Abstract
Free full text
Regeneration versus scarring in vertebrate appendages and heart
Abstract
Injuries to complex human organs, such as the limbs and the heart, result in pathological conditions, for which we often lack adequate treatments. While modern regenerative approaches are based on the transplantation of stem cell‐derived cells, natural regeneration in lower vertebrates, such as zebrafish and newts, relies predominantly on the intrinsic plasticity of mature tissues. This property involves local activation of the remaining material at the site of injury to promote cell division, cell migration and complete reproduction of the missing structure. It remains an unresolved question why adult mammals are not equally competent to reactivate morphogenetic programmes. Although organ regeneration depends strongly on the proliferative properties of cells in the injured tissue, it is apparent that various organismic factors, such as innervation, vascularization, hormones, metabolism and the immune system, can affect this process. Here, we focus on a correlation between the regenerative capacity and cellular specialization in the context of functional demands, as illustrated by appendages and heart in diverse vertebrates. Elucidation of the differences between homologous regenerative and non‐regenerative tissues from various animal models is essential for understanding the applicability of lessons learned from the study of regenerative biology to clinical strategies for the treatment of injured human organs. © 2015 The Authors. The Journal of Pathology published by John Wiley & Sons Ltd on behalf of Pathological Society of Great Britain and Ireland.
Introduction
Restoration of tissue integrity following injury is a fundamental property of multicellular organisms. Animals respond to traumatic organ loss either by reparative sealing of the wound or by regeneration. Non‐regenerative healing often involves synthesis of a scar to repair the interrupted continuity of the organ without reproduction of the missing tissue. In contrast, regeneration recreates the architecture and function of the damaged body part, either without scarring or with transient fibrosis. This process depends on cellular plasticity, accessibility to developmental programmes and functional integration of newly formed tissues with the pre‐existing organ remnants. Despite extensive research in various model animals, it remains poorly understood what specific factors predispose for non‐regenerative repair versus complete reconstruction of complex vertebrate organs, such as limbs and heart 1, 2, 3, 4, 5, 6, 7.
In humans, injury followed by inadequate regeneration and scarring is a common pathological mechanism. This is the case for an irreversible limb loss and for a heart infarct. By contrast, certain non‐mammalian adult vertebrates, such as zebrafish, newts and axolotls, can reconstitute fully functional appendages and a cardiac ventricle 6, 8, 9, 10, 11, 12, 13, 14, 15, 16. These animals provide unique in vivo model systems to understand how damaged mature organs are naturally capable of a rapid switch between resting and re‐growing phases. A great deal of attention has been dedicated to the molecular mechanisms of regeneration, as discussed in many recent reviews 10, 11, 12, 17, 18, 19, 20, 21, 22, 23, 24, 25, 26, 27. Here we focus on the cellular and anatomical features of appendage and heart regeneration in anamniotic vertebrates (fish and amphibians) and how this information is relevant for the goals of regenerative medicine.
Regeneration versus scarring from the functional and adaptive perspective
The absence of an appendage, such as a limb or a tail, impedes efficient locomotion, resulting in a decreased survival rate. Myocardial infarction disrupts the performance of the blood‐pumping organ, leading to a life‐threatening condition. Given that the organism survived the initial trauma, complete organ regeneration intuitively provides an advantage to regain fitness after injury. Ironically, lower vertebrates possess an innate ability to restore their damaged appendages and the heart, while mammals lack this capability. This uneven phylogenic distribution of regeneration could be due to various proximate constraints of anatomical, physiological and molecular nature, such as the high complexity of the mammalian organs, a cytokine profile of inflammatory cells and inaccessible morphogenetic information, which arose as adaptive traits or as side‐effects of other evolutionary changes 2, 23, 28, 29, 30. Among many concepts, the classic hypothesis proposes that the regenerative capability declines whenever the lost part of organ becomes absolutely indispensable for survival:
To qualify for replacement, a structure … must be important enough to be missed when it is gone, but not so vital that an animal cannot survive its loss long enough to grow a replacement 31.
Accordingly, the loss of limb regeneration in the evolution of vertebrates may be associated with the move onto land, when limbs become indispensable for locomotion, much more than when an animal is suspended in water (Figure1A). The limbs of terrestrial animals have an essential function to carry the body mass while remaining in direct contact with rough ground, which might biophysically preclude regenerative outgrowth formation from the stump 25. Among urodeles, the efficiency and fidelity of regeneration become drastically lower in land‐phase animals as compared to water‐phase forms 32, 33. The adult aquatic urodeles, eg newts and paedomorphic axolotls, can complete regeneration within 30–60 days after amputation, whereas the terrestrial forms, eg certain salamanders and postmetamorphic axolotls, require 200–400 days 32, 33, 34. In addition, a proper terrarium with wet and soft substrates is a prerequisite for limb regeneration in the land‐phase urodeles, as adverse environmental conditions impair this process 32, 35. It is possible that during the evolution of terrestrial amniotic vertebrates, beginning with reptiles, the limb regenerative capability has been replaced with scarring as a more suitable strategy for repairing stumps while walking on rough land. Nevertheless, some degree of appendage restoration can evolve locally, exemplified by the gain of digit tip regeneration in mice and humans 36, 37, 38.

Potential impact of external and internal milieux on the regenerative feasibility of vertebrate appendages and heart. (A) The transition onto land coincides with the reduction of limb‐regenerative capability. Fish and aquatic urodeles possess the ability to completely and efficiently reproduce amputated appendages. Postmetamorphic terrestrial frogs and toads completely lack this capability, while aquatic Xenopus froglets are capable of heteromorphic regeneration of a cartilaginous 'spike' from the amputation plane. Amniotic vertebrates, ie reptiles, birds and mammals, which adapted to life and reproduction on the land, display no limb regeneration. A certain capacity of appendage restoration can be regained, as exemplified by digit tip regeneration in mice and humans under certain circumstances. (B) The rise in cardiac workload in endothermic vertebrates correlates with compaction of the myocardial wall and elaboration of the coronary vasculature; schematic representation of heart anatomy with a transverse section of the ventricle in fish (left) and mammals (right). In the poikilothermic vertebrates, ie fish, amphibians and reptiles, the ventricle is a trabecular, sponge‐like chamber. This architecture allows for oxygenation of ventricular cardiomyocytes directly from blood flowing through many miniscule cavities of the trabecular wall. The outer compact myocardial layer is typically thin, with fine coronary vasculature. Among animals with the trabecular heart, zebrafish, newts and axolotls display heart regeneration. A radical change of cardiac anatomy is observed in homeothermic mammals and birds, in which the ventricles are composed predominantly of a compact myocardium and a discrete central lumen. Oxygenation of cardiomyocytes is dependent on coronary circulation. This architecture has been associated with no regenerative capabilities
Although the heart is obviously a life‐essential organ, a partial loss of cardiac muscle can be differentially tolerated among vertebrates. Among fish, medakas were reported to react with high lethality and health impairment to ventricular surgery in comparison to zebrafish 39. Zebrafish can generally survive genetic ablation of 60% of cardiomyocytes or damage to 20% of the ventricle 40, 41, 42, 43, 44, 45, 46, which would have fatal consequences in adult mammals 47. Thus, a portion of the myocardium can be of low/intermediate importance for the global function of the heart in certain vertebrates. In the line of the above‐cited hypothesis, proposed by Goss 31, 48, such animals might have a better predisposition for cardiac regeneration than mammals, especially in a milieu of low metabolism and with a trabecular myocardium typical of poikilotherms. In poikilothermic vertebrates, a significant portion of cardiomyocyte oxygen supply derives from the blood flow in cavities of the ventricular chamber. This is in contrast to the compact myocardium of homeothermic mammals, which depends strongly on coronary circulation 49, 50, 51 (Figure1B). Indeed, the thickness of the mammalian compact myocardium prevents gas diffusion from luminal blood to subepicardial cardiomyocytes, a mechanism that could possibly attenuate the damage during left ventricular ischaemia. The trabecular architecture, such as in zebrafish and newt heart, may account for a permissive environment for a regenerative response at the site of injury 11, 52. Mammals, which are homeothermic animals, require a higher performance of the blood‐pumping organ. Remarkably, the ventricular blood pressure in adult zebrafish reaches the value of approximately 2.5
mmHg 53, as compared to an average of 120
mmHg in adult humans and mice. It is possible that the biomechanical properties of the ventricular wall and the mode of oxygen supply might limit the choice of the tissue type to restore the interrupted myocardium, while the heart has to beat continuously during the healing process.
Studies of anamniotes revealed that the basic regenerative principles are similar in the appendages and the heart 3, 6, 9. Nevertheless, specific cellular programmes are poorly comparable between the appendages and the heart, as these body parts are built with different 'blocks'. Therefore, we discuss the current knowledge on regeneration of these two distinct organs in separate sections.
Appendage: the blastema and the pro‐regenerative environment
Complete restoration of the amputated vertebrate appendage, called epimorphic regeneration, involves the creation of two key structures, a blastema and a wound epithelium 21, 54. Importantly, neither is fundamentally different from the stump tissues. The wound epidermis derives from the migrating epithelial cells at the site of injury, whereas the blastema arises by the conversion of local non‐dividing cells into lineage‐restricted proliferating cells, which give rise to the outgrowth 55, 56, 57, 58, 59, 60. To date, there is no evidence for the involvement of remote cell sources. To initiate epimorphic regeneration, the participating cells change gene expression and reactivate developmental signalling pathways to guide cell dedifferentiation, proliferation and migration, as recently reviewed 10, 12, 17, 18. The wound epidermis serves not only for healing of the interrupted organ, but also forms a specialized layer that acts as a signalling centre by producing cytokines, such as Wnts, Fgfs and Shh 17, 18. The reciprocal communication between the wound epidermis, nerves and the underlying tissues is a prerequisite for appendage regeneration 17, 18, 61, 62, 63. The interruption of epithelial–mesenchymal interaction by covering the amputation plane with a mature skin graft prevents regeneration 64, 65. A contribution of the wound epidermis and the mesenchymal blastema has been observed during digit tip regeneration in mice and humans, suggesting parallel regenerative strategies between diverse vertebrates 36, 66. In humans, it is assumed that dermal scarring and the inability to form the blastema are responsible for the lack of limb regeneration. Thus, the mechanisms regulating wound healing and the plasticity of mature cells are of central interest in the field of regenerative biology and medicine.
Blastema formation is associated with cellular dedifferentiation of specialized appendage tissues, such as bone‐forming osteoblasts in zebrafish 56, 57, 58, 59, 60, 67, 68 and skeletal muscles in urodeles 69, 70, 71, 72. However, dedifferentiation is not the only mechanism for the generation of new cells for both of these specialized tissues. In the fin, genetic ablation of all osteoblasts, using a nitroreductase system, does not prevent bone regeneration, suggesting a possible secondary source of osteoblasts under certain restrictive conditions 59. Although myofibre dedifferentiation occurs during urodele limb restoration, Cre–loxP genetic fate mapping in the axolotl revealed that new muscle mainly originates from endogenous Pax7‐positive satellite cells 69. These examples indicate a conditional plasticity and a species‐specific variability of possible sources of cells for appendage regeneration.
By contrast to bone and muscle tissues, dermal fibroblasts do not appear to dedifferentiate markedly during regeneration, as they are originally at a relatively low cytospecialization level. Instead, the connective tissue undergoes disorganization and remodelling of the extracellular matrix (ECM) to facilitate fibroblast proliferation and migration. In urodeles, the population of mesenchymal cells is highly over‐represented in the blastema in comparison to other cell types 73. Moreover, mesenchymal cells are the pioneers promoting the protrusion of the regenerative outgrowth. This suggests that dermal fibroblasts play a leading role in appendage regeneration. Thus, it is important to identify the structural differences of dermal tissue between blastema‐forming versus scar‐forming model organisms.
As the skin acts as a protective barrier, the surface of the body in terrestrial vertebrates underwent specialization from mucogenesis to cornification, while the dermis shifted toward a more robust collagen fibre‐rich matrix (Figure2A, F). In most mammals, cutaneous injuries heal by scarring. In contrast, the surface of wet anatomical sites in humans, such as the oral mucosa, is capable of scar‐free wound closure, which is associated with a higher proliferative capacity of local keratinocytes and fibroblasts, beneficial growth factor production and appropriate matrix deposition, as compared to skin 74. Based on these observations, it can be proposed that cellular specialization between homologous structures may explain the regenerative differences, even in the same organism.
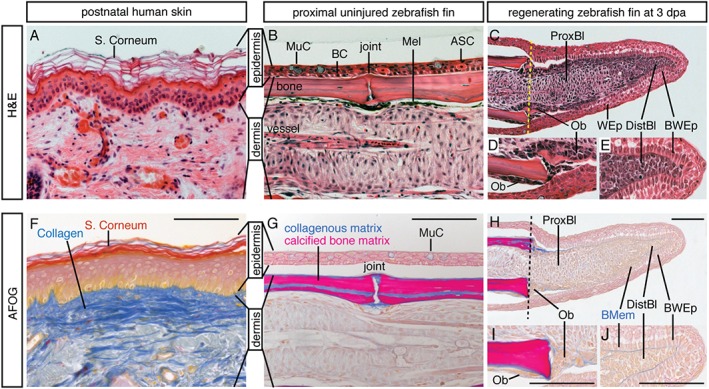
Histological comparison reveals excessive collagenous matrix in human dermis as compared to mesenchyme of the fin. Postnatal human skin (section of a cheek) and zebrafish fin (longitudinal section of a ray) were incubated with the same histological staining solutions. (A–E) Haematoxylin (purple‐brownish, nuclear marker) and eosin (orange, protein marker) (H&E) staining. (A) The multilayered epidermis of human skin comprises a stratum corneum with anuclear keratinocytes; the dermis is rich in ECM that is produced by loosely distributed fibroblasts. (B) The stratified epidermis of the fin is composed of only a few cell layers and lacks a stratum corneum; it contains a basal cell layer (BC) and specialized epithelial cells, such as mucous cells (MuCs) and alarm‐substance cells (ASCs); the dermis of the fin ray comprises segmented bone produced by osteoblasts and vascularized mesenchymal tissue; Mel, melanocytes. (C–E) A fin regenerate at 3 days post‐amputation (dpa); the regenerate is located to the right of the amputation plane (dashed line); wound epidermis (WEp) surrounds the outgrowth; the basal wound epithelium (BWEp) contains cuboidal cells at the level of the proximal blastema (Prox.Bl) or columnar cells at the level of the distal blastema (Dist.Bl). (D) Higher magnification of regenerating bone with osteoblasts (Ob). (E) The cells of the distal blastema are tightly packed under the wound epithelium, with little intercellular space. (F–J) Aniline blue, acid Fuchsin and Orange G (AFOG) staining reveals collagen or mucous in blue, cornified epidermis in red, mineralized bone matrix in magenta and cytoplasm in orange; histological procedures were performed in parallel on human skin and fin sections. (F) Dermis of human skin contains a high amount of ECM with collagen fibrils. (G) The dermal bone of the fin is mineralized (magenta); a thin layer of collagen (blue) covers the bone surface, the bone canal and joints; dermal fibroblasts of the ray do not deposit extensive collagen fibres, consistent with the mesenchymal character of this tissue. (H–J) A section adjacent to the specimen shown in (C–E): collagen is detected in the basement membrane (BMem) and around the osteoblasts (Ob). Scale bars=
100
µm
The zebrafish fin is a non‐muscularized dermal fold that encompasses multiple bony rays spaced by soft interrays 10. The stratified epidermis contains only a few cell layers, while the dermis is subdivided into two compartments: (a) dermal bone, produced by osteoblasts; and (b) a network of fibroblasts with little collagenous matrix (Figure2B, G). The dermal bone is located directly underneath the basal layer of the epidermis, suggesting a contact between both tissues. Remarkably, a structural connection between the bone and the epidermis has been reported in the regenerative part of the mammalian limb, viz. in the distal tip of the last phalangeal element (P3) in mice 36. The significance of the link between the bone and epidermis during appendage regeneration in vertebrates remains to be determined. In aquatic urodeles, the dermis contains relatively few collagen fibrils, especially in its subepidermal layer 75, 76. Mammals with exceptionally elastic skin and little fibrillar collagen, such as the African spiny mouse, can also regenerate large skin holes perfectly 77. These examples suggest a correlation between a low content of collagen fibrils and regenerative success. Thus, a dense accumulation of fibrous matrix in the human dermis could cause one of the barriers for intercellular communication and tissue plasticity, which are essential factors for organ regeneration.
The fin and urodele limb initiate regeneration remarkably quickly, as the re‐epithelialization and local disorganization of mesenchyme occurs within the first 12h post‐amputation 75, 76, 78. During this time, tenascin C and matrix metalloproteinases become up‐regulated to stimulate tissue remodelling 79, 80. In zebrafish, a regenerative outgrowth becomes clearly visible at 3 days post‐amputation (dpa) (Figure
2C, H). The apical zone is considered to be the upstream signalling organizer of the regenerate, which includes a columnar wound epithelium and mesenchymal progenitor cells 81 (Figure
2E, J). The proximal part of the blastema contains highly proliferative mesenchymal cells and regenerating osteoblasts that initiate the deposition of new bone matrix in a proximal–distal direction of the outgrowth 56 (Figure
2D, I). The progression of regeneration depends on a balance between proliferation and redifferentiation, which is regulated by a combination of multifunctional signalling pathways, such as FGF, Wnt, TGFβ, Activin, BMP, IGF, Notch, Shh, retinoic acid (RA) and Hippo 9, 17, 68, 82, 83, 84, and epigenetic mechanisms 85. Depending on the temperature, fin regeneration is completed approximately 3 weeks after injury 10, 86. It is still poorly understood how the amputation event triggers the programme that generates the specialized wound epithelium and the blastema from the stump tissues. At least, the histological comparison of the dermis between intact and regenerating fins suggests an absence of extracellular obstacles, such as excessive collagen fibres, which could potentially impede the execution of the intrinsic regenerative programme.
What is the particular function of fibroblasts during blastema formation? Grafting experiments in urodeles demonstrated an inductive role of dermal fibroblasts during reconstruction of the appropriate structure along the body axis 24, 87. A juxtaposition of cells from the proximal and distal blastemas elicits a segregation of cells according to their original source. In some instances, the positional discontinuity between cells stimulates regrowth of the missing intermediate structures, resulting in intercalary regeneration of an ectopic appendage that bridges the gap between the positional disparities 88, 89. Notably, transcriptome analyses provide evidence that positional memory, which was established during embryogenesis, is also present in mature mammalian fibroblasts 90, 91. It will be essential to elucidate how the coordinates are communicated between cells to guide pattern formation during regeneration. Although cell transplantation has been practised in regenerative medicine, the key unsolved problem is the creation of such regeneration‐competent progenitor cells that can recognize the position in the stump, integrate with the host organ and/or act as 'pattern‐forming' cells. The fin and amphibian limb regeneration models can provide some hints on how to establish a regeneration‐competent environment and 'plasticizing' agents to enhance survival, intercellular communication and integration of the transplanted cells into the stump.
Heart: cardiomyocyte plasticity in vertebrates
The zebrafish heart can fully regenerate within 30–60 days, either after removal of up to 20% of the ventricle 92, after cardiomyocyte‐specific genetic ablation that causes the loss of up to 60% of the myocardium 40, 41, 42, 43 or after cryoinjury‐induced cardiac infarction of 20–25% of the ventricle 44, 45, 46 (Figure3). In the newt, complete myocardial restoration has been observed within 2–7 months after ventricular apex amputation or mechanical disruption of
>
50% of the ventricle 8, 52, 93, 94, 95, 96. Cardiac regeneration has been reported in axolotls 3 months after ventricular resection 15, 16. These findings have raised several fundamental questions about the origin of the new myocardium and signalling pathways involved in this process and, importantly, whether these findings are transposable to humans.
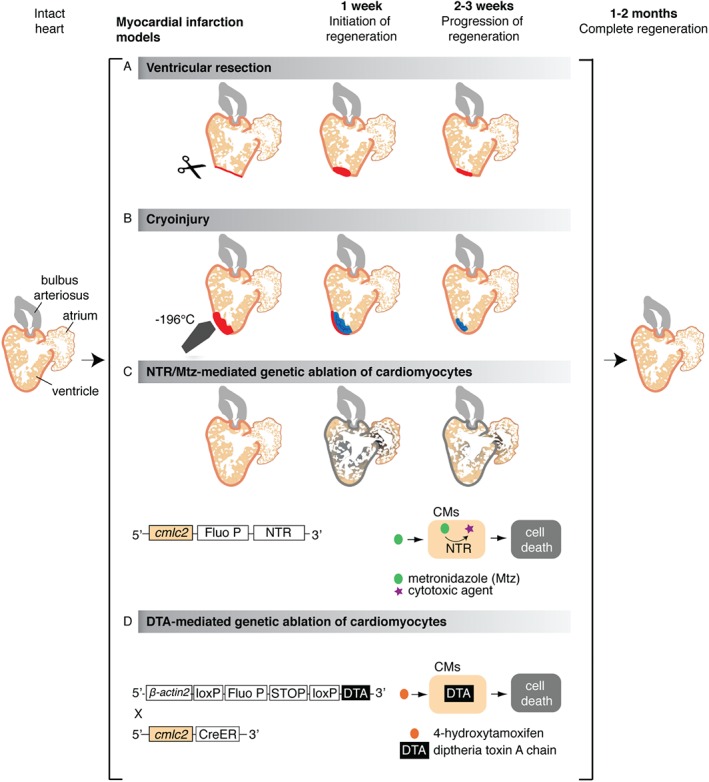
Cardiac injury models in zebrafish. Schematic representation of different experimental procedures to induce cardiac damage in the zebrafish; illustration shows a longitudinal section of the heart. (A) The ventricular resection method was developed in 2002 92; in this model, the apex of the ventricle (about 20% of the heart volume) is amputated with scissors. (B) The cryoinjury procedure was established in 2011 44, 46, 182, 183 and is based on rapid freezing–thawing of the cardiac tissue by the application for 20–25s of a stainless steel cryoprobe precooled in liquid nitrogen; as a result, massive cell death occurs in the frozen area (about 20% of the heart volume). (C) The nitroreductase (NTR)/metronidazole (Mtz) genetic ablation system enables depletion of specific cardiac cell types in an inducible manner 40, 42, 43, 149; in this method, first applied to zebrafish in 2007 43, 184, a bacterial nitroreductase fused to a fluorescent protein (Fluo P) is expressed under the control of a tissue‐specific promoter, such as the cardiac myosin light chain 2 (cmlc2), which is specific for cardiomyocytes (CMs) 43, 185. This enzyme catalyses the conversion of the prodrug metronidazole into a cytotoxic agent, thereby inducing cell death in the NTR‐expressing cells. (D) The Cre/loxP transgenic system, developed by Wang and colleagues in 2011, is based on the inducible expression of diphtheria toxin A chain (DTA) in cardiomyocytes (CMs) 41; in brief, this procedure is based on the use of a double transgenic system, including Tg(βactin2:loxP‐Fluo P‐STOP‐loxP‐DTA), which contains an inducible cytotoxic DTA gene preceded by a loxP‐flanked reporter gene and stop codon, and the Tg(cmlc2:CreER) 98, which allows expression of the 4‐hydroxytamoxifen (4‐HT)‐inducible Cre recombinase in cardiomyocytes. Treatment of the intercrossed fish cmlc2:CreER;βactin2:loxP‐Fluo P‐STOP‐loxP‐DTA results in a massive myocardial cell depletion (up to 60% of the ventricle)
Similarly to mammals, there is no evidence of regeneration‐competent cardiac progenitor cells in the post‐embryonic stages in lower vertebrates. In zebrafish, genetic fate‐mapping experiments with Cre–loxP transgenic lines have demonstrated that the regenerated myocardium is derived from pre‐existing adult cardiomyocytes 97, 98. Consistently, different proliferative assays based on the detection of G1–S‐phase or mitotic markers have revealed an accumulation of proliferating cardiomyocytes in injured myocardium 44, 45, 92, 97, 98, 99. In newts, experiments demonstrating the origin of the new cardiac muscle are still missing. However, evidence of the cell‐cycling activity in endogenous cardiomyocytes after injury strongly suggests a regenerative mechanism similar to that in zebrafish 8, 96.
The main question is why adult cardiomyocytes can multiply to restore a damaged myocardium in fish and urodeles, but not in mammals. Obstacles for cell‐cycle entry can exist at the molecular, cellular and organismic levels. During development, cardiomyocytes are functional as contractile cells but remain at a low differentiated state that is compatible with cell division and morphogenesis 26, 100. After the completion of development, cardiomyocytes differentiate and adopt a mature cytoarchitecture, which can exhibit different properties in distinct species. This developmental transition has been associated with a reduction of proliferative potential in higher vertebrates, but not in zebrafish and newts. In mammals, the proliferative capacity becomes dramatically limited at birth, when the heart switches from a hyperplastic to a hypertrophic mode of growth 101. Accordingly, studies in mice and rats have demonstrated that the regenerative capacity is restricted to 1 week of life 102, 103, 104. In contrast to rodents, sheep have already undergone hypertrophic transition 10 days before birth 105. Thus, myocardial maturation follows a distinct temporal frame in altricial and precocial non‐primate mammals, suggesting a possible correlation between cardiomyocyte differentiation and the increased heart workload associated with locomotion. In humans, the enlargement of cardiac cells during early childhood is accompanied by remarkable cardiomyocyte proliferation 106, which contrasts with the rapid perinatal switch from proliferation to binucleation/hypertrophy in other mammalian species.
Adult mammalian cardiomyocytes become enlarged and more complex compared to their perinatal form (Figure4A, B). The tightly packed myofibrils are separated by invaginations of the plasma membrane that form transverse T‐tubules 107, 108, 109. Cardiac cytospecialization is less advanced in fish and urodeles, whereby cardiomyocytes form a rod shape but remain relatively thin (ca. 5
µm) and lack T‐tubules 110, 111, 112 (Figure
4C). In vitro and in vivo experiments have demonstrated that such adult cardiomyocytes can undergo cell division (Figure
4C) 96, 111. Despite these morphological differences, action potential characteristics of cardiomyocytes in zebrafish closely resemble those in mammals 110, 113. While the majority of adult cardiomyocytes in fish are mononucleated/diploid, the rodent heart contains predominantly binucleated/diploid cardiomyocytes, whereas the majority of human cardiac cells remain mononucleated/polyploid (Figure
4D) 11, 27, 104, 114, 115, 116. Thus, the degree of cardiomyocyte cytospecialization varies across species, probably in correlation with the specific organismic demands. In primates, it is possible that a transition from tetra‐ to bipedal locomotion with vertical blood pumping might have imposed polyploidy as an adaptation for the increase in myocyte size 117.
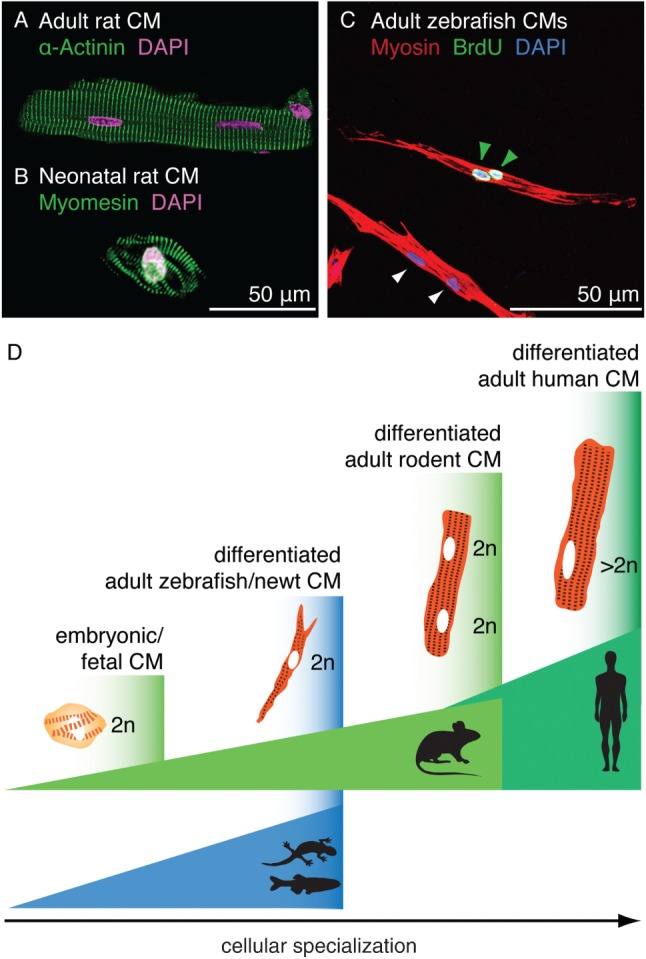
Comparison between mammalian and zebrafish cardiomyocytes in vitro. (A) Image of an isolated adult rat cardiomyocyte stained with DAPI (false‐coloured pink) and antibody against α‐Actinin (green, Z‐disc). (B) Image of an isolated neonatal rat cardiomyocyte stained with DAPI (pink) and antibody against Myomesin (green, M‐band). (A and B) Courtesy of E. Ehler. (C) Image of adult zebrafish cardiomyocytes after 7 days in culture and 3 days of BrdU treatment; the cells were stained with DAPI and antibodies against BrdU (green) and Myosin (red); the presence of BrdU‐positive nuclei (green arrows) indicates proliferative activity of the cultured cardiomyocytes; non‐proliferating cardiomoycytes are indicated by white arrows. (D) Schematic representation of different levels of cardiomyocyte specialization in the adult heart across species: differentiated myocytes of zebrafish and newts display a less complex cytoarchitecture than their mammalian counterparts; the specialization level is adapted to organismic demands
In mammals, it has been suggested that the enlarged size, complex membranous architecture and dense intracellular sarcomere network might impede cell division 118. This assumption has been refined by studies of cultured cardiomyocytes, showing that the constraints for cell division are represented by the loss of centrosome integrity and defective contractile ring formation 119, 120. Nevertheless, measurements of nucleotide analogue incorporation and analysis of 14C levels have provided evidence of DNA synthesis activity and low cardiomyocyte turnover in the adult mammalian heart 121, 122, 123, 124. Moreover, several laboratories have demonstrated a proliferative response of adult cardiac cells after various experimental manipulations, such as transfection with specific micro‐RNAs (miRNAs) 125, 126, 127, modulation of cyclin expression 128, 129 or administration of specific extracellular factors 130, 131, 132, 133, 134. In general, these findings suggest an inherent potential of differentiated mammalian cardiomyocytes to access the proliferative machinery under appropriate circumstances. Boosting of this potential represents an interesting perspective for regenerative medicine.
Although adult cardiomyocytes in newts and zebrafish are capable of proliferation, cardiac injury has been shown to trigger several features of cellular dedifferentiation in these model organisms. Specifically, transmission electron microscopy imaging in the zebrafish has revealed that proliferating cardiomyocytes show disassembled sarcomeric structures in the vicinity of the injury 97. Moreover, certain developmental genes, such as the cardiac transcription factors gata4 and hand2, or embryo‐specific myosin heavy chain, are up‐regulated in the regenerating myocardium in zebrafish 97, 98, 99, 135, 136, 137, 138. Ventricular injury in newts has been associated with the down‐regulation of cardiac muscle genes 8, 93, 94, 95, 96. In these species, dedifferentiation has been suggested to facilitate mitotic and morphogenetic activity during reconstitution of the lost part of the myocardium 96, 97, 99, 136. Nevertheless, a clear interdependency between these two processes has not yet been established. Dedifferentiation of adult mammalian cardiomyocytes has also been observed during cardiac remodelling under diverse circumstances, including heart ischaemia 139, 140, 141, 142. In addition to sarcomere disorganization, this conversion is also characterized by a metabolic switch to carbohydrates to preserve cardiac function under pathophysiological stress. However, such modulation of structural and metabolic activities is not associated with enhanced cardiomyocyte proliferation 139, 140, 143, 144, 145, 146. Further comparative analysis between different model organisms would shed light on the mechanisms governing plasticity of cardiomyocytes in response to heart injury.
In summary, several in vitro and in vivo studies have shown that mammalian, zebrafish and newt cardiomyocyte cell division occurs either with or without any evident sign of dedifferentiation 96, 99, 111. In zebrafish, the local generation of less‐differentiated cardiomyocytes in the proliferative zone of the injury border is accompanied by stimulation of mitotic divisions in the remote part of the ventricle, where dedifferentiation was not detected (Figure5). The organ‐wide proliferative response resembles a compensatory mode of regeneration, such as in mammalian liver 147. Further studies are needed to determine the mechanisms and significance of enhanced cardiomyocyte proliferation in the injury‐remote part of the ventricle. Moreover, the level of independence between dedifferentiation and proliferation has to be thoroughly assessed under various conditions, such as cardiac regeneration, normal ontogenetic growth or homeostatic turnover.

Zebrafish heart regeneration involves proliferation of mature and dedifferentiated cardiomyocytes. (A) Model showing two possible modes of cardiomyocyte proliferation during cardiac regeneration in zebrafish. At the vicinity of the injured zone, cell‐cycling activity is observed in dedifferentiated cardiomyocytes, which re‐express embryonic markers; this response predominantly occurs during the initial stages (1–2 weeks) of regeneration 99. Cardiomyocytes can also enter mitosis without a visible modification of their structural differentiation status. This process occurs in an organ‐wide pattern during regeneration but also in intact hearts during ontogenetic growth. (B, C) Confocal images of heart sections from transgenic fish cmlc2:EGFP (blue) at 7 days post‐cryoinjury (dpci). (B) A population of cardiomyocytes (blue) at the injury border expresses a proliferation marker, Minichromosome maintenance complex component 5 (MCM5; green) and reactivates the expression of an embryo‐specific isoform of Cardiac myosin heavy chain (embCMHC; red), which indicates cellular dedifferentiation. (C) The immunostaining for phospho‐(Ser10)‐Histone H3 (PH3; green) reveals the presence of mitotic cardiomyocytes at the injury‐remote position; in this case, cell cycle re‐entry occurs without a re‐expression of embCMHC 99, 186
Heart: cellular interactions in cardiac regeneration
Cardiac regeneration in lower vertebrates involves essential molecular interplays between cardiomyocytes and other cardiac components, such as epicardium, endocardium and nerves 148, 149, 150. Among these interactions, FGF, IGF and retinoic acid (RA) signalling represent key communicative elements between epi/endocardial cells and cardiomyocytes 138, 151, 152, 153. Shortly after injury, the epi/endocardium start to re‐express embryonic markers, including the transcription factors tbx18, wt1 or the RA‐synthetizing enzyme retinaldehyde dehydrogenase 2 (raldh2), and release RA, which represents an important mitogen for cardiomyocytes during development and regeneration 138, 152, 154. In addition, epicardial cells have been shown to enhance the migration and integration of cardiomyocytes in the injured area 155, 156 and to promote neovascularization in this zone through the production of epicardial‐derived cells (EPDCs), which migrate in the regenerating myocardium and form perivascular cells 138, 157, 158. Thus, epicardial cells play pleiotropic roles during cardiac regeneration in zebrafish. Interestingly, a developmental reactivation of the adult epicardium has also been observed after myocardial infarction in adult mammals 148, 159, 160. Therefore, the potential of epicardial cells might be further considered in the treatment of myocardial infarction in humans.
In opposition to the concept of 'scarless regeneration' in vertebrate appendages, accumulation of fibrotic tissue in the post‐infarcted heart is thought to play a beneficial structural role by providing robustness to the force‐generating contractile organ 161, 162. In mammals, dense layers of collagen are deposited to replace a damaged part of the ventricular wall, which is challenged to withstand the high internal blood pressure. In comparison to humans and rodents, fish and amphibian ventricles are confronted by a lesser workload 53, 163, 164, which may permit heart regeneration without the involvement of compact scarring. Instead, a transient collagenous trabecular network is sufficient and necessary to support the function of the injured ventricle and to provide a guidance scaffold for invading cardiomyocytes in zebrafish (Figure6A–D) and newts 52. Our laboratory has demonstrated that the suppression of collagen deposition by inhibition of TGFβ signalling coincided with regenerative failure and geometrical deformation of infarct shape 165. Thus, collagen‐deficient infarcts can lead to detrimental remodelling in both mammals and fish. A balance between transient fibrosis and progressive replenishing of cardiomyocytes has to be achieved to synchronize the reparative and regenerative processes according to physiological conditions.

Transient connective tissue in the post‐infarcted in zebrafish; transverse sections of the zebrafish ventricle at 14 days post‐cryoinjury (dpci); the post‐infarcted area is encircled with a dashed line. (A, B) Haematoxylin (dark purple, nuclei) and eosin (red, proteins) (H&E) staining; the infarct contains acellular remnants of fibrin that were deposited shortly after cryoinjury (pink) and granulation tissue. (C, D) Aniline blue, acid Fuchsin and Orange G (AFOG) staining reveals collagen in blue, fibrin in red and cytoplasm in orange; the infarct tissue is supported by fibrin (red) and loose network of collagen fibres that serves as a provisional structural matrix and a scaffold for migrating cardiomyocytes (orange) 44. (E–J) Confocal images of heart sections immunostained with antibody against Tropomyosin (TPM; red) to highlight the intact myocardium and a marker of connective tissue. (E, F) α‐Smooth muscle actin (α‐SMA)‐positive myofibroblasts (green) accumulate along the outer border of the infarct. (G, H) Fibronectin (green) forms a reticular pattern in the infarct zone. (I, J) Tenascin C (green) is predominantly detected at the interface of connective tissue and regenerating myocardium. Scale bars=
100
µm
Transdifferentiation of fibroblasts into myofibroblasts, which express α‐Smooth muscle actin (α‐SMA), is associated with fibrosis in mammalian heart 166, 167, 168, 169, 170. In injured hearts of zebrafish, α‐SMA‐positive myofibroblasts accumulate along a peripheral rim of the post‐infarct and only a few of these cells penetrate the central portion of the damaged zone 165 (Figure6E, F). This distribution suggests a role of the contractile fibroblasts in providing mechanical stability to the outer border of the damaged ventricular wall in zebrafish. Other fibroblast populations infiltrate the middle portion of the post‐infarct, where they produce the ECM that is known not only to have structural functions but also to regulate many cellular processes, including cell migration, proliferation and differentiation, via, for instance, integrin signalling 171, 172, 173, 174. Cell–substrate communication has been attributed to various adhesive and matricellular proteins, including fibronectin and tenascin C, respectively 166, 175, 176, 177. During heart regeneration in zebrafish, the entire post‐infarct area displays abundant expression of fibronectin, while tenascin C remains predominantly enriched at the interface between the regenerating myocardium and connective tissue 155, 165 (Figure
6G–J). Similarly, fibronectin, tenascin‐C and hyaluronic acid form a pro‐regenerative matrix in the newt heart 178. During regeneration, this connective matrix progressively resolves by mechanisms that still remain poorly understood. Defining the intrinsic properties of fibroblasts and the external factors that determine the composition and dynamics of the ECM in the myocardial post‐infarcts would be highly relevant for regenerative medicine.
Outlook
The appendage and heart cells of some anamniotic vertebrates possess an intrinsic ability to respond to injury by proliferation and reactivation of morphogenetic programmes to replace the missing structures. This remarkable regenerative capability correlates with the relatively low cytospecialization of the adult organs in teleost fish and urodele amphibians in comparison to mammals. Histological analyses indicate that the adult differentiated cells of the non‐mammalian and mammalian species cannot be directly placed on the same scale. Although mature appendage and cardiac cells in fish and urodeles maintain an intrinsic proliferative potential, cellular dedifferentiation is often observed at the site of injury of the regenerating organs. This suggests a general significance of the separation of tissues from one another and a function of tissue isolation for regaining of a certain degree of cellular plasticity. In this context, dedifferentiation does not need to imply a complete reversion to an embryonic state, but a temporary reduction of the cellular complexity, which may facilitate proliferation and morphogenesis. Mimicking this strategy in injured mammalian organs would demand a molecular toolkit to impose a transient and partial reversion of the local tissue complexity without reprogramming into the pluripotent state. The recent advances of cell fate conversion suggest that the manipulation of cellular specialization seems to be an achievable goal 179, 180, 181. The challenging part of this task concerns the understanding of organismic factors that impact tissue regeneration at molecular and biomechanical levels. The combination of findings across different animals will help to recognize the permissive and restrictive factors to establish a regeneration‐competent environment. These advances will move the field of regenerative biology closer to clinical implementation.
Author contributions
All authors contributed equally to this review and revised the final manuscript.
Acknowledgements
We thank L Mène‐Saffrané, A Buchala, T Carney, D König and C Pfefferli for critical reading of the review, V Duruz for preparing a figure, E Ehler for images of mammalian cardiomyocytes and M Celio for providing histological material. This work was supported by the Schweizerische Stiftung für die Erforschung der Muskelkrankheiten and the Swiss National Science Foundation (Grant Nos 310030_159995 and CRSII3_147675).
Notes
No conflicts of interest were declared.
References
Full text links
Read article at publisher's site: https://doi.org/10.1002/path.4644
Read article for free, from open access legal sources, via Unpaywall:
https://onlinelibrary.wiley.com/doi/pdfdirect/10.1002/path.4644
Citations & impact
Impact metrics
Article citations
Syndecan-4 is required for early-stage repair responses during zebrafish heart regeneration.
Mol Biol Rep, 51(1):604, 03 May 2024
Cited by: 0 articles | PMID: 38700644 | PMCID: PMC11068835
Lineage Tracing of Bone Cells in the Regenerating Fin and During Repair of Bone Lesions.
Methods Mol Biol, 2707:99-110, 01 Jan 2024
Cited by: 0 articles | PMID: 37668907
The salamander blastema within the broader context of metazoan regeneration.
Front Cell Dev Biol, 11:1206157, 11 Aug 2023
Cited by: 3 articles | PMID: 37635872 | PMCID: PMC10450636
Review Free full text in Europe PMC
Unlocking cardiomyocyte renewal potential for myocardial regeneration therapy.
J Mol Cell Cardiol, 177:9-20, 17 Feb 2023
Cited by: 4 articles | PMID: 36801396 | PMCID: PMC10699255
Review Free full text in Europe PMC
Molecular characterization of the immediate wound response of the solitary ascidian Polycarpa mytiligera.
Dev Dyn, 251(12):1968-1981, 19 Sep 2022
Cited by: 3 articles | PMID: 36001356 | PMCID: PMC10087333
Go to all (41) article citations
Similar Articles
To arrive at the top five similar articles we use a word-weighted algorithm to compare words from the Title and Abstract of each citation.
A dual epimorphic and compensatory mode of heart regeneration in zebrafish.
Dev Biol, 399(1):27-40, 31 Dec 2014
Cited by: 63 articles | PMID: 25557620
Specific NuRD components are required for fin regeneration in zebrafish.
BMC Biol, 12:30, 29 Apr 2014
Cited by: 21 articles | PMID: 24779377 | PMCID: PMC4038851
Uncovering the regeneration strategies of zebrafish organs: a comprehensive systems biology study on heart, cerebellum, fin, and retina regeneration.
BMC Syst Biol, 12(suppl 2):29, 19 Mar 2018
Cited by: 7 articles | PMID: 29560825 | PMCID: PMC5861487
The zebrafish as a model for complex tissue regeneration.
Trends Genet, 29(11):611-620, 06 Aug 2013
Cited by: 281 articles | PMID: 23927865 | PMCID: PMC3812420
Review Free full text in Europe PMC
Funding
Funders who supported this work.
Swiss National Science Foundation (4)
Grant ID: CRSII3_147675
Grant ID: 310030_159995
Mechanisms of organ regeneration in zebrafish
Anna Jazwinska, University of Fribourg
Grant ID: 159995
Grant ID: 310030