Abstract
Free full text

From the Cover
Firing properties of dopamine neurons in freely moving dopamine-deficient mice: Effects of dopamine receptor activation and anesthesia
Abstract
To examine the regulation of midbrain dopamine neurons, recordings were obtained from single neurons of freely moving, genetically engineered dopamine-deficient (DD) mice. DD mice were tested without dopamine signaling (basal state) and with endogenous dopamine signaling (after L-dopa administration). In the basal state, when dopamine concentration in DD mice is <1% of that in control animals, the firing properties of midbrain dopamine neurons were remarkably similar among genotypes. However, L-dopa treatment, which restores dopamine and feeding and locomotor behavior in DD mice, profoundly inhibited the firing rate and bursting of dopamine neurons in DD mice. In addition, dopamine neurons in DD mice were hypersensitive to the dopamine receptor agonists quinpirole and SKF 81297. Anesthesia markedly reduced the firing rate of dopamine neurons in DD mice but did not significantly decrease the firing rate in control dopamine neurons. These data suggest that restoration of endogenous dopamine signaling activates hypersensitive long-loop feedback pathways that serve to limit dopamine release and underscore the importance of recording from awake animals.
Dopamine neurons fire in pacemaker, irregular, and bursting modes (1-6). Different modes of dopamine neuron activity are thought to lead to different forms of neuronal plasticity. For example, in molecular models of learning, long-term depression at corticostriatal synapses is induced by the release of low concentrations of dopamine (i.e., the pacemaker or irregular mode), whereas long-term potentiation is induced by the release of high concentrations of dopamine (i.e., the bursting mode) (7-13). These dopamine-dependent forms of synaptic plasticity provide a basis for context-dependent changes that may underlie reward-related learning. Behavioral evidence also supports a role for dopamine in reward-related learning (14). Specifically, burst firing of dopamine neurons (6, 15, 16) and transient increases in striatal dopamine concentration (17, 18) have been shown to correlate with behavioral adaptations during reward-related learning in primates and rodents.
The DD mouse model provides an excellent opportunity to test whether behaviorally relevant burst firing of dopamine neurons is necessary for goal-directed, reward-related behaviors. In contrast to lesion models, dopamine neurons in DD mice are intact, and the ability to restore endogenous dopamine signaling is under experimenter control. DD mice were generated by the selective inactivation of the tyrosine hydroxylase gene in dopamine neurons (19). Endogenous dopamine signaling is restored in DD mice by administration of L-dopa, which is presumably taken up by dopamine neurons, converted to dopamine, packaged into vesicles, and released in a behaviorally relevant manner. After L-dopa administration, DD mice become hyperactive and hyperphagic, consuming all of their daily food within 6-9 h, after which they again become dopamine-depleted and thus hypoactive and hypophagic (19, 20).
One objective of this study was to characterize the basal firing properties of dopamine neurons and their responses to dopamine receptor D1 (D1R) and dopamine receptor D2 (D2R) agonists. These characterization experiments were conducted to (i) permit comparison of recordings from freely moving mice with previously reported recordings from intact and dopamine-depleted rats (2-4, 21-27), (ii) compare the effects of exogenous dopamine receptor activation (agonist studies) to the effects of endogenous dopamine release (L-dopa studies), and (iii) provide a foundation from which to design future studies in DD mice that explore the role of dopamine in reward-related behaviors.
In contrast to L-dopa, dopamine receptor agonists do not elicit sufficient feeding by DD mice for their survival, whereas both L-dopa and dopamine agonists stimulate locomotion (19, 20, 28). One interpretation of this phenomenon is that burst firing and transient activation of dopamine receptors, as achieved after L-dopa administration (but not with agonist treatment), is necessary for shaping and maintaining certain goal-directed behaviors, such as eating. Therefore, we investigated changes in dopamine neuron activity that accompany L-dopa-mediated restoration of goal-directed behaviors. We considered the following scenarios, while recognizing that many factors could affect the firing properties of dopamine neurons of DD mice. First, L-dopa administration to DD mice might restore burst firing along with behavioral activation and feeding. This possibility is based on our previous finding that dopamine neurons of anesthetized DD mice did not fire in bursts, unlike controls, and that L-dopa partially restored bursting activity (29). Second, the bursting activity and firing rate of dopamine neurons from DD mice might exceed that of controls, and L-dopa treatment might slightly dampen neural activity to match that of controls. This scenario assumes that the lack of burst firing reported previously was an artifact of anesthesia (30) and that dopamine dampens activity via D2 autoreceptors, as described by others (27, 31-35). Third, the bursting activity of dopamine neurons might be normal in the absence of dopamine but greatly suppressed by treatment with L-dopa as a consequence of the hypersensitive striatal projection neurons that inhibit dopamine neuron activity through long-loop, feedback pathways. This possibility is based on reports describing the effects of dopamine receptor agonists in dopamine-depleted rats (25, 26). The results support the third possibility, which indicates that life-sustaining behavioral activation obtained by restoration of dopamine signaling with L-dopa is accompanied by substantial inhibition of midbrain dopamine neuron activity.
Materials and Methods
Extracellular Recordings in Freely Moving Mice. Surgical procedures were performed in accordance with guidelines established by the University of Washington Animal Care Committee. Tungsten electrodes were manufactured as stereotrodes as described (36). Moveable microdrives composed of four stereotrodes were implanted by using the following coordinates (in mm): anterio-posterior = 3.2; mediolateral = 0.5 from bregma; and dorsoventral = 3.5 from dura. One week later, individual mice were placed in a recording arena and attached to the recording cable. Electrodes were advanced in 22-μm increments until putative dopamine neurons were encountered (defined below). The neural signals were passed through field-effect transistors, filtered between 600 and 6,000 Hz, and amplified (1,000- to 10,000-fold). Records containing spikes exceeding a user-defined threshold were amplified, filtered, and saved for offline analysis with the Neuralynx Data Acquisition System (Tucson, AZ).
Identification of Dopamine Neurons. A neuron was classified as dopaminergic if the following criteria were met: (i) postmortem histological confirmation that an electrode was located in the substantia nigra pars compacta/ventral tegmental area; (ii) an average firing rate of <15 Hz; and (iii) pharmacological identification: the firing rate had to be inhibited by >80% after D2R activation and partially reversed by D2R antagonism. Therefore, in most experiments, quinpirole (QUIN, a D2R agonist) and eticlopride (ETIC, a D2R antagonist), the “confirmation drugs,” were administered after the “experimental drugs.” To avoid false-positive statistical results, the effects of the confirmation drugs were analyzed separately from the effects of the experimental drugs. Neurons that did not meet these criteria were considered nondopaminergic and were not included in the analysis.
Data Analysis. Waveforms from putative dopamine neurons were sorted and analyzed offline by using mclust software, by A. David Redish, written for matlab (The MathWorks, Natick, MA). The sorting process was facilitated by the use of stereotrodes, which allows for discrimination of many individual neurons recorded simultaneously from multiunit recordings (see Fig. 1). After the records from individual neurons were isolated, the basal firing properties and responses by individual neurons to drugs were analyzed by using software written for matlab, burst analysis by Chris Higginson. Values were generated for the following parameters: the average firing rate, the average interspike interval (ISI), the average percentage of spikes fired in bursts (%SFB), the average number of spikes fired within a burst, and the average within-burst firing frequency. For each parameter, a mean value was generated per animal per 4-min block. Bursts were defined by using the previously established criteria of ≤80 msec ISI to signal the onset of a burst and an ISI of >160 msec to signal the end of a burst (4). To calculate the %SFB, the number of spikes fired during bursts was divided by the total number of spikes per 4-min block. Autocorrelograms and ISI histograms were generated to aid in identification of firing patterns (1, 5). One-way and repeated-measures ANOVA followed by Tukey's post hoc analysis were used to analyze the data.
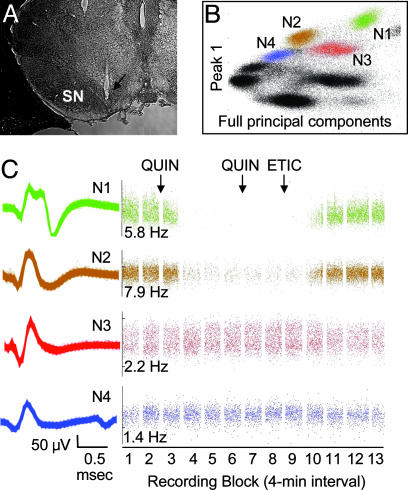
Identification of dopamine neurons in freely moving mice. (A) Coronal section showing the location of the recording electrode (arrow). (B) Clusters that represent four distinct neurons (N1-N4) recorded from a single stereotrode in a DD mouse. Each dot in a cluster represents one action potential. The remaining clusters (black) are shown to illustrate how the data appear before clusters are assigned. (B) Cluster plot axes denote waveform characteristics used to isolate clusters. (C) Representative waveform traces (Left) from clusters shown in B and drug-induced changes in firing rate (Right) in response to QUIN (50 μg/kg) and ETIC (0.5 mg/kg). In C and in all subsequent figures, the recording sessions were divided into 4-min blocks separated by 1-min intervals during which drugs were administered (arrows). Baseline firing rates (Hz) are shown on the raster displays.
Drugs. All drugs were obtained from Sigma or Research Biochemicals (Natick, MA) and were administered i.p. at a volume of 10 μl/g (except L-dopa, see below). Apomorphine was administered at 0.1 or 0.5 mg/kg in 0.9% saline; QUIN was administered at various doses (25-500 μg/kg) in 0.9% saline; ETIC was diluted in sterile water and administered at 0.1 and 0.5 mg/kg; SKF 81297 (SKF; 6-chloro-7,8-dihydroxy-1-phenyl-2,3,4,5-tetrahydro-1H-3-benzazepine) was diluted in 0.9% saline and administered at 1.25 mg/kg; SCH 23390 (SCH; 7-chloro-8-hydroxy-3-methyl-1-phenyl-2,3,4,5-tetrahydro-1H-3-benzazepine) was diluted in sterile water and administered at 0.1 and 0.5 mg/kg. Ketamine/xylazine/acepromazine (KXA) were diluted in PBS to final concentrations of 10%, 10%, and 0.06%, respectively. L-dopa was dissolved in 0.25% ascorbate in PBS and administered at 50 mg/kg (33 μl/g).
Histology. At the end of the experiment, the mice were deeply anesthetized and perfused transcardially with fixative. The brains were quickly removed, frozen, sectioned at 30 μm, and stained with cresyl violet.
Results
Identification of Dopamine Neurons. Histological examinations indicated that most electrodes were located in the substantia nigra pars compacta (Fig. 1A) with a few recording sites in the ventral tegmental area. In two brains, it was difficult to see the exact location of the recording electrode, which extended ≈2 mm beyond the tip of the cannula. For these cases, we relied on the pharmacological criteria to identify dopamine neurons. Clusters that represent four distinct neurons (N1-N4) recorded from a single stereotrode in a DD mouse are shown (Fig. 1B), along with representative waveform traces (Fig. 1C Left) and raster displays depicting drug-induced changes in firing rate (Fig. 1C Right). Two neurons (N1 and N2) were classified as dopaminergic based on firing rate (<15 Hz) and their responses to D2R activation and subsequent blockade. In contrast, neurons N3 and N4 were not inhibited by QUIN and thus were classified as nondopaminergic.
Basal Firing Properties of Dopamine Neurons. Baseline data from five control mice (105 neurons) and from six DD mice (71 neurons) were collected during 12 recording sessions. A total of 68 neurons (36 DD and 32 control mice) were identified as putative dopamine neurons. Dopamine neurons from DD and control mice had waveforms that were typically 1-2 msec in duration (Fig. 1C). As shown in Table 1, the basal firing properties of dopamine neurons from DD and control mice were similar; no differences were found in firing rate, ISI, number of spikes fired per burst, %SFB, or within-burst firing frequency. The scatter plot (Fig. 2A) shows the %SFB as a function of firing rate for each dopamine neuron. There were significant positive correlations between firing rate and the %SFB for dopamine neurons in both genotypes (DD, r = 0.81, P < 0.001; control, r = 0.70, P < 0.001, Pearson's correlation). The three firing modes (bursting, irregular, and pacemaker) that are typically encountered during recordings of dopamine neurons in rodents were present in both genotypes (5, 6). Representative ISI histograms (Left) and autocorrelograms (Right) of three dopamine neurons from DD mice are displayed in Fig. 2B (see legend for details).
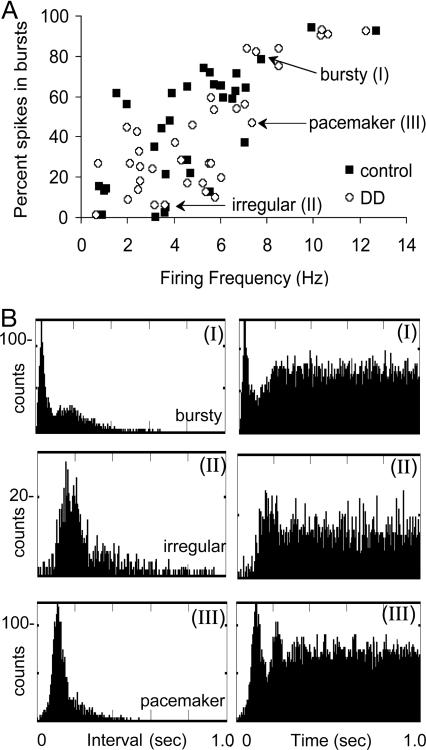
Basal firing properties of dopamine neurons are similar in DD and control mice. (A) Scatterplot showing the %SFB as a function of firing rate of individual neurons from DD (36 neurons) and control (32 neurons) mice from a 4-min baseline recording block. (B) ISI histograms (Left) and autocorrelograms (Right) describing the firing patterns typical of dopamine neurons. (I) This dopamine neuron fired predominantly in the bursting mode; the “burstiness” of this neuron is described by the bimodal ISI histogram (highest peak represents intervals between spikes that were fired within the burst mode, and the lower, more skewed peak represents intervals between spikes that were fired in the single-spike mode) and the initial peak in the autocorrelogram with a decay to a steady-state level. (II) A neuron that typically fired in the irregular mode; this neuron fired in a single-spike pattern with few bursts, indicated by the relatively long, skewed ISI histogram and the flat autocorrelogram, with a paucity of counts within the first 100 msec. (III) This dopamine neuron fired in a pacemaker mode, as indicated by the narrow ISI histogram and the distinct peaks in the autocorrelogram. Data are binned in 5-sec intervals.
Table 1.
Mice | No. of mice | No. of dopamine neurons | FF, Hz | %SFB | No. of spikes per burst | WBF, Hz | ISI, msec |
---|---|---|---|---|---|---|---|
Control mean | 5 | 32 of 105 | 4.6 ± 0.4 | 48.9 ± 5.7 | 4.0 ± 0.8 | 40.2 ± 2.6 | 388.8 ± 41.5 |
Control range | 0.8−12.7 | 0.3−93.8 | 2.0−19.3 | 25.1−100.1 | 49.3−1622 | ||
DD mean | 6 | 36 of 71 | 5.3 ± 0.4 | 41.6 ± 3.7 | 3.7 ± 0.2 | 39.4 ± 2.8 | 278.4 ± 39.2 |
DD range | 0.6−12.3 | 1.3−92.7 | 2.0−10.24 | 21.4−126.6 | 81.6−1515 |
Data are expressed as mean ± SEM and range from 4-min baseline recording sessions. FF, firing frequency; WBF, within-burst frequency; m, mean; r, range
Response to l-Dopa Administration. L-dopa profoundly inhibited the firing rate of the majority of dopamine neurons recorded from DD mice but had little effect in control mice. Representative examples of this difference are depicted in Fig. 3A. The effects of L-dopa on dopamine neurons from all mice are summarized in Fig. 3B. In this experiment, a total of 35 dopamine neurons were recorded from four DD mice. Visual inspection of the raster displays generated for individual neurons revealed that 66% (23 of 35) of dopamine neurons in this sample were inhibited by L-dopa, whereas 34% (12 of 35) were nonresponsive (but were inhibited >80% by D2R activation). We concluded that these were distinct populations of dopamine neurons and did not include the nonresponsive neurons (12 of 35) in the analysis.
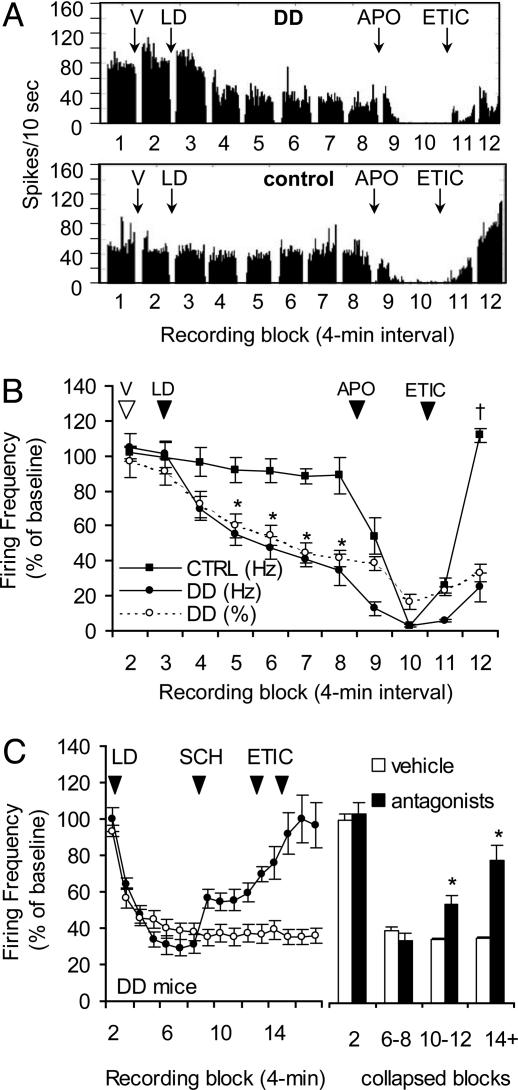
Dopamine neurons in DD mice are inhibited by l-dopa. (A) Histograms showing that l-dopa (50 mg/kg) decreased the firing rate of a dopamine neuron from a DD mouse (Upper) but not a control mouse (Lower). Data are binned in 10-sec intervals. (B) Summary data showing that l-dopa (LD) decreased the firing rate and %SFB of dopamine neurons in DD mice (23 of 35 neurons, four mice) during blocks 5-8, whereas dopamine neurons from control mice (12 neurons, three mice) were not significantly inhibited. Each data point represents the mean firing rate for each 4-min block (for all figures). APO, apomorphine; V, vehicle. *, P < 0.001 compared with DD VEH; †, P < 0.001 compared with DD block 12. (C) Summary data showing that D1R and D2R antagonism attenuates the l-dopa effect (Left). The mean drug effect (Right) was calculated by averaging the mean firing rates during the following blocks: LD, blocks 6-8; ETIC, blocks 10-12; and SCH, blocks 14-17.
Repeated-measures ANOVA was conducted from blocks 2-8 to evaluate the time course of the response to L-dopa. There was a main effect for the time × genotype interaction [F (6, 30) = 16.23, P < 0.001]. Inspection of the curves revealed that dopamine neurons (23 of 35) in DD mice had a steep decrease (≈65% inhibition) in response over time, compared with dopamine neurons (n = 12) from three control mice. The firing rates of dopamine neurons in DD mice were significantly inhibited during blocks 5-8 [P < 0.001 compared with DD vehicle (VEH)], whereas dopamine neurons in control mice were unaffected by L-dopa. The %SFB (Fig. 3B, ○) decreased in parallel to the firing rate after L-dopa. Different doses of the confirmation drugs were administered to DD and control mice in this experiment because of the behavioral hypersensitivity to D1R agonists manifested by DD mice (blocks 9-12).
To elucidate the contribution of dopamine receptor subtypes in the L-dopa-induced inhibition of firing rate, D1R- and D2R-specific antagonists were administered to DD mice after L-dopa treatment (Fig. 3C). A total of 22 dopamine neurons from four DD mice decreased their firing rate in response to L-dopa (blocks 3-8). The L-dopa-induced inhibition of dopamine neuron activity was partially reversed by the D1R antagonist SCH and further reversed by ETIC (Fig. 3C, •). To quantify the effects of the antagonists, data from individual blocks after an injection (L-dopa, SCH, or ETIC) were collapsed to give one value for each drug effect (Fig. 3C Right). Repeated-measures ANOVA involved the VEH, L-dopa, SCH, and ETIC treatments in DD mice and revealed a main effect of treatment [F (16, 16) = 15.86, P < 0.001]. The L-dopa-induced inhibition was significantly attenuated by SCH (blocks 10-12, P < 0.05 compared with L-dopa) and by ETIC (blocks 14-17, P < 0.001 compared with L-dopa; P < 0.01 compared with SCH). L-dopa was administered to one DD mouse without antagonists to monitor the stability of the L-dopa-induced inhibition. During this recording, 73% (11 of 15) of dopamine neurons were inhibited by L-dopa for the entire session (Fig. 3C, ○), indicating that the L-dopa effect was long-lasting and stable and that the changes observed after antagonist treatment were not due to spontaneous recovery of firing rate. These data suggest that both D1R and D2R activation contribute to the L-dopa-induced inhibition of dopamine neurons in DD mice.
Response to D2R Activation. Previous reports have shown that D2 autoreceptors are hypersensitive to dopamine receptor agonists after dopamine-depleting lesions of rats (24). In addition, DD mice are hyperactive in response to D2R agonists (19, 28). However we found no difference in D2 autoreceptor sensitivity in slices from control and DD mice (29). We chose to revisit the issue of D2R hypersensitivity by measuring the activity of dopamine neurons in freely moving mice after D2R activation. During each session, one of four doses of QUIN (25, 50, 75, or 100 μg/kg; see Fig. 4A for injection schedule) was administered (followed 20 min later by the confirmation drugs). Over the course of the experiment, each mouse was tested with each experimental dose of QUIN in random order; each dose was separated by ≥2 days. Fig. 4A shows that a moderate dose of QUIN (50 μg/kg) inhibited the firing rate of a dopamine neuron from a DD mouse (Upper) but had little effect on a dopamine neuron from a control mouse (Lower). The %SFB decreased in parallel with the firing rate (data not shown). The QUIN dose-response experiment is summarized in Fig. 4B. Repeated-measures ANOVA was used to compare the responses of dopamine neurons (15-27 neurons per data point) to VEH and to each dose of QUIN (data from block 5 were used in this analysis because it represented stable activity after QUIN) and found a dose × genotype interaction [F (4, 16) = 13.30, P < 0.001]. Post hoc analysis revealed that dopamine neurons in DD mice were inhibited at each dose of QUIN, whereas dopamine neurons in control mice were only inhibited by the two higher doses. A second analysis involved the confirmation drugs: the responses to VEH (block 2), the second dose of QUIN (block 8), and ETIC (block 12) were compared among genotypes. Repeated-measures ANOVA found a significant genotype × treatment interaction [F (2, 8) = 99.87, P < 0.001]. The two genotypes responded similarly to VEH and to QUIN, but ETIC affected DD and control dopamine neurons differently. In control mice, ETIC caused the firing rate to significantly overshoot baseline value (P < 0.001 compared with control VEH), whereas in DD mice, the firing rate was only restored to baseline (P > 0.05 compared with DD VEH). Fig. 4C shows that the half-maximal inhibition (ED50) of dopamine neuron activity in DD mice was achieved with the 25 μg/kg dose, compared with an ED50 of 75 μg/kg for controls. These QUIN experiments reveal that dopamine neurons in DD mice are ≈3 times more sensitive than controls to D2R activation. In addition, the ETIC results suggest that dopamine receptors are tonically occupied by endogenous dopamine in control mice but not in DD mice.
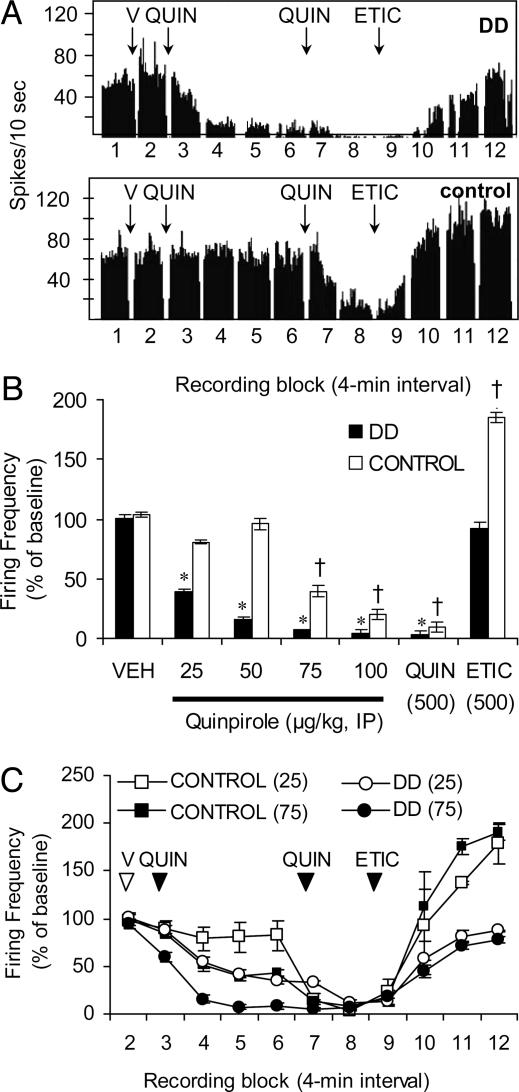
Dopamine neurons in DD mice are hypersensitive D2R activation. (A) Histograms showing that QUIN (50 μg/kg) decreased the firing rate of a dopamine neuron from a DD mouse (Upper), but not a control mouse (Lower). QUIN (0.5 mg/kg, third arrow) and ETIC (0.5 mg/kg, fourth arrow) were administered at the end of each recording session to identify dopamine neurons. (B) Summary data showing the dose-dependent decreases in firing rate in response to four experimental doses of QUIN. Each data point represents the mean firing rate of 14-27 dopamine neurons from four DD and two control mice 15 min after administration of QUIN. *, P < 0.001 compared with DD VEH; †, P < 0.001 compared with control VEH. (C) Summary data showing the time course of QUIN-induced inhibition of firing rate of dopamine neurons in DD mice (21-24 neurons) and controls (13-14 neurons).
Response to D1R Activation. SKF, a D1R agonist, induces robust c-fos expression in the striatum and causes hyperlocomotion in DD mice suggestive of hypersensitive D1R-containing striatal projection neurons in DD mice (28). To determine the effects of D1R activation on the firing properties of dopamine neurons, SKF (1.25 mg/kg) was administered to DD and control mice. The specificity of the response was measured by administering D2R and D1R antagonists after SKF. Repeated-measures ANOVA was conducted on the responses to VEH, SKF, ETIC, and SCH for both genotypes and revealed a main effect for the genotype × treatment interaction [F (3, 12) = 27.27, P < 0.001]. Fig. 5 shows that, at this low dose, SKF significantly inhibited the firing rate of dopamine neurons in DD mice to 30% of baseline (n = 3 mice; 13 dopamine neurons) but was without effect in control mice (n = 3 mice; 15 dopamine neurons). This D1R-mediated inhibition of dopamine neurons in DD mice was not significantly attenuated by ETIC (Fig. 5, SKF + ETIC; P < 0.05 compared with DD VEH), whereas D1R antagonism significantly attenuated the SKF-induced inhibition (SKF + ETIC + SCH; P > 0.05 compared with DD VEH). In contrast, in controls, ETIC significantly increased the firing rate of dopamine neurons above baseline (2.5-fold) presumably because of antagonism of endogenous dopamine at D2Rs (P < 0.01). The %SFB was similarly reduced with SKF treatment (data not shown).
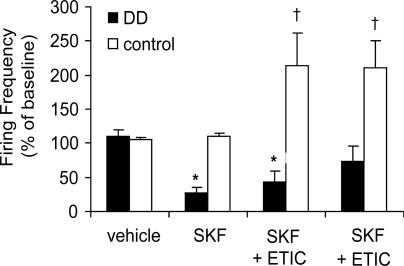
D1R activation inhibits dopamine neurons in DD mice. Summary data from three DD (13 dopamine neurons) and three control (15 dopamine neurons) mice show that SKF (1.25 mg/kg) inhibited the firing rate of dopamine neurons in DD mice but not control mice. Each mouse was injected with VEH and then SKF. ETIC (0.1 mg/kg) was administered 20 min later, followed by SCH (0.5 mg/kg) 15 min later. Note that a D1R but not a D2R antagonist attenuated the effects of SKF. *, P < 0.001 compared with DD VEH; †, P < 0.001 compared with control VEH.
Effects of Anesthesia on Dopamine Neurons. Previously, we reported that dopamine neurons in DD mice do not fire in bursts; however, the mice were deeply anesthetized. Because we found different results in freely moving DD mice (≈42% of spikes were fired in bursts; Table 1), we were interested in comparing the firing rates and %SFB in both states (awake and anesthetized) in the same animal. Dopamine neurons in DD (n = 4 mice; 14 dopamine neurons) and control (n = 2 mice; 8 dopamine neurons) mice were recorded for a baseline block and a VEH block, and then the mice were injected with KXA. Mice were monitored until they were fully anesthetized and then injected with either L-dopa or VEH. Statistical analysis was conducted on data from DD mice from blocks 2-11 to evaluate the effect of KXA (Fig. 6). Main effects of genotype [F (1, 4) = 12.68, P < 0.05] and time [F (9, 36) = 8.18, P < 0.001] were observed, and post hoc analysis revealed that, in DD mice, the firing rate and %SFB of dopamine neurons were significantly inhibited beginning 5 min after anesthesia (P < 0.001 for blocks 4-11 compared with DD VEH), whereas controls did not significantly differ over time. L-dopa did not affect the firing rate or %SFB in anesthetized DD or control mice (data not shown).
Discussion
The objectives of these studies were to characterize the basal firing properties of dopamine neurons in DD and control mice and to determine how endogenously released dopamine influences the activity of dopamine neurons in freely moving mice. The main findings are as follows: (i) that the basal firing properties of dopamine neurons are indistinguishable among genotypes; (ii) that endogenously released dopamine (L-dopa studies) profoundly inhibits the firing rate of a majority of dopamine neurons in DD mice; (iii) that dopamine neurons in DD mice are hypersensitive to systemic activation of D2Rs and D1Rs; and (iv) that anesthesia markedly blunts the firing rate and bursting of dopamine neurons in DD mice but has little effect in control mice.
Dopamine Depletion Does Not Alter the Basal Firing Properties of Dopamine Neurons. Putative dopamine neurons had waveforms that were 1-2 msec in duration. These durations are shorter than previous reports of 3-5 msec in rats (2, 5, 22). The discrepancy may be due to the filter settings used here, which are appropriate for recordings from awake animals but differ from those typically used with anesthetized preparations. In agreement with our findings, a recent study that recorded the activity of dopamine neurons extracellularly from freely moving rats reported a mean action potential duration of 1.5 msec (6). We observed no differences in the basal firing properties of dopamine neurons in control and DD mice (20-24 h after the last L-dopa injection). Overall, the average firing rates of dopamine neurons in mice were comparable to those reported by others in vivo (2-4, 21-24). Similarly, we report that ≈40% of spikes occurred in bursts, a finding that is within the range previously reported (21-59%) in awake rats (6, 23, 37). The mean ISI and autocorrelograms (indicators of the firing patterns of neurons) were also similar between genotypes, suggesting that dopamine neurons in DD mice fire with the appropriate rate and pattern, but because dopamine is not being released, they are essentially “firing blanks.” We suggest that, even in the absence of dopamine-mediated feedback modulation, intact excitatory glutamatergic inputs result in normal firing properties of dopamine neurons. Given the evidence that under normal conditions the local release of dopamine provides an inhibitory tone by means of D2 autoreceptors, one might expect the firing rate of dopamine neurons to be elevated in DD mice, compared with control mice (33). Our results may be explained by compensation within the neural circuitry in DD mice. For example, absence of local autoreceptor inhibition may be offset by increased basal activity of GABA containing striatal projection neurons as reported in 6-hydroxydopamine-lesioned rats (38, 39).
Dopamine Neurons in DD Mice Are Hypersensitive to Dopamine Receptor Activation. Here we establish that dopamine neurons in DD mice are hypersensitive to L-dopa, D1R, and D2R activation. D1R activation potently inhibited firing of dopamine neurons at concentrations that had no effect in controls, in agreement with results obtained with reserpine-treated rats (25, 26). Similarly, relative to controls, dopamine neurons in DD mice were ≈3-fold more sensitive to D2R activation. These findings extend our previous observations that DD mice are hyperactive in response to D1R and D2R stimulation and show elevated immediate early gene expression in the striatum in response to D1R activation (19, 20, 28).
Although the experiments described here cannot distinguish between short-loop feedback pathways (i.e., D2 autoreceptor-mediated inhibition) and long-loop feedback pathways (i.e., inhibition of dopamine neurons by striatonigral projection neurons), evidence suggests that the latter is more important. D1Rs are localized on striatonigral GABAergic neurons that synapse directly onto dopamine neurons (41, 42). Further, D1R-induced inhibition of dopamine neuron firing in reserpine-treated rats is not observed when the agonist is applied directly to the midbrain and is eliminated by hemitransection, implicating the long-loop feedback pathway (40). The response of dopamine neurons to D2 autoreceptor activation is most likely due to a combination of short- and long-loop feedback pathways. Because D2 autoreceptors are unoccupied in DD mice, part of the difference in response between genotypes is likely due to activation of unoccupied D2 autoreceptors in DD mice (whereas D2 autoreceptors are presumably occupied in control mice) and partially due to D2R-bearing striatal projection neurons that initiate an indirect, long-loop feedback pathway. Because dopamine neurons in slices from DD mice mount a normal response to D2R activation at several doses, the effect observed here is most likely due to hypersensitive D2R-bearing striatal projection neurons (29). Thus, as reported in the rat, we suspect that regulation by afferents from supersensitive striatal neurons to dopamine neurons represents a likely mechanism of feedback inhibition that dampens dopamine neuron activity.
Anesthesia Markedly Suppresses Dopamine Neuron Activity in DD Mice. Previously, we reported that dopamine neurons in anesthetized DD mice do not fire in bursts. Because we found different results in freely moving DD mice (≈42% of spikes were fired in bursts; Table 1), we were interested in comparing the firing rates and %SFB in both states (awake and anesthetized) in the same animal. We chose KXA as the anesthetic based on evidence that it had little effect on the basal firing rate or the responsiveness of dopamine neurons to dopaminergic compounds (43). However, KXA profoundly inhibited the firing rate and the %SFB of dopamine neurons in DD mice but had little effect in neurons from control mice. At present, we do not know which components of KXA are responsible for the effect. Nevertheless, these data underscore the importance of measuring the activity of dopamine neurons in awake animals.
Paradoxical Effects of l-Dopa in DD Mice. Our main objective was to characterize the firing rate and bursting of dopamine neurons in DD mice before and after restoration of dopamine signaling. We considered three possibilities as described in the Introduction. We found that systemic L-dopa administration markedly decreased but did not eliminate the firing rate and bursting of dopamine neurons in DD mice while simultaneously inducing hyperphagia and hyperlocomotion. As in the case of D1R and D2R agonists, the inhibition of dopamine neurons is probably a consequence of hypersensitive striatal feedback pathways. We encountered a subset of dopamine neurons that were unaffected by L-dopa treatment (but were inhibited by the confirmation drugs). Possibly the population of non-L-dopa-responsive neurons express D2 autoreceptors but are innervated by relatively few striatonigral afferents. Although L-dopa and dopamine receptor agonists both decreased the activity of dopamine neurons, there is an important difference between the effects of L-dopa, which elicits feeding, and dopamine agonists, which do not. L-dopa permits the synthesis, vesicular transport, and regulated release of dopamine, with transient receptor activation, whereas chronic receptor occupancy occurs with agonists. Thus, we suggest that, despite the overall decrease in firing rate and bursting after L-dopa treatment, the residual regulated release of dopamine may be necessary for complex behaviors such as feeding.
Acknowledgments
We thank K. Nagata and N. Meneses for animal care and J. Williams and N. Bamford for helpful comments. This work was supported in part by Institutional Grant for Neurobiology GM07108-29 (to S.R.), National Institutes of Health Grant MH67399 (to D.M.S.), and National Institute of Mental Health Grant MH58755 (to S.J.Y.M.).
Notes
Abbreviations: DD, dopamine-deficient; D1R, dopamine receptor D1; D2R, dopamine receptor D2; ETIC, eticlopride; ISI, interspike interval; KXA, ketamine/xylazine/acepromazine; %SFB, percentage of spikes fired in bursts; QUIN, quinpirole; SCH, SCH 23390; SKF, SKF 81297; VEH, vehicle.
See Commentary on page 13103.
References
Articles from Proceedings of the National Academy of Sciences of the United States of America are provided here courtesy of National Academy of Sciences
Full text links
Read article at publisher's site: https://doi.org/10.1073/pnas.0405084101
Read article for free, from open access legal sources, via Unpaywall:
https://europepmc.org/articles/pmc516529?pdf=render
Citations & impact
Impact metrics
Citations of article over time
Article citations
A mathematical model for the role of dopamine-D2 self-regulation in the production of ultradian rhythms.
PLoS Comput Biol, 20(5):e1012082, 03 May 2024
Cited by: 0 articles | PMID: 38701077 | PMCID: PMC11095719
Neurovascular effects of cocaine: relevance to addiction.
Front Pharmacol, 15:1357422, 22 Feb 2024
Cited by: 0 articles | PMID: 38455961 | PMCID: PMC10917943
Review Free full text in Europe PMC
Zinc Transporter ZnT1 mRNA Expression Is Negatively Associated with Leptin Serum Concentrations but Is not Associated with Insulin Resistance or Inflammatory Markers in Visceral Adipose Tissue.
Biol Trace Elem Res, 202(12):5319-5327, 06 Feb 2024
Cited by: 0 articles | PMID: 38319549
Biochemically functionalized probes for cell-type-specific targeting and recording in the brain.
Sci Adv, 9(48):eadk1050, 29 Nov 2023
Cited by: 2 articles | PMID: 38019917 | PMCID: PMC10686571
The dopamine circuit as a reward-taxis navigation system.
PLoS Comput Biol, 18(7):e1010340, 25 Jul 2022
Cited by: 4 articles | PMID: 35877694 | PMCID: PMC9352198
Go to all (54) article citations
Data
Similar Articles
To arrive at the top five similar articles we use a word-weighted algorithm to compare words from the Title and Abstract of each citation.
Effects of full D1 dopamine receptor agonists on firing rates in the globus pallidus and substantia nigra pars compacta in vivo: tests for D1 receptor selectivity and comparisons to the partial agonist SKF 38393.
J Pharmacol Exp Ther, 286(1):272-281, 01 Jul 1998
Cited by: 51 articles | PMID: 9655869
Nigrostriatal lesion and dopamine agonists affect firing patterns of rodent entopeduncular nucleus neurons.
J Neurophysiol, 88(1):487-496, 01 Jul 2002
Cited by: 34 articles | PMID: 12091570
D1 dopamine receptor stimulation enables the postsynaptic, but not autoreceptor, effects of D2 dopamine agonists in nigrostriatal and mesoaccumbens dopamine systems.
Synapse, 4(4):327-346, 01 Jan 1989
Cited by: 95 articles | PMID: 2532422
Functional anatomical and behavioral consequences of dopamine receptor stimulation.
Ann N Y Acad Sci, 835:153-156, 01 Dec 1997
Cited by: 12 articles | PMID: 9616770
Review
Funding
Funders who supported this work.
NIGMS NIH HHS (2)
Grant ID: GM07108-29
Grant ID: T32 GM007108
NIMH NIH HHS (5)
Grant ID: MH67399
Grant ID: R56 MH058755
Grant ID: R01 MH058755
Grant ID: F32 MH067399
Grant ID: MH58755