Abstract
Free full text

Insulin induction of SREBP-1c in rodent liver requires LXRα-C/EBPβ complex
Significance
In patients with type 2 diabetes, excessive insulin stimulates the conversion of glucose to fat in the liver. The resultant fatty liver can cause liver failure. Fat accumulation in blood produces hypertriglyceridemia, which accelerates heart attacks. Insulin increases fat production by increasing the mRNA-encoding sterol regulatory element-binding protein-1c (SREBP-1c), which activates genes encoding enzymes required for fat synthesis. If we can understand how insulin increases SREBP-1c mRNA, we may be able to prevent insulin-induced fat synthesis. Here we show that insulin action requires a complex of two nuclear proteins, LXRα and C/EBPβ, which activate the Srebp-1c gene. This work provides insight into a mechanism that controls fat production and has major implications for health.
Abstract
Insulin increases lipid synthesis in liver by activating transcription of the gene encoding sterol regulatory element-binding protein-1c (SREBP-1c). SREBP-1c activates the transcription of all genes necessary for fatty acid synthesis. Insulin induction of SREBP-1c requires LXRα, a nuclear receptor. Transcription of SREBP-1c also requires transcription factor C/EBPβ, but a connection between LXRα and C/EBPβ has not been made. Here we show that LXRα and C/EBPβ form a complex that can be immunoprecipitated from rat liver nuclei. Chromatin immunoprecipitation assays showed that the LXRα-C/EBPβ complex binds to the SREBP-1c promoter in a region that contains two binding sites for LXRα and is known to be required for insulin induction. Knockdown of C/EBPβ in fresh rat hepatocytes or mouse livers in vivo reduces the ability of insulin to increase SREBP-1c mRNA. The LXRα-C/EBPβ complex is bound to the SREBP-1c promoter in the absence or presence of insulin, indicating that insulin acts not by increasing the formation of this complex, but rather by activating it.
Sterol regulatory element-binding protein-1c (SREBP-1c) is a transcription factor that activates all of the genes required for fatty acid synthesis (1). In liver, SREBP-1c is activated by insulin. In livers of fasted mice and rats, plasma insulin is low and SREBP-1c mRNA levels are barely detectable. SREBP-1c mRNA increases by up to 40-fold when the animals are fed and plasma insulin rises (2, 3). The insulin induction can be mimicked by adding insulin at nanomolar concentrations to freshly isolated rat hepatocytes (4, 5).
The enhancer elements necessary for insulin-mediated induction of SREBP-1c mRNA in fresh hepatocytes were delineated by transfection of plasmids encoding luciferase under control of the SREBP-1c enhancer region (6). All of the insulin-inducing activity was found to be contained within a fragment containing 368 nucleotides upstream of the transcription start site. This region contains two elements that are recognized by liver X receptors (LXRs), nuclear receptors that control lipid metabolism. Deletion of either of these two LXR elements markedly reduced insulin-mediated of SREBP-1c transcription (6). Moreover, administration of LXR agonists to mice markedly increased SREBP-1c mRNA levels in liver from wild-type mice, but not from LXRαβ−/− knockout (KO) mice (7).
C/EBPβ is another transcription factor that has been implicated in activation of SREBP-1c transcription in liver. The founding member of the C/EBP family was identified as a transcription factor by Graves et al. (8), and it served as the prototype for the delineation of leucine zipper domains as dimerization surfaces (9). Subsequent studies expanded the family to include α and β homologs (10). Germ-line deletion of the gene encoding C/EBPβ led to reduced SREBP-1c mRNA levels in the liver of mice on a high-fat diet, and the mRNAs for the SREBP-1c target genes declined (11). One caveat is that these mice had diminished white adipose tissue, owing to the requirement for C/EBPβ in adipose differentiation. The diminished SREBP-1c in liver might have been attributable to a decreased insulin level in these fed mice. In another study, Schroeder-Gloecker et al. (12) produced mice deficient in C/EBPβ and in the leptin receptor. Elimination of C/EBPβ reduced adiposity, and mRNA levels for SREBP-1c target genes in liver were reduced; however, these authors did not observe a decrease in nuclear SREBP-1c in their C/EBPβ-deficient mice. The caveat here is that the authors used a commercial anti-SREBP antibody, and they did not provide control data showing that the antibody was specific for SREBP-1c.
In the present study, we explored the relationship between LXRs and C/EBPβ in activating the SREBP-1c gene in rat liver. We used a newly prepared and highly specific anti-LXRα antibody that efficiently immunoprecipitates the protein, and found that C/EBPβ and LXRα form a tight immunoprecipitable complex in vivo and in vitro. Chromatin immunoprecipitation (ChIP) assays revealed that this complex binds to the region of the SREBP-1c enhancer that contains the LXR elements. Knockdown of C/EBPβ by RNA interference in fresh rat hepatocytes or in mouse liver reduced the SREBP-1c induction by insulin. We conclude that both LXRα and C/EBPβ are required for insulin induction of SREBP-1c mRNA, and that the two proteins function by forming a complex.
Results
To facilitate our study of LXR-interacting proteins, we immunized mice with full-length rat LXRα and prepared a mouse monoclonal antibody designated IgG-2B7. IgG-2B7 visualizes a single protein of the apparent molecular weight of LXR (49 kDa) in nuclear extracts from wild type mouse and rat livers (Fig. 1A, lanes 1 and 3). This band was absent in extracts from KO mice that did not express LXRα or β (Fig. 1A, lane 2). We used IgG-2B7 for all of the LXR immunoprecipitation experiments. For immunoblotting, we also used a rabbit polyclonal antibody directed against full-length rat LXRα, and found that this polyclonal antibody also recognized a protein corresponding to LXR in mouse and rat liver (Fig. 1B).
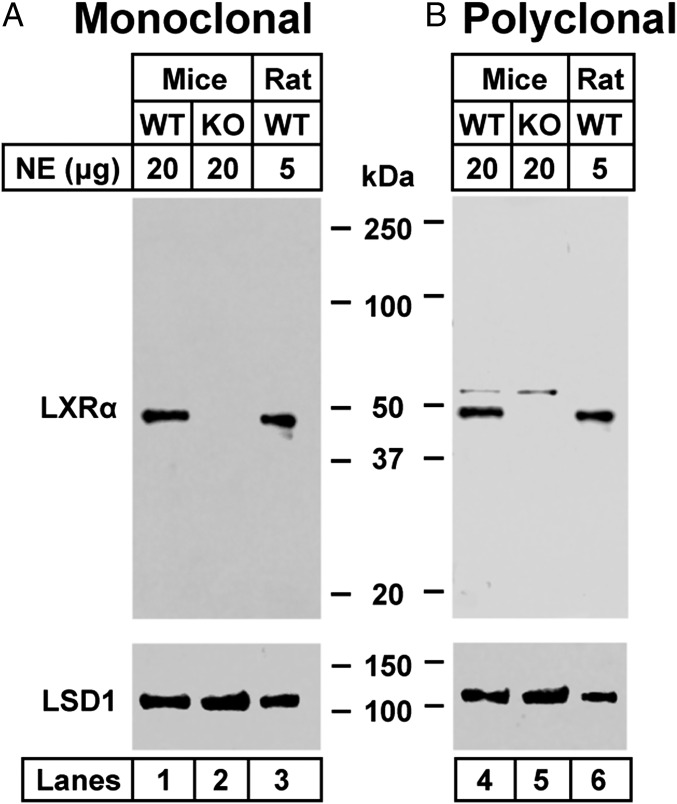
Immunoblot analysis of mouse and rat nuclear extracts using mouse monoclonal anti- LXRα (A) and rabbit polyclonal anti-LXRα (B). The indicated amount of nuclear extracts (prepared as described in Materials and Methods) from WT mice, LXRαβ−/− (KO) mice, and WT rats was subjected to 4–12% SDS/PAGE, followed by immunoblot analysis with 1.5 µg/mL of mouse monoclonal anti-LXRα (A) or rabbit polyclonal anti-LXRα (B). LSD1 served as a loading control and was immunoblotted with a 1:1,000 dilution of rabbit monoclonal anti-LSD1. Proteins were detected with the LI-COR Odyssey Infrared Imaging System using a 1:5,000 dilution of anti-mouse IgG or anti-rabbit IgG conjugated to horseradish peroxidase. NE, nuclear extract.
Based on previous data implicating C/EBPβ in promoting the transcription of SREBP-1c in adipocytes and liver (11, 13, 14), and based on our previous demonstration that LXRα is required for SREBP-1c transcription in rat liver (6), we investigated whether C/EBPβ interacts with LXRα in liver, and whether that interaction is affected by insulin. Nuclear extracts were prepared from livers of rats that had been fasted for 48 h (low-insulin group) or fasted for 48 h and then refed for 6 h (high-insulin group). After immunoprecipitation with IgG-2B7, the precipitated material was subjected to SDS/PAGE and blotted with an antibody to C/EBPβ. Fig. 2A shows a schematic diagram of the three isoforms of human C/EBPβ (15). LAP1 is the full-length protein (345 amino acids; predicted mass), LAP2 lacks the N-terminal 23-amino acids, and LIP is a truncated isoform that retains the DNA-binding domain but lacks the transactivation domain. LIP is thought to be an inhibitor of C/EPBβ. Rat liver also contained the three isoforms of C/EPBβ (Fig. 2B, lanes 1 and 2). LAP1 and 2 were coimmunoprecipitated with IgG-2B7, but LIP was not. C/EPBPα also was not precipitated, confirming the specificity of the LXRα-C/EBPβ coimmunoprecipitation (co-IP).
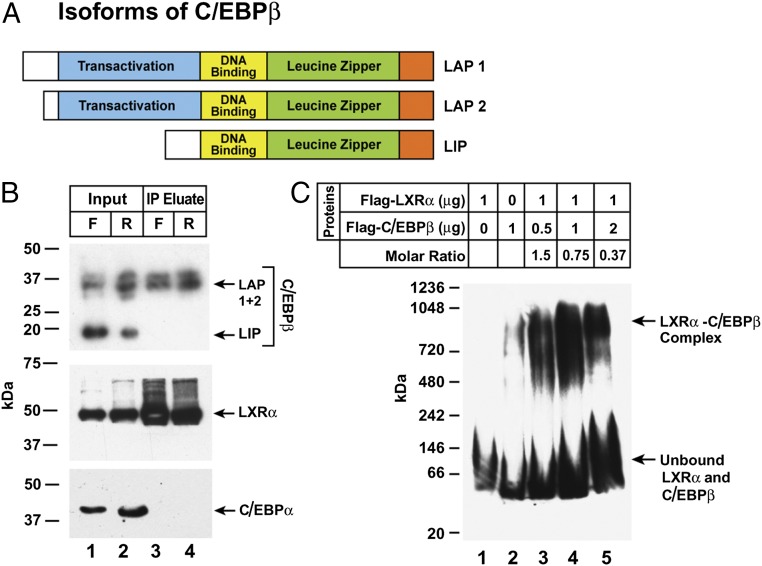
Interaction between C/EBPβ and LXRα. (A) Schematic diagram showing the three isoforms of C/EBPβ. (B) Anti-LXRα–mediated co-IP. Nuclear extracts were prepared from livers of rats subjected to either 48-h fasting (F) or 48-h fasting followed by 6-h refeeding (R). LXRα was immunoprecipitated with 5 µg/mL of monoclonal IgG-2B7 as described in Materials and Methods. An aliquot of the input (20% of total) and the entire eluted immunoprecipitated fraction were subjected to 4–12% SDS/PAGE and immunoblotted with a 1:1,000 dilution of monoclonal anti-C/EBPβ, 5 µg/mL of polyclonal anti-LXRα, or a 1:1,000 dilution of polyclonal anti-C/EBPα. Blots were exposed to film for 5–30 s at room temperature. (C) Complex between recombinant LXRα and C/EBPβ. The indicated amounts of purified recombinant Flag-LXRα and Flag-C/EBPβ were preincubated in 20 µL of buffer B for 2 h at 4 °C, after which the protein mixture was subjected to 4–16% blue native-PAGE and immunoblot analysis with 1:1,000 dilution of anti-Flag antibody. The blot was exposed to film for 10 s at room temperature.
To demonstrate the formation of an LXRα-C/EBPβ complex in vitro, we used recombinant Flag-tagged versions of LXRα and C/EBPβ, mixed them together, and subjected the mixture to blue native gel electrophoresis followed by blotting with anti-Flag antibody. When the two proteins were incubated separately, they ran as monomers (Fig. 2C, lanes 1 and 2). When the amount of LXRα was held constant and the amount of C/EBPβ was increased, the two proteins formed a complex that migrated much more slowly in this native gel system (Fig. 2C, lanes 3–5).
To determine a role for C/EBPβ in insulin-mediated induction of SREBP-1c mRNA, we introduced an siRNA targeting C/EBPβ into fresh rat hepatocytes by transfection and then treated the cells with insulin (Fig. 3). An siRNA targeting GFP served as a control. The C/EBPβ siRNA reduced the C/EBPβ mRNA by 78% (Fig. 3A). In the absence of the siRNA, insulin increased the SREBP-1c mRNA by 5.5-fold (Fig. 3B). The C/EBPβ siRNA markedly inhibited this induction, but the control GFP siRNA had no effect. The C/EBPβ siRNA did not reduce the RNA encoding C/EBPα (Fig. 3C).

Knockdown of C/EBPβ inhibits insulin-induced increase of SREBP-1c mRNA in rat primary hepatocytes. (A) C/EBPβ mRNA. (B) SREBP-1c mRNA. (C) CEBPα mRNA. On day 0, hepatocytes were isolated and plated as described in Materials and Methods. On day 1, the cells were transfected with 50 nM of control siRNA (siGFP) or siRNA targeting C/EBPβ (siC/EBPβ). After 6 h, cells were switched to medium B. On day 2, cells were treated with or without 100 nM insulin for 6 h and then harvested for measurement of the indicated mRNA by quantitative RT-PCR. Each value represents the mean ± SEM of three incubations. The Ct values in the absence of insulin for SREBP-1c, C/EBPβ, and CEBPα were 24.9, 23.8, and 25.3, respectively.
To determine whether C/EBPβ is required for activation of other LXR target genes, we reduced C/EBPβ expression with siRNA and treated the cells with T0901317, a direct LXR activator (16). We measured mRNAs for SREBP-1c and ABCA1, an LXR target (17). As before, insulin increased the SREBP-1c mRNA, and this effect was partially blocked by the C/EBP-β siRNA (Fig. 4A). Paradoxically, the C/EBPβ siRNA increased the ABCA1 mRNA in the absence or presence of insulin (Fig. 4B). T0901317 markedly increased the ABCA1 mRNA, but the C/EBPβ siRNA had no effect. These data indicate that C/EBPβ is required only for insulin induction of SREBP-1c mRNA. The data also suggest that insulin does not activate the SREBP-1c gene by creating a ligand for LXR. If an insulin-induced ligand were the sole activator of LXR, then activation of SREBP-1c would not have been inhibited by the C/EBPβ siRNA.
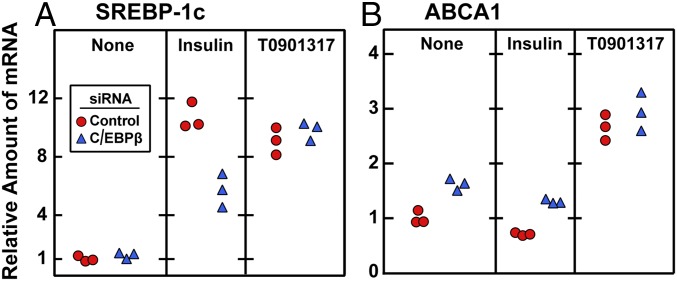
Knockdown of C/EBPβ does not reduce T0901317-induced SREBP-1c and ABCA1 mRNA levels in rat hepatocytes. On day 0, hepatocytes were isolated and plated as described in Materials and Methods. After attachment for 2 h, cells were transfected with 50 nM control siRNA (siControl) or siRNA targeting C/EBPβ (siC/EBPβ). On day 1, cells received one of the following treatments: none, 100 nM insulin, or 10 µM T0901317. After 6 h, cells were harvested for measurement by quantitative RT-PCR of the mRNA for SREBP-1c (A) and ABCA1 (B). Each circle or triangle represents an individual dish. The Ct values for SREBP-1c and ABCA1 without any treatment were 24.3 and 22.6, respectively. The Ct values for C/EBPβ before and after siC/EBPβ treatment were 23.2 and 25.0, respectively.
Fig. 5 shows an experiment in which we reduced the expression of C/EBPβ in mouse livers by injecting an adenovirus encoding an shRNA directed against C/EBPβ. When the mice were fasted for 12 h, the amount of SREBP-1c mRNA in liver was low, and there was no reduction by the C/EBPβ shRNA. When the mice were refed for 12 h, the amount of SREBP-1c mRNA increased by 56-fold. This increase was reduced by 45% in the mice that received the shRNA targeting C/EBPβ. Fig. 4B shows that the adenoviral shRNA markedly reduced the level of C/EBPβ protein as revealed by immunoblotting.
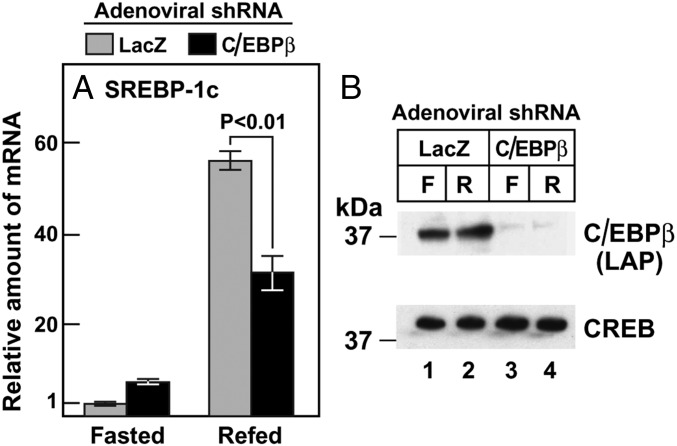
Adenovirus-mediated knockdown of C/EBPβ reduces SREBP-1c mRNA in livers of refed mice. (A) Male mice (age 6–8 wk; four mice/group) were injected with the indicated adenoviral shRNA as described in Materials and Methods. At 3 d after injection, the mice were subjected to fasting for 12 h, followed by refeeding for 12 h, after which total liver RNA was prepared for measurement of mRNA by quantitative RT-PCR. Each bar represents the mean ± SEM of values from four mice and is shown relative to the fasted value for mice treated with adenoviral shLacZ (denoted as 1). The Ct values for SREBP-1c in fasted and refed mice receiving adenoviral shLacZ were 25.5 and 20.1, respectively. (B) Nuclear proteins from the same mice as in A were extracted individually and pooled (four mice/sample) for immunoblotting analysis with 1:1,000 dilution of anti-C/EBPβ antibody. The blot was exposed to film for 3 s. Each value denotes the mean ± SEM of data from four animals. F, fasted; R, refed.
We performed ChIP to confirm the binding of the C/EBPβ-LXRα complex to the SREBP-1c enhancer/promoter region (Fig. 6A; ref. 6). We prepared slices of rat liver and incubated them with formaldehyde to cross-link proteins to DNA. The tissue was disrupted, and the DNA was sheared by sonication. After preclearance with protein A agarose/salmon sperm DNA resin, the supernatant was incubated with various antibodies, and the antibody-bound proteins were precipitated with protein A agarose. The precipitated DNA was subjected to PCR with primer pair 1, which amplifies the SREBP-1c enhancer region that includes two LXR binding sites; with primer pair 2, which amplifies a region 5 kb upstream; or with primer pair 3, which amplifies a region 50 kb upstream (Fig. 6A). Anti-C/EBPβ brought down DNA that was amplified by primer pair 1 (Fig. 6B). Although the data shown in Fig. 5 suggest that the amount of precipitable DNA was increased by refeeding, this was not a consistent finding. In an average of five experiments, the amount was the same when the liver slices were obtained from fed rats or from fasted rats. This segment of DNA was not precipitated by a control antibody (anti-IgG).
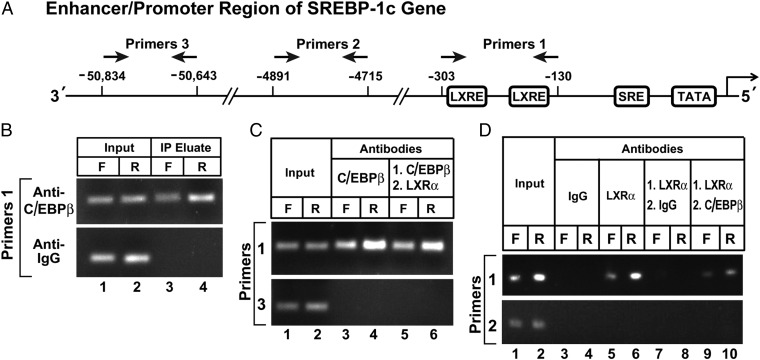
ChIP assay to demonstrate C/EBPβ binding to the enhancer/promoter region of rat SREBP-1c gene. (A) Schematic diagram of rat SREBP-1c enhancer/promoter region, showing the location of the two LXREs and one SRE (6). Arrows denote the location of the primers used in the ChIP assays. (B-D) ChIP assays were performed with livers from rats that had been subjected to either 48-h fasting (F) or 48-h fasting followed by 6-h refeeding, as described in Materials and Methods. After cross-linking, the sheared chromatin was incubated with the indicated antibody. (B) Incubation with 4 µg/mL anti-C/EBPβ or anti-IgG (control), after which the DNA was purified from each immunoprecipitate and subjected to PCR with primer pair 1. The products were visualized on an agarose gel. (C and D) Sequential ChIP using antibodies against C/EBPβ and LXRα. (C) In lanes 3 and 4, the incubation was performed with 4 µg/mL anti-C/EBPβ as in B. In lanes 5 and 6, the first incubation was performed with 4 µg/mL anti-C/EBPβ, after which the DNA recovered from the immunoprecipitate was subjected to a second precipitation with 4 µg/mL monoclonal anti-LXRα. (D) In lanes 3–6, the incubation was performed with 4 µg/mL anti-IgG (lanes 3 and 4) or anti-LXRα (lanes 5 and 6). In lanes 7–10, the sheared chromatin was first incubated with 4 µg/mL anti-LXRα, after which the DNA recovered from the immunoprecipitate was subjected to a second immunoprecipitation with 4 µg/mL anti-IgG (lanes 7 and 8) or anti-C/EBPβ (lanes 9 and 10). (C and D) The DNA recovered from the first or second immunoprecipitate was used for PCR with either primer pair 1 or primer pair 3 as indicated, and the products were visualized on an agarose gel.
The sheared DNA was first precipitated with anti-C/EBPβ (Fig. 6C, lanes 3 and 4), and the precipitated DNA was visualized by PCR with primer pair 1. The anti-C/EBPβ was then released by denaturation and the DNA was reprecipitated with anti-LXRα (Fig. 6C, lanes 5 and 6). The pelleted DNA was again subjected to PCR with primer pair 1, and again the amplified band was visualized in DNA from the fasted and fed mice. Amplification with primer pair 3 did not produce a positive signal.
We next reversed the order of antibodies, with the protein/DNA complex first precipitated with anti-LXRα and then subjected to a second precipitation with anti-C/EBPβ. Again we were able to amplify the DNA segment from the SREBP-1c promoter using primer pair 1 (Fig. 6D, lanes 9 and 10). No band was visible when we used the anti-IgG control antibody in the second immunoprecipitation (Fig. 6D, lanes 7 and 8). The antibodies did not precipitate a segment of DNA that is 4,700-bp upstream of the transcription start site, as visualized with primer pair 2.
Discussion
The present study indicates a requirement for C/EBPβ in the insulin-mediated induction of SREBP-1c mRNA expression in rodent liver. Coupled with previous data showing that this induction requires LXRα (6, 7), our data reported herein indicate a requirement for both transcription factors. Moreover, we show that C/EBPβ and LXRα are part of a protein complex that binds to a region of the SREBP-1c promoter that contains two LXRα-binding sites and is required for insulin activation.
LXRα forms an active complex with retinoid X receptor α (RXRα) (18). Highly enriched in liver, LXRα has been shown to be activated by certain oxysterols and intermediates in the cholesterol synthetic pathway (19). Synthetic agonists of LXR are known to stimulate lipid synthesis in liver, mediated by activation of the SREBP-1c promoter (20). Along with activating SREBP-1c, LXRs perform many other regulatory functions in metabolism in a variety of tissues (21).
Members of the C/EBP family also perform many functions related to metabolism. Prominent is their ability to promote the differentiation of adipose tissue, an action mediated in part by their ability to activate the SREBP-1c gene (14). In 3T3 L1 adipocytes, C/EBPβ is known to bind to three sites in the SREBP-1c promoter (14). All of these sites are upstream of the of the 368-nucleotide promoter fragment that is sufficient for insulin induction of SREBP-1c transcription in liver (6), suggesting that C/EBPβ acts by a different mechanism in this organ.
Although C/EBPβ and LXRα have widespread effects on gene transcription, we are unaware of any previous studies showing a direct interaction between these proteins. The present study was facilitated by our development of a highly specific monoclonal antibody that precipitates LXRα with high efficiency. The specificity of the co-IP was confirmed by our finding that anti-LXRα did not coprecipitate C/EBPα. Moreover, it did not coprecipitate LIP, a version of C/EBPβ that retains the DNA-binding domain but lacks the transactivation domain (Fig. 2B).
The mechanism for complex formation between C/EBPβ and LXRα remains to be established. Our previous studies did not identify a potential C/EBPβ-binding site within the 368-bp region that is sufficient for insulin induction of SREBP-1c transcription. Moreover, our ChIP assays showed that the protein complex is precipitated together with a DNA fragment that contains the LXR-binding sites (Fig. 6). These data raise the possibility that LXRα binds to DNA and that C/EBPβ binds to LXRα without itself having to bind DNA. This hypothesis can be tested by producing versions of C/EBPβ with mutations that inactivate its DNA-binding domain and determining whether it still activates SREBP-1c transcription and whether it forms a complex with LXRα.
The C/EBPβ-LXRα complex was identified in fasted animals in which insulin levels were low, and it was not increased when the animals were fed and insulin rose (Fig. 2B). Moreover, the complex was attached to the promoter region in both fasted and fed conditions (Fig. 6). Although the complex was present in the fasted state, SREBP-1c transcription was low, indicating that the complex was not active. Insulin must activate the complex in some fashion, perhaps by stimulating the phosphorylation of one of its components, perhaps by recruiting a coactivator, or perhaps by providing a ligand for LXRα or its partner RXRα. All of these questions should be amenable to experimental solutions now that the C/EBPβ-LXRα complex has been identified.
Materials and Methods
Materials.
We obtained Sprague–Dawley rats from Harlan Laboratories; C57BL/6J mice from Jackson Laboratories; bovine insulin (catalog no. I6634), T0901317 (catalog no. T2320) dexamethasone, 3,3′,5-triiodo-l-thyronine, arachidonic acid, and PMSF from Sigma-Aldrich; calpain inhibitor I (ALLN) from AG Scientific; Lipofectin, Lipofectamine 2000, and Medium 199 from Invitrogen; PBS, DMEM, and RPMI 1640 from Mediatech; collagen I-coated dishes (catalog nos. 356400 and 356401) from BD Biosciences; FCS from Atlanta Biologicals; human Flag-C/EBPβ (catalog no. TP305882), human Flag-LXRα (catalog no. TP323767), and monoclonal anti-Flag (catalog no. TA100011) from OriGene; protease inhibitor mixture (cOmplete tablets) from Roche; Halt Phosphatase Inhibitor Cocktail from Thermo Fisher Scientific; monoclonal rabbit anti-rat C/EBPβ (catalog no. C2589-90E) from US Biologic; monoclonal rabbit anti–lysine-specific demethylase 1 (LSD1; catalog no. 2184), polyclonal rabbit anti-C/EBPα (catalog no. 2295), and polyclonal rabbit anti-mouse C/EBPβ (catalog no. 3087) from Cell Signaling Technology; and anti-mouse IgG (catalog no. NA934) and anti-rabbit IgG (catalog no. NA931) from GE Healthcare. LXRαβ−/− (KO) mice were obtained from Joyce Repa and David Mangelsdorf (7).
Generation of LXRα Monoclonal and Polyclonal Antibodies.
Recombinant LXRα-GST, produced in Escherichia coli transformed with pGEX-KG-LXRα encoding rat LXRα (RefSeq accession no. NM_031627), was purified on a glutathione column. Monoclonal anti-LXRα was prepared by fusion of SP2-IL6 mouse myeloma cells with splenic B lymphocytes obtained from BALB/c mice immunized with four injections of 25 μg of purified rat LXRα-GST mixed in Titermax Gold adjuvant (Sigma-Aldrich). Hybridoma culture supernatants were screened using ELISA procedures. One positive hybridoma, designated IgG-2B7, was subcloned by serial dilution three times. IgG-2B7 (subclass 1) was purified from hybridoma culture supernatant by affinity chromatography on protein G Sepharose 4 Fast Flow columns (GE Healthcare). A polyclonal LXRα antibody was prepared by s.c. injection of rabbits with 500 µg of the above antigen in incomplete Freund’s adjuvant, followed by biweekly injections of 250 µg. An IgG fraction of the immune serum was prepared by affinity chromatography as described above.
Insulin and LXR Agonists.
Stock solutions of 0.1 mM insulin were prepared in distilled water adjusted to pH 4.5 with glacial acetic acid, stored at 4 °C, and used within 3 mo. Stock solutions of 10 mM T0901317 (synthetic LXR agonist) were prepared in DMSO and stored at −20 °C.
Primary Rat Hepatocytes.
Primary rat hepatocytes were prepared as described previously (6). Male Sprague–Dawley rats (age 7–8 wk) were housed in animal colony cages, maintained on a reverse 12-h light/dark cycle (dark phase from 10:00 AM to 10:00 PM), and fed a Harlan Teklad Global Diet 2018 (Harlan Laboratories). Nonfasted rats were anesthetized with isoflurane 30 min before the dark cycle, and primary hepatocytes were isolated by the collagenase method (22) with modifications as described by Shimomura et al. (2). On day 0, the isolated hepatocytes were plated onto collagen I-coated 60-mm dishes (2 × 106 cells/dish) or six-well plates (5 × 105 cells/well) in medium A [DMEM supplemented with 5% (vol/vol) FCS, 100 U/mL sodium penicillin, and 100 µg/mL streptomycin sulfate]. The cells were incubated at 37 °C in 5% CO2. After 3–4 h, the attached cells were washed once with PBS, incubated for 14–16 h in medium B (Medium 199 supplemented with 100 nM dexamethasone, 100 nM 3,3,5-triiodo-l-thyronine, 100 units/mL sodium penicillin, and 100 µg/mL streptomycin sulfate) containing 1 nM insulin, and then used for transfection and siRNA knockdown experiments. All animal experiments were performed with approval of the Institutional Animal Care and Research Advisory Committee at University of Texas Southwestern.
siRNA Knockdown.
Triplicate wells of hepatocytes in a six-well plate (5 × 105 cells/well) were washed once with PBS, switched to 2 mL of serum-free medium B, transfected with 50 nM of synthetic double-stranded siRNAs against C/EBPβ (Invitrogen; catalog no. 4390771) using Lipofectamine 2000, and incubated in 5% CO2 at 37 °C. At 6 h after transfection, cells were washed once with PBS and incubated in medium B. After 14–16 h, cells were washed once with PBS, switched to medium B with or without 100 nM insulin, incubated for 6 h at 37 °C, and then were harvested for RNA analysis.
Quantitative Real-Time PCR.
Total RNA was prepared from rat hepatocytes or mouse liver and subjected to real-time PCR analysis. mRNAs for rat acidic ribosomal phosphoprotein 36B4 and mouse apolipoprotein B served as invariant controls for rat hepatocytes and mouse liver, respectively, as described previously (4). The primer sequences used for PCR are listed in Table 1.
Table 1.
Primer sequences for PCR: mRNA measurements
Gene (species) | Sequences for forward and reverse primers (5′ to 3′) |
36B4 (rat) | Forward: TTCCCACTGGCTGAAAAGGT |
Reverse: CGCAGCCGCAAATGC | |
SREBP-1c (rat) | Forward: GACGACGGAGCCATGGATT |
Reverse: GGGAAGTCACTGTCTTGGTTGTT | |
C/EBPβ (rat) | Forward: AAGCTGAGCGACGAGTACAAGA |
Reverse: GTCAGCTCCAGCACCTTGTG | |
C/EBPα (rat) | Forward: GGTTTAGGGTCGCTGGATCTC |
Reverse: GGCGACACCAGAATCTCCTAGT | |
ABCA1 (rat) | Forward: GGACACCAGCATTAGGGACAT |
Reverse: TGGGATGCTTGATCTGCTGTA | |
SREBP-1c (mouse) | Forward: GGAGCCATGGATTGCACATT |
Reverse: GGCCCGGGAAGTCACTGT | |
ApoB (mouse) | Forward: CGTGGGCTCCAGCATTCTA |
Reverse: TCACCAGTCATTTCTGCCTTTG |
Primer sequences were custom synthesized by Integrated DNA Technologies.
Fasting and Refeeding Protocol for Rats and Mice.
Male Sprague–Dawley rats (age 3–4 mo) and male C57BL/6J mice (age 6–8 wk) were used in these experiments. The animals were divided into two groups: fasted and refed. For rats, the fasted group was fasted for 48 h, and the refed group was fasted for 48 h and then refed for 6 h with a 61.2% carbohydrate, fat-free diet (MP Biomedicals; catalog no. 960238). For mice, the fasted group was fasted for 12 h, and the refed group was fasted for 12 h and then refed for 12 h with the same high-carbohydrate, fat-free diet.
Nuclear Extract Preparation.
Nuclear extracts from fasted and refed rats were prepared using a sucrose cushion at the bottom of the centrifuge tube (23). Isolated rat livers were homogenized at 4 °C in buffer A [10 mM Hepes pH 7.6, 25 mM KCl, 1 mM sodium EDTA, 2 M sucrose, 10% (vol/vol) glycerol, 0.15 mM spermidine, 2 mM spermindine, 1 mM PMSF, 1 mM DTT, 0.5 mM Pefabloc, 10 µg/mL leupeptin, 5 µg/mL pepstatin, 25 µg/mL ALLN, and 10 µg/mL aprotinin]. The homogenate was laid over of buffer A in an ultracentrifuge tube and spun at 1 × 105g for 1 h at 4 °C. The resulting nuclear pellet was resuspended in buffer B [10 mM Hepes pH 7.6, 100 mM KCl, 2 mM MgCl2, 1 mM sodium EDTA, 1 mM DTT, 10% (vol/vol) glycerol, 1 mM PMSF, 0.5 mM Pefabloc, 10 µg/mL Leupeptin, 5 µg/mL pepstatin, 25 µg/mL ALLN, 10 µg/mL aprotinin, 1 tablet cOmplete per 10 mL, and 1% (vol/vol) Halt Phosphatase Inhibitor]. Ammonium sulfate was added to the resuspended lysate to a final concentration of 0.4 M, after which the mixture was incubated for 45 min on a rotator at 4 °C. Each mixture was spun at 3 × 105g for 45 min at 4 °C, after which the supernatant was transferred to a fresh tube for co-IP experiments.
Co-IP.
The LXRα co-IP experiments were performed with monoclonal LXRα antibody IgG-2B7 using a co-IP kit from Thermo Scientific (catalog no. 26149). The LXRα antibody-conjugated resin was prepared according to the manufacturer’s instructions. Pooled liver nuclear extracts from four rats (~1 mg protein in 0.1 mL) were then precleared with control agarose resin, added to 50 µL of the antibody-immobilized resin, and incubated with gentle end-over-end mixing for 14–16 h in buffer B at 4 °C. The precipitated pellets were washed with 0.4 mL of buffer B six times (10 min for each wash), eluted with 20 µL of SDS/PAGE sample buffer, and then subjected to SDS/PAGE on 4–12% gradient gels (Invitrogen), followed by immunoblotting.
Blue Native PAGE for Detection of C/EBPβ-LXRα Complex.
Blue native PAGE was carried out using the NativePAGE Bis-Tris Gel System from Invitrogen. Various amounts of purified LXRα and C/EBPβ were premixed and incubated with gentle rotation for 2 h at 4 °C. The protein solutions were then mixed with NativePAGE Sample Buffer and loaded onto NativePAGE Novex 4–16% Bis-Tris gels. After electrophoresis, proteins were transferred electrophoretically to a Hybond-C extra nitrocellulose membrane and subjected to immunoblot analysis with anti-Flag.
ChIP Assay.
These assays were performed using a ChIP assay kit containing protein A Agarose/Salmon Sperm DNA resin (protein A resin) (Upstate Biotechnology). Portions of male rat liver were sliced into ~0.5-mm-thick fragments. Each slice (70 mg) was incubated with 1% (wt/vol) formaldehyde at room temperature for 10 min to cross-link proteins to DNA. The tissue was sonicated six times for 10 s at 4 °C to disrupt the cells and shear the DNA. After centrifugation, the soluble chromatin solution was precleared by precipitation with the protein A resin. The supernatant was incubated with the indicated antibody (Fig. 5) at 4 °C for 14–16 h, after which the mixture was incubated with the protein A resin for 2 h at 4 °C. The precipitated protein-DNA complexes were washed and eluted with the buffers provided by the manufacturer. The eluted DNA was treated with proteinase K (Ambion) at 45 °C for 30 min, followed by incubation with 0.2 M NaCl at 65 °C for 4 h, extraction with phenol-chloroform-isoamylalcohol, and ethanol precipitation. The purified DNA was subjected to PCR using the primers shown in Table 2 and then visualized after electrophoresis on a 1.5% agarose gel.
Table 2.
Primer sequences for PCR: ChIP assays
Primer | Sequences for forward and reverse primers (5′ to 3′) |
Primer 1 | Forward: ATTCCGAACCCAGGCACTT |
Reverse: CAGCAGCTCGGGTTTCAC | |
Primer 2 | Forward: TTGGGCTGTTTCTCACCTCC |
Reverse: GGCAATTTCCACTGTCCCTT | |
Primer 3 | Forward: ATGGAGGGATCTCACTGTACCT |
Reverse: GAGAATAGTGACATCGGCAGAG |
Primer sequences were custom synthesized by Integrated DNA Technologies.
For the experiment with sequential ChIP, we used the protocol described by Furlan-Magaril et al. (24). After the first immunoprecipitation with either anti-C/EBPβ or anti-LXRα, the pellets were incubated with buffer containing TrisHCl pH 8, 1 mM sodium EDTA, and 10 mM DTT to denature the antibody and release the protein-DNA complex. After removal of the protein A resin by centrifugation, the supernatant solution was diluted 20-fold to lower the DTT concentration below the threshold for antibody denaturation. This was followed by the addition of anti-LXRα, anti-C/EBPβ, or control IgG, and overnight incubation at 4 °C. After precipitation with protein A resin, the protein-DNA complexes were eluted by incubation with 1% (wt/vol) SDS and 0.1 M NaHCO3. The proteins were released from the DNA by treatment with proteinase K, and the DNA was analyzed by PCR using the primers listed in Table 2.
Adenovirus-Mediated C/EBPβ Knockdown in Mice.
Recombinant adenovirus clones expressing C/EBPβ shRNA were generated with BLOCK-iT Adenoviral RNAi kits (Invitrogen), including the Block-iT Adenoviral RNAi expression system and the pAd/BLOCK-iT-DEST RNAi Gateway Vector kit. These plasmids and a control plasmid expressing β-galactosidase (LacZ) were transfected into HEK-293A cells with Lipofectamine 2000. The resulting adenoviruses were amplified in HEK-293A cells and purified by cesium chloride density centrifugation.
Male C57BL/6J mice (age 6–8 wk) housed in colony cages under standard 12-h light/12-h dark cycles were injected via the tail vein with 1 × 1011 particles of purified adenoviruses diluted in PBS. At 3 d after injection, these mice were used for fasting-refeeding studies.
Reproducibility.
All experiments were carried out on two or more occasions on different days, with similar results.
Acknowledgments
We thank Guosheng Liang and Shijie Li for helpful suggestions; Joyce Repa, Steven Kliewer, and David Mangelsdorf for providing the LXRαβ−/− mice and for critical comments on the manuscript; Christina Li, Linda Donnelly, Angela Carroll, and Jeff Cormier for excellent technical assistance; and Lisa Beatty for invaluable help with tissue culture. This work was supported by NIH Grant HL20948 and the Moss Heart Foundation. J.T. was the recipient of a postdoctoral fellowship from the American Diabetes Association.
References
Articles from Proceedings of the National Academy of Sciences of the United States of America are provided here courtesy of National Academy of Sciences
Full text links
Read article at publisher's site: https://doi.org/10.1073/pnas.1608987113
Read article for free, from open access legal sources, via Unpaywall:
https://www.pnas.org/content/pnas/113/29/8182.full.pdf
Citations & impact
Impact metrics
Citations of article over time
Alternative metrics
Article citations
Team players in the pathogenesis of metabolic dysfunctions-associated steatotic liver disease: The basis of development of pharmacotherapy.
World J Gastrointest Pathophysiol, 15(4):93606, 01 Aug 2024
Cited by: 0 articles | PMID: 39220834 | PMCID: PMC11362842
Review Free full text in Europe PMC
Lactobacillus rhamnosus NKU FL1-8 Isolated from Infant Feces Ameliorates the Alcoholic Liver Damage by Regulating the Gut Microbiota and Intestinal Barrier in C57BL/6J Mice.
Nutrients, 16(13):2139, 04 Jul 2024
Cited by: 0 articles | PMID: 38999886
The cellular and molecular targets of natural products against metabolic disorders: a translational approach to reach the bedside.
MedComm (2020), 5(8):e664, 24 Jul 2024
Cited by: 1 article | PMID: 39049964 | PMCID: PMC11266934
Review Free full text in Europe PMC
Bioelectric medicine: unveiling the therapeutic potential of micro-current stimulation.
Biomed Eng Lett, 14(3):367-392, 11 Mar 2024
Cited by: 0 articles | PMID: 38645592
Review
Effect of FTY720P on lipid accumulation in HEPG2 cells.
Sci Rep, 13(1):19716, 12 Nov 2023
Cited by: 1 article | PMID: 37953311 | PMCID: PMC10641067
Go to all (42) article citations
Data
Similar Articles
To arrive at the top five similar articles we use a word-weighted algorithm to compare words from the Title and Abstract of each citation.
BHLHE40, a third transcription factor required for insulin induction of SREBP-1c mRNA in rodent liver.
Elife, 7:e36826, 27 Jun 2018
Cited by: 15 articles | PMID: 29952285 | PMCID: PMC6023608
Central role for liver X receptor in insulin-mediated activation of Srebp-1c transcription and stimulation of fatty acid synthesis in liver.
Proc Natl Acad Sci U S A, 101(31):11245-11250, 20 Jul 2004
Cited by: 348 articles | PMID: 15266058 | PMCID: PMC509189
Insulin activates the rat sterol-regulatory-element-binding protein 1c (SREBP-1c) promoter through the combinatorial actions of SREBP, LXR, Sp-1 and NF-Y cis-acting elements.
Biochem J, 385(pt 1):207-216, 01 Jan 2005
Cited by: 92 articles | PMID: 15330762 | PMCID: PMC1134689
SREBP-1c transcription factor and lipid homeostasis: clinical perspective.
Horm Res, 68(2):72-82, 05 Mar 2007
Cited by: 212 articles | PMID: 17344645
Review
Funding
Funders who supported this work.
HHS | National Institutes of Health (1)
Grant ID: HL20948
Moss Heart Foundation (1)
Grant ID: None
NHLBI NIH HHS (1)
Grant ID: P01 HL020948
The American Diabetes Association Research Foundation (1)
Grant ID: None