3e), and simultaneously increased the JAK-dependent ability of the KC cells to contract and remodel collagen gels (Fig. 3g; p ≤ 0.0.001–05; Supplementary Fig. 6c). A stiff ECM can enhance Stat3 activation47, and we observed higher steady state pStat3 levels in KC tumor cells and higher levels of secreted cytokines in the media of Kras pancreatic tumor cells when they were plated on a stiff ECM (Fig. 3f,i, p ≤ 0.01; Fig. 3g, p ≤ 0.05–0.001; Supplementary Fig. 6d–f). These findings identify an epithelial Jak-Rock-Stat3 signaling circuit as a candidate KTC tumor cell-specific contractility mechanism that could induce ECM remodeling and foster the development of a matricellular-enriched pancreatic fibrosis.
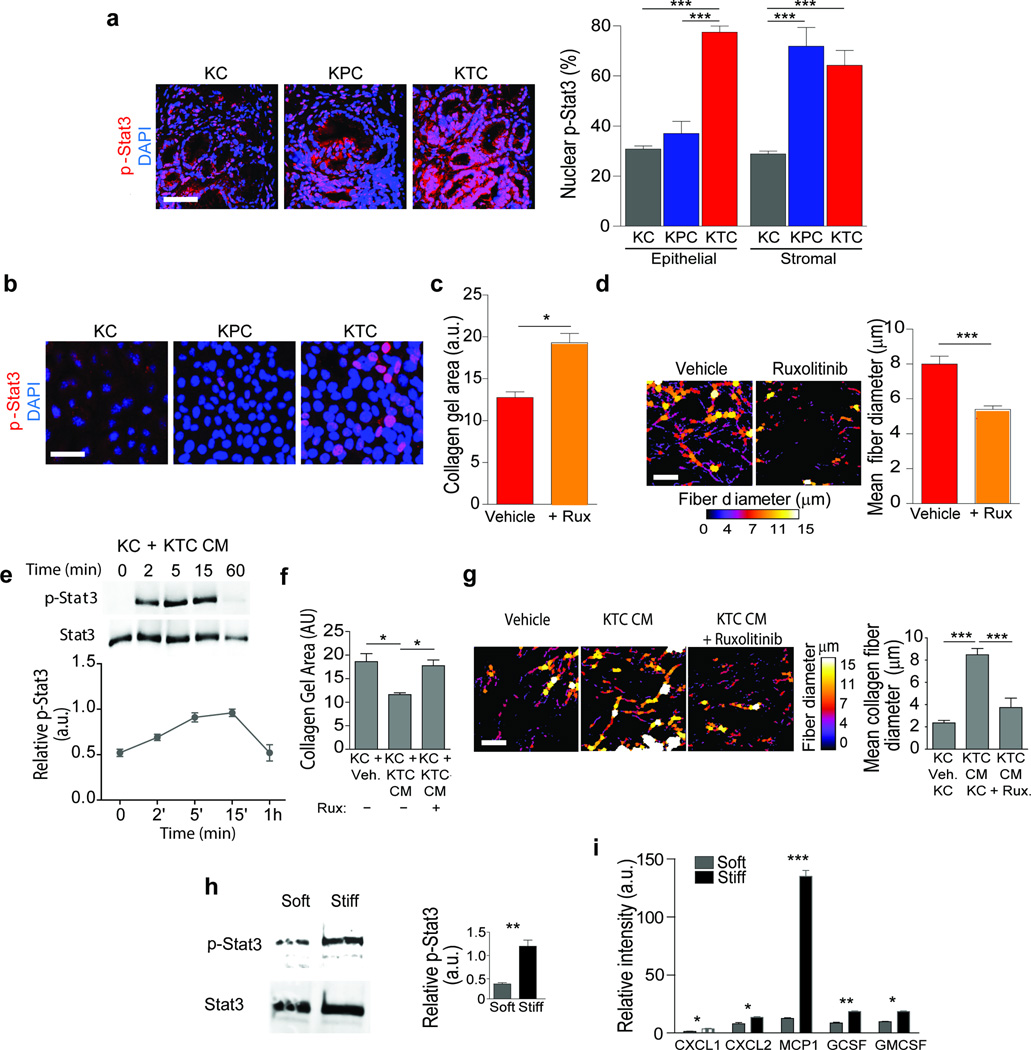
(a) Immunofluorescence images and quantification of epithelial and stromal staining in 20 week old KC, KPC and KTC mice stained for pStat3 and DAPI; Scale bar, 50 µm. (b) Immunofluorescence images of KC, KPC and KTC tumor cells stained for p-Stat3 and DAPI; Scale bar, 25 µm. (c) Quantification of collagen contraction as measured by total collagen gel area in three dimensional collagen gels with KTC tumor cells for 24 hours either with vehicle or the Jak inhibitor Ruxolitinib. (d) Polarized light images and quantification of PR stained color-coded fibrillar collagen diameter on gels from (c); Scale bar 75 µm. (e) Immunoblot and relative quantification of Stat3 activation (pStat3) in KC cells following exposure to KTC conditioned media (CM). (f) Quantification of collagen contraction measured by total collagen gel area of gels with KTC vehicle or Ruxolitinib CM treated KC tumor cells for 48 hours. (g) Polarized light images and quantification of PS, color-coded fibrillar collagen diameter; Scale bar 75 µm. (h) Immunoblot and relative quantification of total Stat3 and p-Stat3 levels in KC tumor cells cultured on soft or stiff polyacrylamide substrates. (i) Quantification of cytokine levels in the CM of KC cells cultured on soft or stiff polyacrylamide substrates. For in vitro quantification, results represent 3 technical replicates for and are the mean +/− SEM of 3 independent experiments. For in vivo experiments, n = 5 mice per group. Subsequent statistical analysis was performed with either unpaired two-sided student t-tests, one-way ANOVA with Tukey’s method for multiple comparisons. For survival analysis, the log-rank (Mantel-Cox) test was used. (*P < 0.05; **P < 0.01, ***P < 0.001, ****P < 0.0001, “ns” not significant).
Tumor cell tension accelerates PDAC progression in mice
To test links between pStat3, tumor tension and fibrosis in PDAC progression we crossed transgenic mice expressing Ptf1α-Cre with mice expressing a conditional V737N β1-integrin (Supplementary Fig. 7a), which recapitulates tension-dependent integrin clustering and promotes focal adhesion signaling and ROCK-dependent cell contractility in the pancreatic epithelium18,48,49. Immunofluorescence staining for confirmed elevated Ptk2 activity (p≤0.001) in the pancreatic epithelium in the β1–V737N mice, and revealed higher epithelial contractility as indicated by increased pMlc2 (p ≤ 0.001) and higher mechanosignaling as indicated by nuclear localization of Yap targets including Ctgf (p ≤ 0.05; Supplementary Fig. 7b–c). Evidence of fibrosis was indicated by increased picrosirius red (PR) staining (Supplementary Fig. 7b) and second harmonic generation (SHG) revealed thicker, denser collagens (not shown); AFM mechanical testing showed a significant stiffening of the ECM surrounding the ductal epithelium (Supplementary Fig. 7b). The presence of pStat3-positive pancreatic epithelial cells in the β1-V737N mice (p ≤ 0.05), even in the absence of any activating oncogene or pre-existing inflammation, confirmed that epithelial tension can directly enhance Stat3 activation, altered cytokine expression, and immune cell infiltration (Supplementary Fig. 7b–e). Consistently, treatment of the β1-V737N mice with a FAK inhibitor reduced pStat3, p397FAK, and pMLC2 abundance as well as the matricellular-enriched fibrosis (Supplementary Fig. 8a). Orthotopic injection of KTC cells expressing an shRNA against FAK (Supplementary Fig. 9a,b) into immunocompromised mice also reduced fibrosis (p ≤ 0.001), decreased stiffness (p ≤ 0.01) and lowered tenascin C levels (p ≤ 0.001) towards that of control tumors. The pancreatic tumor epithelium expressing the Ptk2 shRNA was less mechanically-activated, as indicated by reduced nuclear Yap1 (p ≤ 0.001), and had reduced levels of nuclear pStat3 (p ≤ 0.001; Fig. 4e), than control tumors.

(a) Cartoon of mouse manipulations used to study the impact of increasing pancreatic epithelial cell mechanosignaling using β1-V737N on Kras-induced pancreatic malignancy. (b) Immunofluorescence images and quantification of pancreatic tissues from 3 month old KC and KC/β1-V737N mice stained for p397-Ptk2 and p-Mlc2, Tenascin C, Yap1, p-Stat3; Scale bar, 50 µm, CD68 and DAPI; Scale bar, 100 µm. Polarized light images of PS staining; Scale bar, 75 µm. Force maps of ECM stiffness. (c) Alcian blue H&E images of KC and KC/β1-V737N tissue; Scale bar, 100µm. (d) Quantification of histopathologic phenotypes present in KC and KC+β1-V737N mice. (e) Polarized light images and quantification of PS staining of pancreatic tissues in nude mice injected with KTC tumor cells expressing either a control shRNA or a FAK shRNA; Scale bar, 75 µm. Immunofluorescence images and quantification of Tenascin C (scale bar 75 µm), Yap1 (75 µm), p-Stat3 (scale bar, 25 µm) and DAPI. (f) Quantification of average elastic modulus (Pa). For in vivo experiments, n = 5 mice per group. Subsequent statistical analysis was performed with unpaired two-sided student t-tests.. (*P < 0.05; **P < 0.01, ***P < 0.001, ****P < 0.0001, “ns” not significant).
To assess the relationship between epithelial tension and PDAC development, we bred Ptf1α-Cre V737N β1-integrin mice with KC mice (Fig. 4a) and found that, at 5 weeks of age, the pancreatic epithelium in the KC mice expressing the β1-V737N transgene had significantly higher p397Ptk2 (p ≤ 0.001) and pMlc2 (p ≤ 0.01). The amount and distribution of collagen fibers and ECM stiffness were highest in the periductal region (Fig. 4b; p ≤ 0.001), similar to what we observed in the developing KTC lesions (compare SHG in Fig. 2a to PS in Fig. 4b). Consistent with the matricellular-enriched fibrosis observed in both the patient samples lacking pSmad and the PDAC lesions in the KTC mice, we detected abundant tenascin C and higher levels of epithelial pStat3 in the KC/β1-V737N tumors (Fig. 4b; p ≤ 0.001). This phenotype was accompanied by an increase in infiltrating CD68 macrophages (Fig. 4b; p ≤ 0.05), and FACS characterization of the immune cell population revealed an increase in CD45+/CD11b myeloid cells and CD45+/Ly6C monocytes (Supplementary Fig. 9d). Indeed, cytokine array analysis demonstrated that the KC/β1-V737N mouse pancreas expressed higher levels of pro-inflammatory factors C5α and IL1 (Supplementary Fig. 9e). KC/β1-V737N mice developed chronic pancreatitis as early as 3 months of age (Fig. 4c). Alcian blue staining of the 3 month old KC/V737N mouse pancreatic tissue revealed that the lesions had progressed to advanced, high grade PanINs and that these foci were evenly distributed throughout the tissue. 38% of the KC/β1-V737N animals developed frank PDAC by 5-8 months of age, with accompanying physiological trauma, including loss of body weight (Fig. 4d, Supplementary Fig. 9c). These results reveal that increasing the cytoskeletal contractility in pancreatic tumor cells drives remodeling and stiffening of the periductal ECM, induces a matricellular-enriched fibrosis and promotes pancreatic transformation.
Stat3 induces fibrosis and accelerates PDAC
We next explored the relationship between Stat3 activation, cell contractility and a pancreatic matricellular-enriched fibrosis in PDAC. We crossed KC mice to mice expressing one allele of a constitutively-active Stat350 (Stat3C, Fig. 5a). Stat3C mice contained significantly more cells within the pancreas that stained positively for activated Stat3 (Supplementary Fig. 11a; p ≤ 0.001) and the level of pStat3 was further increased after expression of activated Kras (Fig. 5b; p ≤ 0.001). We quantified higher numbers of CD45+ immune cells (p≤0.001) and CD68+ macrophages (p ≤ 0.05) within the Stat3C pancreatic tissues (Supplementary Fig. 11a); these immune infiltrate further increased with Kras activation (Fig. 5b; p ≤ 0.05–0.001). Indeed, the pancreatic tissues of the Stat3C/Kras mice showed marked pancreatitis that developed into frank PDAC, and shortened survival compared to Kras mice (Fig. 5c). Consistent with a link between Stat3 and ROCK, although tumors were stiffer and pMLC2 was abundant in the KC/Stat3C mice, pMLC2 levels were already elevated significantly (p ≤ 0.001) in the pancreatic tissues of the Stat3C mice, even in the absence of Kras (Fig. 5b, 5d, Supplementary Fig. 11a). The pancreas of the Stat3C mice contained abundant mature type I collagen (Supplementary Fig. 11a; p ≤ 0.005) and elevated p397Ptk2 throughout (Supplementary Fig. 11a; p≤0.05). These findings link Stat3 activation and tissue inflammation, and suggest that pStat3 induces ROCK-dependent ECM remodeling and stiffening to drive fibrosis and elevate mechanosignaling in PDACs.

(a) Cartoon depicting the mouse crosses used for the activated Stat3C manipulations. (b) Immunofluorescence images and quantification of pancreatic tissues from 5 week old KC and KC/Stat3C mice stained for p-Stat3, scale bar, 75 µm, p397-Ptk2 and p-Myl2, scale bar, 50 µm, CD68, scale bar, 100 µm, and DAPI. Polarized light images and quantification of PS staining; Scale bar, 75 µm and H&E staining; Scale bar, 100 µm. (c) Kaplan-Meier graph showing survival of KC and KC/Stat3C mice. (d) Quantification of ECM stiffness in (b). (e) Cartoon depicting the mouse crosses used for the Stat3 knock studies. (f) Immunofluorescence images and quantification of pancreatic tissues from 5 week old homozygous KTC (Control) and KTC+Stat3−/− mice (KTC Stat3−/−) stained for p-Stat3, scale bar, 75 µm, p397-Ptk2 and p-Myl2, scale bar, 50 µm, Tenascin C, CD68, scale bar, 100 µm, Yap1 scale bar, 50 µm, and DAPI. Polarized light images and quantification of PS staining; Scale bar, 75 µm, and H&E staining. Scale bar, 100 µm. (g) Kaplan-Meier graph showing survival of KTC and KTC/Stat3 KO mice. (h) Quantification of ECM stiffness on tissue in (f). For in vivo experiments, n = 5 mice per group. Subsequent statistical analysis was performed with unpaired two-sided student t-tests. (*P < 0.05; **P < 0.01, ***P < 0.001, ****P < 0.0001, “ns” not significant).
To establish a causal relationship between Stat3 activation, tissue mechanics and a matricellular-enriched fibrosis and PDAC development, we crossed mice expressing Kras and homozygous for loss of both Tgfbr2 alleles21 (KTC KO; KrasLSL-G12D/+Tgfbr2flox/flox), which exhibit an accelerated matricellular-enriched fibrotic phenotype and PDAC development (Supplementary Fig. 10a–c) and mice expressing a conditional Stat3 knockout51 in the pancreatic epithelium (Stat3 KO; Stat3flox/floxPtf1α-Cre) to create KTC KO/Stat3 KO mice (Fig. 5e). Immunostaining confirmed deletion of Stat3 in the pancreatic epithelium (Fig. 5f; p ≤ 0.001) accompanied by significantly reduced CD68+ cell tissue infiltration (p ≤ 0.001), consistent with decreased inflammation (Fig. 5f). Loss of epithelial Stat3 was also accompanied by a significant reduction in epithelial p397Ptk2, pMlc2 and nuclear Yap1 levels (p ≤ 0.001) as well as Yap transcriptional activity as indicated by significantly lower Ctgf expression (Fig. 5f, Supplementary Fig. 9b; p ≤ 0.05). Also, pancreatic tissues with deleted epithelial Stat3 showed a significant reduction in a matricellular-rich fibrosis and ECM stiffness (p ≤ 0.001), along with a significant increase in mouse survival (Fig. 5f–h).
Direct links between a matricellular-rich fibrotic phenotype, mechanics and pStat3 were confirmed in a cohort of KTC mice treated with the JAK inhibitor Ruxolitinib. Ruxolitinib treatment inhibited pStat3 activity and significantly reduced p397Ptk2, pMlc2, collagen fibrillogenesis, matricellular matrix enrichment and ECM stiffening (Supplementary Fig. 11c–d; p ≤ 0.01–0.001) as well as Yap activation (not shown). Moreover, in the absence of JAK-driven matricellular-rich fibrosis, mechanosignaling, Stat3-dependent inflammation and PDAC development were significantly reduced (Supplementary Fig. 11c, 11e; p ≤ 0.01–0.001).
We investigated the clinical relevance of STAT3 activation in matricellular-enriched fibrosis and PDAC progression, and found elevated levels of tenascin C, fibronectin and collagen 12 (p ≤ 0.05), high nuclear YAP1 (p ≤ 0.05) (Fig. 6a) and elevated SOX2, vimentin (p ≤ 0.01; Supplementary Fig. 12a) and pSTAT3 (Fig. 6a; p ≤ 0.05) in the mesenchymal-like, vimentin-positive PDAC biopsies excised from patients with shorter median survival. The PDAC tissue with confirmed SMAD4 mutations also had elevated levels of epithelial pSTAT3 (p ≤ 0.001), tenascin C (p ≤ 0.001), fibronectin (p ≤ 0.01) and collagen 12 (p ≤ 0.05) (Fig. 6b). The epithelium in the PDAC tumors with mutated SMAD4 was more mechanically activated as evidenced by a significant increase in nuclear YAP1 (p ≤ 0.01). Moreover, the samples with SMAD4 mutations had significantly higher nuclear SOX2 (p ≤ 0.01) and contained significantly more vimentin (p ≤ 0.001) which is consistent with a quasi-mesenchymal phenotype that typically has a more aggressive disease pathology (Supplementary Fig. 12b).
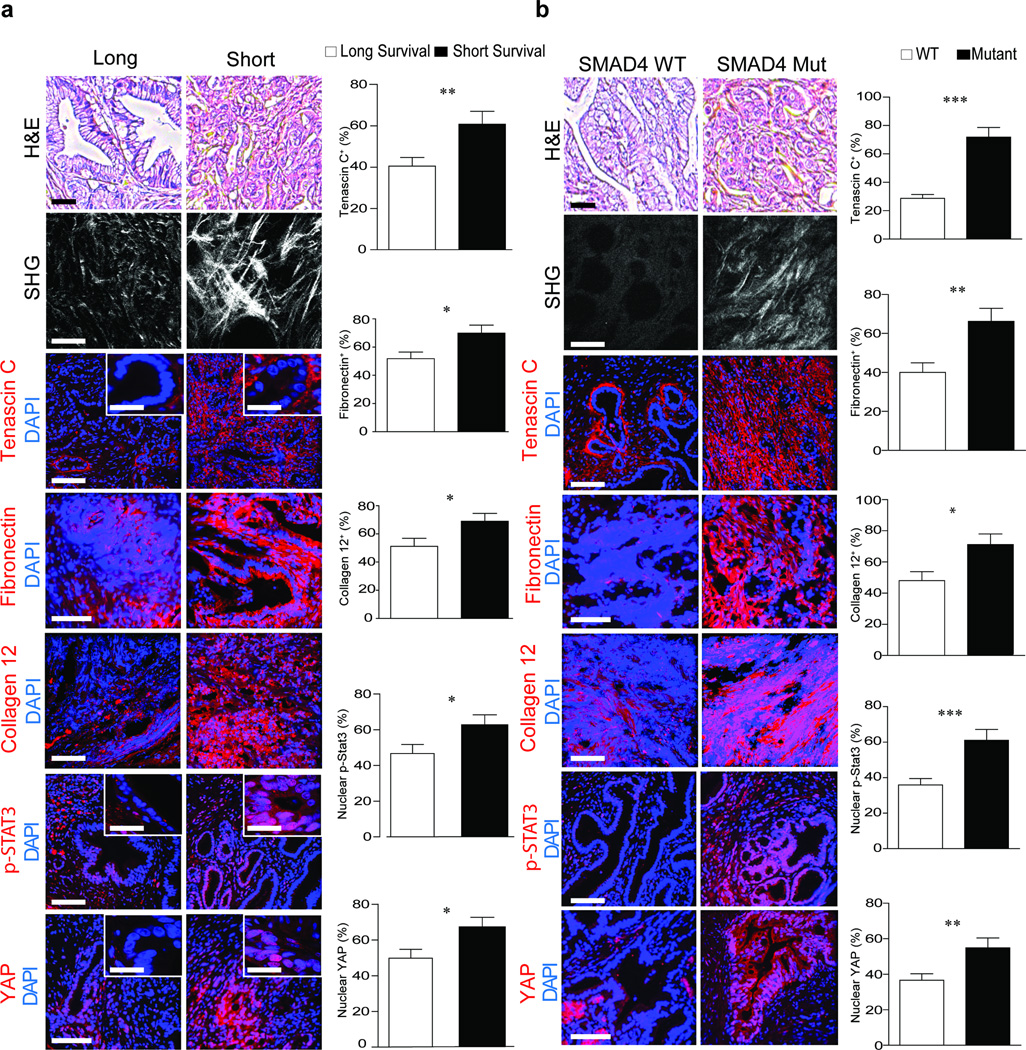
(a) H&E images of PDAC tumors from patients with a median short survival of 11–289 days (n = 29) and median long survival of 1090–3298 days (n = 28). Scale bar, 100 µm. SHG images and quantification of extracellular collagen architecture in the pancreatic tissue around the epithelial ductal region in tissue described above. Scale bar, 75 µm (main). Immunofluorescence images and quantification of Tenascin C, Fibronectin, Collagen XII alpha1, p-STAT3, YAP1 and DAPI. Scale bar, 75 µm and 10 µm (insert). (b) H&E images of PDAC tumors from patients with WT SMAD4 (n = 10) or mutant SMAD4 (n = 10); Scale bar, 100 µm. SHG images and quantification of periductal collagen. Scale bar, 75 µm. Immunofluorescence images and quantification of Tenascin C, Fibronectin, Collagen XII alpha1, p-STAT3, YAP1 and DAPI. Scale bar, 75 µm. Results are presented as the mean +/− SEM. Subsequent statistical analysis was performed with unpaired two-sided student t-tests. (*P < 0.05; **P < 0.01, ***P < 0.001, ****P < 0.0001, “ns” not significant).
Discussion
Using clinical specimens and experimental mouse models, we identified a unique, highly rigid, matricellular-stromal phenotype linked specifically to a PDAC genotype that results in reduced epithelial TGF-β signaling and elevated tumor cell contractility. Our data suggest that the genetically-induced tumor cell tension differentially "tunes" the composition and mechanics of the periductal stroma in PDACs, regardless of the bulk stromal phenotype, to promote tumor progression and aggression by engaging mechanosignaling pathways linked to integrins and YAP. The findings are consistent with the mathematical predictions identifying two distinct stromal phenotypes termed "normal" versus "activated" in PDAC subtypes of increasing aggression24. Our findings provide the first direct evidence that tumor genotype, per se, can calibrate tumor cell contractility to modulate the fibrotic phenotype of the tissue and modify the pathology of the cancer. Our data further suggest that bulk collagen content is a poor surrogate for the multi-faceted contributions of PDAC fibrosis to cancer aggression, and advocate imaging approaches to visualize differential distribution of the stroma such as MR imaging and elastography52.
PDAC-associated fibrosis has been viewed as a constraint that directly compromises lymphatic drainage and impedes neovascularization, and indirectly increases interstitial fluid pressure to disrupt blood vessel integrity and induce hypoxia1. This physical barrier compromises drug delivery, reduces immune cell accessibility and promotes disease aggression and therapy resistance, underscoring the critical importance of tissue mechanics to tumor biology12–4,53. Importantly, our data show that the fibrosis is not merely a passive physical restraint. Rather, the elevated tissue mechanics directly activate key pathways such as integrin-dependent signaling and YAP to promote tumor progression and aggression10–12. To this end, our clinical findings revealed that poorly differentiated PDACs and the PDACs with impaired TGF-β signaling were the most mechanically-activated and expressed elevated YAP and SOX2, which are transcriptional regulators that have been implicated in tumor aggression and epithelial-to-mesenchymal transition54–57. Indeed, these same YAP- and SOX2-expressing tumors expressed markers of a mesenchymal-like phenotype including vimentin, fibronectin and tenascin C (Fig. 6b)58–60. Our findings thus imply that the more aggressive phenotype of PDACs with a quasi-mesenchymal phenotype may be linked to their altered mechano-behavior. Moreover, given that YAP signaling can drive Kras-independent PDAC growth and survival, our observations indicate that fibrotic PDAC tumors may naturally evolve towards Kras independence and that this phenotype will be potentiated by specific tumor genotypes that increase tissue fibrosis including loss of TGF-β signaling. Our data thereby present one plausible reason why there has been such a mixed response of PDAC cancer patients to anti-stromal therapies, and suggest that FAK and JAK inhibitors, which target both stroma and epithelial-driven fibrosis, and both of which reduced YAP and tenascin induction in our studies, may comprise more efficacious therapeutic options.
Experimental procedures
Human samples
Formalin-fixed, paraffin-embedded human PDAC tissue sections, lacking any patient-identifying information, were commercially purchased from US Biomax (PA961a) or obtained from cohort of patients based on long and short survival. For non-commercial human samples, the study was approved by Regional Scientific Ethics Committee of the Capital Region of Denmark (protocol H-KA-20060181) in Region Hovedstaden, Denmark. The use of control materials was approved by the Ethical Committees in Bergen, Norway and in Heidelberg, Germany32. Informed consent was obtained from human subjects. Snap-frozen human PDAC tissues were collected from autopsy participants with stage IV disease in association with the Gastrointestinal Cancer Rapid Medical Donation Program (GICRMDP) and the study was approved by the Johns Hopkins Institutional Review Board (protocol 36610,36).
Mice studies
All mice were maintained in accordance with University of California Institutional Animal Care and Use Committee guidelines under protocol number AN105326-01D. Transgenic mouse strains, KrasLSL-G12D/+;Ptf1a-Cre (KC;37), KrasLSL-G12D/+;Tgfbr2flox/wt or flox/flox;Ptf1a-Cre (KTC;22); KrasLSL-G12D/+;Tp53R172H/+; Pdx1-Cre (KPC;38) LSL-β1V737N48, Stat3flox/flox 51 and constitutively active Stat3C50 were described previously. Mice were interbred and maintained in mixed background. Aged matched littermates not expressing Cre as well as Ptf1a-Cre and Pdx1-Cre mice were used as controls with mixed sexes were used for all experiments with the exception of xenografts studies which only included female mice.
For FAK inhibition studies, Ptf1a-Cre/β1-V737N mice were treated with FAK inhibitor PND-1186 at 0.5 mg/ml in 5% sucrose in the drinking water, control mice were provided 5% sucrose as drinking water (n = 5 per group). Treatment started at 3 weeks of age. At this time point, mice exhibit pancreatitis and fibrosis at 3 weeks of age and mice were killed after 3 weeks due to animal protocol guidelines. For JAK inhibition, KTC mice were treated with JAK inhibitor Ruxolitinib twice daily by oral gavage at 60 mg/kg body weight in 0.5% methylcellulose in water. Control mice were provided 0.5% methylcellulose in water (n = 5 per group). Treatment started at 3 weeks and mice were killed after 3 weeks. Mixed sexes were used for all experiments with the exception of xenograft studies which only included female mice.
For orthotopic xenografts, 5×105 firefly luciferase-mApple expressing cells in Matrigel (BD Biosciences) were injected into the pancreas of 8-week old nude mice (Simonsen laboratory) (n = 5 per group) and tumor growth was monitored by weekly bioluminescent imaging. Mice were sacrificed at 2 weeks following the cell injection due to animal protocol. Female mice were used in the xenografts studies.
For bioluminescent imaging animals were injected intraperitoneally with 3 mg of D-Luciferin and imaged using IVIS spectrum imaging system. The living imaging 4.3 software was used for analysis of the images post acquisition.
For all animal studies, animals were randomly distributed among the different conditions by the investigator since the animals did not exhibit any size or appearance differences at the onset of the experiments. No animals were excluded, and the investigator was not blinded during the experiment.
Histology
Paraffin-embedded or Fresh frozen pancreatic tissues were analyzed by H&E, picrosirius red, masson’s trichrome or alcian blue according to the manufacturer’s instructions. For mouse and clinical studies, staining intensity of tissue sections was scored blinded pathologist.
LC-MS/MS and LC-SRM Proteomic analysis
Proteomic analysis was performed in triplicate on 5 milligrams of fresh frozen KC, KPC, and KTC pancreatic tissues as previously described61. Briefly, tissues were milled in liquid N2 followed by sequential extraction of cellular proteins, soluble ECM proteins, and insoluble ECM proteins. Tryptic digests of all fractions were analyzed by liquid chromatography-select reaction monitoring LC-SRM mass spectrometry. Stable Isotope Labeled (SIL) peptides were used for absolute quantification of ECM proteins by LC-SRM.
Atomic force microscopy measurements
Atomic force microscopy and analysis were performed as previously described62. Frozen tissue blocks were cut into 20 µm thick sections. Prior to the atomic force microscopy (AFM) measurement, each section was immersed in PBS and thawed at room temperature. The samples were maintained in proteinase inhibitor in PBS (protease inhibitor cocktail, Roche 14 Diagnostics, 11836170001) supplemented with Propidium Iodide (SIGMA P4170, 20 µg/ml) during the AFM session. Five samples for each group were used for AFM quantification of Young’s elastic modulus of the cancer-associated stroma. AFM indentations were performed using an MFP3D-BIO inverted optical AFM (Asylum Research) mounted on a Nikon TE2000-U inverted fluorescent microscope, as previously described (Lopez et al., 2011). Briefly, we used silicon nitride cantilevers with spring constant of 0.06 N/m with borosilicate glass spherical tip with 5 µm in diameter (Novascan Tech). The cantilever was calibrated using the thermal oscillation method prior to each experiment. Samples were indented at a 20 µm/s loading rate, with a maximum force of 2 nN. Five AFM force maps were typically obtained on each sample, each map as a 10×10 µm raster series of indentations utilizing the FMAP function of the IGOR PRO build supplied by Asylum Research. The Hertz model was used to determine the elastic properties of the tissue (E1). Tissue samples were assumed to be incompressible and a Poisson’s ratio of 0.5 was used in the calculation of the Young’s elastic modulus.
Two-photon second harmonics microscopy and image analysis
Two-photon imaging of pancreatic tissues and collagen gels was performed and quantified as previously described63. For two-photon imaging, we used custom resonant-scanning instruments based on published designs containing a 5-PMT array (Hamamatsu, C7950) operating at video rate (11). The setup was used with 2 channel simultaneous video rate acquisition via 2 PMT detectors and an excitation laser (2W MaiTai Ti-Sapphire laser, 710–920 nm excitation range). Second harmonics imaging was carried out on a Prairie Technology Ultima System attached to an Olympus BX-51 fixed stage microscope equipped with a ×25 (NA 1.05) water immersion objective. Unfixed, hydrated samples were exposed to polarized laser light at a wavelength of 830 nm and emitted light was separated with a filter set (short pass filter, 720 nm; dichroic mirror, 495 nm; band pass filter, 475/40 nm). Images of×and y planes of 284 by 284 µm at a resolution of 0.656 µm/pixel were captured using Micro-Manager Open Source Microscopy Software (Micro-Manager). Quantification of collagen fibers was achieved by setting a minimal threshold in the second harmonic signal. The threshold was maintained for all images across all conditions. The area of regions that was covered by the minimal threshold was calculated and 3 images per sample were averaged together (Image J, Image Processing and Analysis in Java). Collagen fiber diameters data were visualized and analyzed using Imaris (Bitplane AG) and MATLAB (MathWorks).
Cell culture and establishment of primary pancreatic cell lines
Establishment of primary pancreatic tumor cells was performed as described previously64. Cells were maintained in RPMI-1640 medium containing 10% FBS (Clontech). All experiments were performed within eight passages to avoid possible gross genomic changes during long-term in vitro culture. Cells were verified by epithelial morphology in two dimensions (2D), expression of the epithelial marker cytokeratin 19 and the ability to form tumors in vivo. Cells were tested for mycoplasma contamination using commercially available kit (PCR-Mycoplasma Test Kit I/C (Promokine PK-CA91-1024) according to manufacturer’s manual at the onset of the work (tested negative) and have never exhibited contamination symptoms after the test. Derivative cells were transduced in vitro with a lentiviral vector-encoding firefly Luciferase and mApple fluorescent protein for combined bioluminescent imaging and flow sorting. To ensure that all cell lines expressed similar levels of luciferase, mApple expressing cells were flow sorted prior to injection into animals.
Soft agar assay
Anchorage-independent growth was assessed using a soft agar assay (65). In brief, 25,000 cells in 1.5 ml 0.35% agarose containing 1× growth media were overlaid with 1.5 ml 0.5% agarose containing 1× growth media, and colonies larger than 30 µm in diameter were scored positive after 14 days.
Lentivirus-mediated shRNA knockdown
Lentiviral shRNA targeting ROCK1, ROCK2 and FAK and nontargeting shRNA control were cloned in the pLKO vector. The clone IDs for shRNA are as follows: sh-ROCK1 (TRCN0000022903), sh-ROCK2 (TRCN0000022922), sh-FAK (TRCN0000023485) and eEGFP as a non specific control cloned into the lentiviral pLKO.1 puro vector (Sigma-Aldrich).
Preparation of luciferase-mApple Virus-Infected Cells
Lentivirus infection was performed with pLKO EEF1α mApple luc2 lentiviral vector for combined flow sorting and in vivo imaging. This was constructed from the pLKO.1 puro vector by inserting an in-frame fusion of mApple with the human codon optimized firefly luciferase gene from pGL4.10[luc2] (Promega) and placing this under control of the human EEF1α promoter. This cassette, followed by a WPRE element was inserted in place of the U6 cppt mPGK puro elements in pLKO.1 puro (from claI to kpnI sites).
Quantitative real-time PCR
RNA was extracted using trizol/chloroform isolation followed by Ambion mirVana kit (AM1560). Total RNA was reverse-transcribed using random primers (Amersham Biosciences), and β-actin primers were used to control for cDNA concentration in a separate PCR reactions for each sample. LightCycler Fast Start DNA Master SYBR Green Mix (Roche) was added to each PCR reaction along with cDNA and 1 pmol primer in a total volume of 10 µl. qPCR was performed on a eppendorf realplex2 mastercycler using the real-time primers provided, according to instructions. Ct values were converted to fold expression changes (2−ΔΔCt values) following normalization to β-actin. Primer sequences are listed below.
Gene | Sequence |
---|---|
CTGF | F 5’-GTGAGTCCTTCCAAAGCAGC-3’ |
R 5’-TAGTTGGGTCTGGGCCAAAT-3’ | |
β-actin | F 5’-GTGGGCCGCCCTAGGCACCA-3’ |
R 5’-CGGTTGGCCTTAGGGTTCAGGG-3’ |
Traction force microscopy
Traction of pancreatic cells was measured as previously described18,66. Images of cells on PA gels containing fluorescent beads were collected before and after trypsinization using a Nikon Inverted Eclipse TE300 microscope and a Photometric Cool Snap HQ camera (Roper Scientific). TFMs were calculated based on differences in bead displacement induced by substrate deformation and relaxation using a software analysis program.
Three dimensional collagen contraction assay
Collagen contraction assay was performed as previously described42 Briefly, collagen I solution was prepared by neutralizing acid-solubilized rat tail collagen I (BD Bioscience) with 1 N NaOH and a DMEM buffer, 300 ul/well in 24 well plate and polymerized at 37 C. Cells were seeded on top of collagen and allowed to adhere overnight. Collagen gel area was quantified using NIH ImageJ analysis software.
Polyacrylamide substrates
Basement membrane–conjugated polyacrylamide hydrogels were prepared as previously described (Lakins, Methods Mol. Biol. 916, 317–350 (2012) with one modification: n-succinimidyl acrylamidohexanoic acid crosslinker was conjugated to polyacrylamide substrates using 0.01% bisacrylamide, 0.025% Irgacure 2959 0.002% Di(trimethylolpropane) tetracrylate (Sigma) and 0.01% n-succinimidyl acrylamidohexanoic acid.
Cytokine antibody array
Proteins from the pancreatic cells or tissues were screened by using the Mouse Cytokine Antibody Array A (R&D ARY006) according to the manufacturer’s instructions. In brief, conditioned media or protein lysate were collected and subjected to the antibody array incubation. The amount of conditioned media used was normalized by the cell number. The membranes were washed and incubated with primary biotin-conjugated antibody and then incubated with horseradish peroxidase–conjugated streptavidin, and the protein spots were detected using the ECL Western blotting detection reagents (GE Healthcare) according to the manufacturer’s instructions. The spot density was quantified and compared between different conditions.
Tissue processing and Immunostaining
Fresh tumors were harvested, embedded in O.C.T. medium (TissueTek) prior to freezing in liquid nitrogen. Frozen sections 5 µm thick were fixed in PFA for 15 min. Paraffin embedded harvested tumors were formalin fixed prior to paraffin embedding. Sections 5 µm thick were deparaffinized, rehydrated and boiled for 1 hour in 10mM citrate buffer at pH 6.0. For all stains, the tissue sections were blocked with 5% normal goat serum/0.3% Triton™ X-100 in PBS for 60 minutes at room temperature. Sections were then incubated with primary antibodies overnight at 4C followed by incubation with fluorochrome conjugated secondary antibodies for 1 hour at room temperature. 1% BSA/0.3% Triton™ X-100 in PBS was used to dilute primary and secondary antibodies. DAPI was used to stain cell nuclei. All stainings were quantified using NIH ImageJ analysis software with the same threshold for each stain; positivity was analyzed in five visual fields for each sample and results were expressed as percentage staining per visual field.
Antibodies and reagents
Antibodies were as follows: a-SMA (Sigma-Aldrich C6198, 1:1000), GLi-1 (Thermo Scientific PA5-32206, 1:500), Tenascin C (Abcam AB108930, 1:500), Fibronectin (Abcam AB2413, 1:500), Collagen 12A1 (Santa Cruz Biotechnology E-15 sc-68449, 1:200), Sox2 (Abcam ab97959, 1:500), Vimentin (Cell Signaling 5741S, 1:200), FAP (Abcam AB53066, 1:500), Collagen III (Abcam AB7778, 1:500), YAP (Cell signaling 4912, 1:200), β1integrin (EMD Millipore MABT409, 1:500), pY397-FAK (Invitrogen 44625, 1:200), total FAK (BD Biosciences 610088, 1:1,000), ROCK1 (Cell Signaling C8F7, 1:1,000), ROCK2 (Cell Signaling D1B1, 1:1,000), p-MLC2 (Cell Signaling 3671, 1:200), p-MyPT1 (Millipore ABS45, 1:200), p-Stat3 (Cell Signaling 9145, 1:200), p-SMAD2/3 (Cell Signaling 8828, 1:200), total Stat3 (Cell Signaling 9132, 1:1,000), GAPDH (Cell Signaling 2118, 1:5,000), CD45 (BD Biosciences 550539, 1:200), CD68 (Thermo Scientific Ab-3, 1:200), Alexa Fluor-conjugated goat secondary anti–mouse IgG and anti–rabbit IgG antibodies (Invitrogen A11012, 1:1,000 and A11005, 1:1,000) and HRP-conjugated rabbit secondary antibody (GE health care life sciences NA934VS, 1:5,000).
Immunoblotting
Iimmunoblotting analysis were performed as previously described67. In brief, equal amounts of cell protein lysate (Laemmli) were separated on reducing SDS-PAGE gels, transferred to nitrocellulose membrane, and probed with primary antibody. Bands were visualized and quantified using a PXI 6 Touch (Syngene), in conjunction with HRP-conjugated secondary antibodies and ECL (Amersham Pharmacia).
Flow sorting
Weighed tumors were minced and allowed to digest in a mixture of 1mg/ml of collagenase P (Roche 11213857001), 0.1 mg/ml Collagenase I (Sigma, C0130), 0.1 mg/ml Dispase II (Roche, 04942078001) and DNase (5MU/ml, Calbiochem, 260913) in DMEM media at 37°C for 30 min. The tissue lysate was filtered through a 40 µm mesh prior to immunostaining. The resulting single-cell suspension was stained with fixable viability dye eFluor 780, anti-CD45-APC-Cy7 (103115, 1:200), anti-CD11b-FITC (101205, 1:100) and anti-Ly6/C-PE (128007, 1:100) (all from BioLegend). The percentage of positive cells were analyzed by FlowJo and gated on CD45 positivity. Unstained, live/dead only, and single stain served as control. Doublets were gated out using forward-scatter width/height and side-scatter width/height event characteristics.
Bioinformatics
Patient expression data was pulled from publicly available dataset GSE21501 obtained from NCBI GEO. Following baseline normalization, expression values for Col1a2, Col2a1, and Col4a1 were used to create a median centroid value representing an average collagen expression score for each patient. These values were then divided into high and low expression based on median centroid value for the patient set and graphed as survival of each group over time. Kaplan–Meier survival curves were analyzed by Cox–Mantel Log-rank analysis.
Statistics
All quantitative results were assessed by unpaired Student’s t-test after confirming that the data met appropriate assumptions (normality, homogenous variance, and independent sampling), non-parametric Wilcox/Mann Whitney exact test (using the normal approximation for the U score), or the Kolmogorov-Smirnov distribution test, all two-tailed. For all in vitro experiments, 3 technical replicates were analyzed for each experiment and results are presented as the mean +/− SEM of 3 biological replicates. For all in vivo experiments, 5 biological replicates were analyzed for each condition and results are presented as the mean +/− SEM. Subsequent statistical analysis was performed with either unpaired two-sided student t-tests, one-way ANOVA with Tukey’s method for multiple comparisons. For survival analysis, the log-rank (Mantel-Cox) test was used. Prism software was used to conduct the statistical analysis of all data. P-values of less than 0.05 were considered to be significant. *p<0.05; **p<0.01, ***p<0.001, ****p<0.0001, “ns” denotes not significant for all experiments. Sample size estimation was initially performed using the Mann-Whitney test (Lab. Animal Sci. 41:6-16, 1991) as a guide and taking into consideration previous experience with assay sensitivity, tissue collection and the different animal strains utilized. For the listed sample size per group at each time point, the numbers provide a minimum of 85% power to detect an effect size of 2.5 (tumor size, stiffness, gene expression, protein expression) with two-sided type I error = 5% (p≤0.05). The effect size is defined (where applicable). For animal studies, animals were randomly distributed among the different conditions by the investigator since the animals did not exhibit any size or appearance differences at the onset of the experiments. No animals were excluded, and the investigator was not blinded during the experiment. For mouse and clinical studies, staining intensity of tissue sections was scored blinded.
Acknowledgments
We thank Dr. M. Dembo for the LIBTRC 2.0 traction force software. Animal handling was supported by L. Korets. This work was supported by US National Institutes of Health NCI grants U01 CA151925-01 (V.M.W., H.L.M. and R.K.), R33 CA183685-01 (V.M.W., K.H.), R01 CA138818-01A1 (V.M.W.), U54CA143836-01 (V.M.W.), CA102310 (D.D.S.), R01CA178015-02 (E.A.C.), R01 CA172045 (M.H.), T32CA108462 (M.W.P.), F31CA180422 (Y.A.M.), the Pancreatic Cancer Action Network- AACR Innovative Grant 30-60-25-WEAV (V.M.W.), NSF GRFP 1144247 (Y.A.M.) and NIH TL1 TR001081 (A.S.B.).
Footnotes
Author Contributions
V.M.W. and H.L. conceived the project. H.L. designed and conducted transgenic mice experiments and treatments, performed histology, immunofluorescence and image analysis on mice and human samples, fabricated and conducted experiments with the PA hydrogels, performed 3D collagen and soft agar assays, performed the cytokine array and analysis, and performed the immunoblotting analyses. H.L. and Y.A.M. performed AFM imaging and analysis. Y.A.M. performed TFM and two-photon imaging and analysis. H.L. and M.W.P. conducted orthotopic xenografts experiments. M.W.P performed RT-PCR and bioinformatics. S.V.N. and M.W.P. performed flow sorting. J.N.L designed and constructed expression constructs and the V737N transgenic mouse. E.A.C. and G.E.K. aided with pathological pancreatic cancer scoring of transgenic mice and human samples. J.S.J. and E.A.C. collected human samples from short and long survival patients and aided in the interpretation of the data. C.A.I-D and L.D.W collected human samples from wild type and mutant SMAD4 patients and aided in the interpretation of the data. R.K., V.S.L, M.H and N.R. provided KPC pancreatic tissues. V.P. provided Stat3flox/flox and constitutively active Stat3C transgenic mice. A.S.B., R.C.H and K.H. performed LC-MS/MS and LC-SRM Proteomic analysis. D.D.S provided FAK inhibitor PND-1186.H.L.M designed and provided KTC transgenic mouse, provided KC mice and aided in interpretation of data. V.M.W., H.L, Y.A.M., J.K.M and M.W.P wrote the manuscript with input from all authors.Competing Financial Interests
We currently have no competing financial interests to report.
References
References
Full text links
Read article at publisher's site: https://doi.org/10.1038/nm.4082
Read article for free, from open access legal sources, via Unpaywall:
https://europepmc.org/articles/pmc4860133?pdf=render
Citations & impact
Impact metrics
Citations of article over time
Alternative metrics
Article citations
Genetic variation drives cancer cell adaptation to ECM stiffness.
Proc Natl Acad Sci U S A, 121(39):e2403062121, 20 Sep 2024
Cited by: 0 articles | PMID: 39302966 | PMCID: PMC11441511
Precision Targeting Strategies in Pancreatic Cancer: The Role of Tumor Microenvironment.
Cancers (Basel), 16(16):2876, 19 Aug 2024
Cited by: 0 articles | PMID: 39199647 | PMCID: PMC11352254
Review Free full text in Europe PMC
Temporally resolved proteomics identifies nidogen-2 as a cotarget in pancreatic cancer that modulates fibrosis and therapy response.
Sci Adv, 10(27):eadl1197, 03 Jul 2024
Cited by: 0 articles | PMID: 38959305 | PMCID: PMC11221519
The importance of 3D fibre architecture in cancer and implications for biomaterial model design.
Nat Rev Cancer, 24(7):461-479, 17 Jun 2024
Cited by: 1 article | PMID: 38886573
Review
Boosting antitumor efficacy of nanoparticles by modulating tumor mechanical microenvironment.
EBioMedicine, 105:105200, 13 Jun 2024
Cited by: 0 articles | PMID: 38876044 | PMCID: PMC11225208
Review Free full text in Europe PMC
Go to all (348) article citations
Data
Data behind the article
This data has been text mined from the article, or deposited into data resources.
BioStudies: supplemental material and supporting data
Similar Articles
To arrive at the top five similar articles we use a word-weighted algorithm to compare words from the Title and Abstract of each citation.
Signal Transducer and Activator of Transcription 3, Mediated Remodeling of the Tumor Microenvironment Results in Enhanced Tumor Drug Delivery in a Mouse Model of Pancreatic Cancer.
Gastroenterology, 149(7):1932-1943.e9, 07 Aug 2015
Cited by: 106 articles | PMID: 26255562 | PMCID: PMC4863449
Interleukin 22 Signaling Regulates Acinar Cell Plasticity to Promote Pancreatic Tumor Development in Mice.
Gastroenterology, 158(5):1417-1432.e11, 14 Dec 2019
Cited by: 36 articles | PMID: 31843590 | PMCID: PMC7197347
TGIF1 functions as a tumor suppressor in pancreatic ductal adenocarcinoma.
EMBO J, 38(13):e101067, 31 May 2019
Cited by: 16 articles | PMID: 31268604 | PMCID: PMC6601038
The Roles of Frequently Mutated Genes of Pancreatic Cancer in Regulation of Tumor Microenvironment.
Technol Cancer Res Treat, 19:1533033820920969, 01 Jan 2020
Cited by: 13 articles | PMID: 32372692 | PMCID: PMC7225789
Review Free full text in Europe PMC
Funding
Funders who supported this work.
NCATS NIH HHS (2)
Grant ID: TL1 TR001081
Grant ID: UL1 TR001082
NCI NIH HHS (12)
Grant ID: F31 CA180422
Grant ID: U54 CA143836
Grant ID: R01 CA102310
Grant ID: R01 CA138818
Grant ID: R33 CA183685
Grant ID: T32 CA108462
Grant ID: R01 CA180769
Grant ID: R01 CA192914
Grant ID: U01 CA151925
Grant ID: R01 CA178015
Grant ID: R01 CA172045
Grant ID: U01 CA202241