Abstract
Free full text

Paracrine cross-talk between skeletal muscle and macrophages in exercise by PGC-1α-controlled BNP
Abstract
Activation of resident and infiltrating immune cells is a central event in training adaptation and other contexts of skeletal muscle repair and regeneration. A precise orchestration of inflammatory events in muscle fibers and immune cells is required after recurrent contraction-relaxation cycles. However, the mechanistic aspects of this important regulation remain largely unknown. We now demonstrate that besides a dominant role in controlling cellular metabolism, the peroxisome proliferator-activated receptor γ co-activator 1α (PGC-1α) also has a profound effect on cytokine expression in muscle tissue. Muscle PGC-1α expression results in activation of tissue-resident macrophages, at least in part mediated by PGC-1α-dependent B-type natriuretic peptide (BNP) production and secretion. Positive effects of exercise in metabolic diseases and other pathologies associated with chronic inflammation could accordingly involve the PGC-1α-BNP axis and thereby provide novel targets for therapeutic approaches.
Skeletal muscle plasticity in response to acute bouts of exercise involves sterile tissue inflammation. A tightly coordinated activation of tissue-resident and infiltrating immune cells is instrumental for proper muscle repair and regeneration after these recurring contraction-relaxation cycles. During this process, macrophages polarize into the M1-type inducing a pro-inflammatory cytokine profile to stimulate tissue clean-up1. This is followed by a second wave of M2 macrophage polarization that is linked to a predominantly anti-inflammatory milieu to boost tissue repair and regeneration1. In contrast to the transient inflammation after exercise, a persistent, low-grade elevation of pro-inflammatory factors, both locally in tissues as well as systemically, is closely associated with a number of chronic diseases, including the metabolic syndrome or cardiovascular pathologies1. Exercise combined with other life-style interventions efficiently prevents or ameliorates many of these diseases2,3,4. In response to exercise, skeletal muscle cells secrete various factors, so-called myokines, which elicit responses in an auto-, para- or endocrine manner5,6,7. Although several factors are known to be secreted by exercised muscles, our understanding of the cross-talk between muscle fibers and other cell types in exercise or disease remains rudimentary7. In particular, it is unclear whether immune cell activation in muscle tissue is a secondary event triggered by fiber damage or if muscle fibers exert direct control on tissue-resident macrophages.
The peroxisome proliferator-activated receptor γ (PPARγ) co-activator 1α (PGC-1α) is a central regulator of endurance training adaptation in skeletal muscle. PGC-1α induces genes involved in mitochondrial biogenesis, oxidative phosphorylation, vascularization and the contractile apparatus to enable a higher endurance capacity by co-activating an array of nuclear receptors and other transcription factors in a poorly understood complex transcriptional network8,9. In addition to the effects on energy metabolism in response to exercise, PGC-1α also has an immunomodulatory role1. In cultured muscle cells, overexpression of PGC-1α exerts a dampening of nuclear factor κB activity and consequently a suppression of pro-inflammatory gene expression upon treatment with tumor necrosis factor α (TNFα), saturated fatty acids or specific activators of the toll-like receptors 1/2, 4 and 6/210. In contrast, specific PGC-1α overexpression in muscle fibers in vivo was not able to reduce the strong, acute inflammation induced by TNFα or bacterial lipopolysaccharide (LPS), indicating a cross-talk of different cell types to overcome the cell autonomous anti-inflammatory effect of PGC-1α in muscle tissue11. These findings imply a multifaceted role for muscle PGC-1α to modulate local inflammation that extends beyond cell autonomous effects with important implications for our understanding of muscle cell plasticity in exercise and disease.
In the present study, we therefore explored the effects of exercise and PGC-1α in skeletal muscle in vivo on inflammation and in particular the possible cross-talk between muscle cells and macrophages. By combining in vivo experiments with in vitro sectretome investigations, we identified B-type natriuretic peptide (BNP) as a novel PGC-1α-dependent myokine that mediates cross-talk with tissue macrophages in skeletal muscle.
Results
Exercise and PGC-1α overexpression increase pro- and anti-inflammatory cytokines in skeletal muscle
As a first step to investigate muscle-immune cell cross-talk, expression levels of pro-inflammatory cytokines associated with M1 macrophage activation (i.e. TNFα, interleukin 6 (IL-6), macrophage inflammatory protein 1α (MIP-1α), monocyte chemoattractant protein 1 (MCP-1) and IL-12) and anti-inflammatory cytokines associated with M2 activation (i.e. C-C motif chemokine 22 (CCL22), IL-1Ra, transforming growth factor β (TGFβ) and IL-10) were determined in quadriceps muscle of mice after a bout of endurance exercise to exhaustion. A change in the expression levels of some of these pro- and anti-inflammatory genes was indeed observed in response to exercise (Fig. 1A and B).
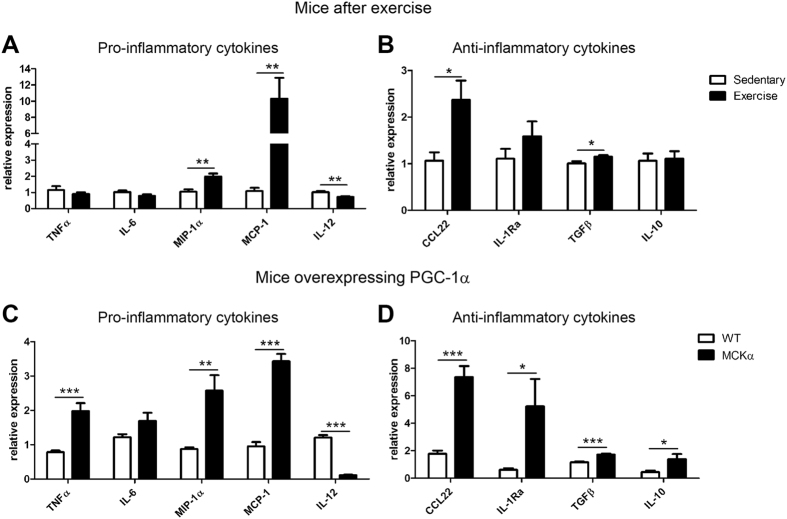
(A–D) Relative expression levels of pro- and anti-inflammatory cytokines were analyzed by RT-PCR in quadriceps muscle after exercise (panel A and B) and in MCKα mice (panel C and D). Values represent the mean of at least 6 animals +SEM. *P<
0.05; **P
<
0.01; ***P
<
0.001; exercise versus sedentary, MCKα versus WT.
We then mapped the cytokine environment in the quadriceps muscle of sedentary PGC-1α muscle-specific transgenic mice (MCKα) and their wild-type littermates (WT) to study whether muscle-specific overexpression of PGC-1α induces a similar cytokine profile as exercise. In MCKα mice, expression of pro-inflammatory cytokines TNFα, MIP-1α and MCP-1 was elevated whereas IL-6 mRNA expression was similar to WT mice (Fig. 1C). As reported previously11, IL-12 levels were strongly suppressed by PGC-1α overexpression. In contrast to the mixed M1 cytokine expression pattern, all of the tested M2 cytokines were significantly augmented by PGC-1α overexpression (Fig. 1D). Our data demonstrate that exercise and PGC-1α alone induce the expression of both pro- and anti-inflammatory cytokines in skeletal muscle. Importantly, even though a strong anti-inflammatory pattern of cytokine expression correlating to an M2-type polarization of macrophages is observed, the increase in some pro-inflammatory cytokines suggests that muscle PGC-1α could generally prime macrophages for rapid action after an exercise bout, in which an M1 to M2 transition has to be tightly orchestrated to ensure proper repair and regeneration.
Cell autonomous and non-autonomous effects of muscle PGC-1α in vitro
Due to the high complexity of skeletal muscle tissue in vivo, it was not possible to distinguish muscle-derived cues from those produced and secreted by cells of the immune system in our experimental settings. We therefore analyzed cytokine mRNA levels in cultured C2C12 myotubes overexpressing either PGC-1α or GFP. Transcript levels of IL-10 were below the detection limit of the qPCR assay and thus most likely derived from immune cells in vivo. CCL22 and TGFβ were detectable, albeit at very low amounts. These genes were however not regulated by PGC-1α in cultured muscle cells as observed in muscle tissue in vivo (Fig. 2A). These results imply that in vivo, immune rather than muscle cells are the main source of these cytokines. In contrast, the induction of IL-1Ra and the strong suppression of IL-12 observed in MCKα mice in vivo were recapitulated in the cellular system upon PGC-1α overexpression (Fig. 2B). Thus, the very low IL-12 levels detected in muscle of transgenic mice are likely due to very low expression of this cytokine in muscle cells themselves.
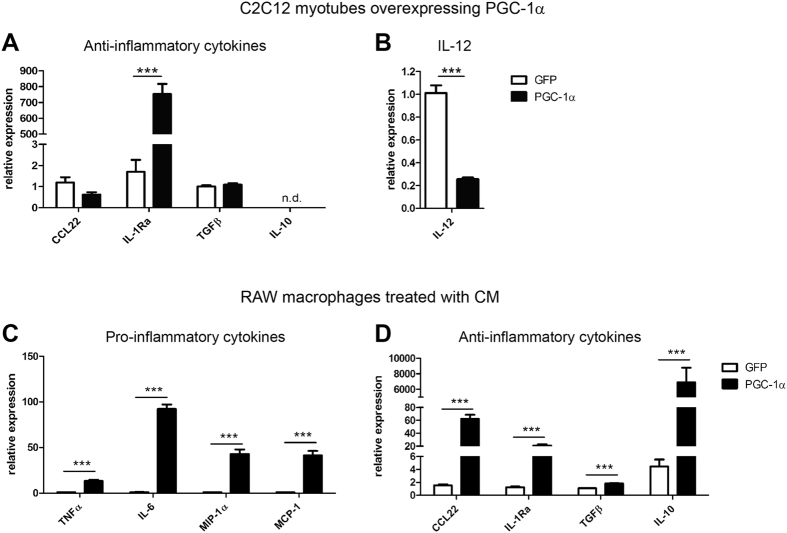
(A,B) mRNA expression of anti-inflammatory cytokines (A) and IL-12 (B) in C2C12 myotubes adenovirally overexpressing PGC-1α or GFP. (C,D) RAW macrophages were treated with conditioned medium of C2C12 myotubes overexpressing PGC-1α or GFP and expression levels of pro- (C) and anti-inflammatory (D) cytokines were determined. Values represent the mean of at least 3 independent experiments +SEM. ***P<
0.001; PGC-1α versus GFP. See also Figure S1.
To dissect the role of muscle PGC-1α on pro- and anti-inflammatory cytokine expression of macrophages, RAW macrophages were treated with conditioned medium (CM) of C2C12 myotubes overexpressing PGC-1α (or GFP as control). After 4hours, both M1 and M2 cytokine expression was augmented in RAW macrophages (Fig. 2C and D). These results show that in vitro, muscle PGC-1α increases the production and secretion of soluble factors that subsequently activate a pro- and an anti-inflammatory response in macrophages.
PGC-1α induces the secretion of proteins from muscle cells to activate macrophages
PGC-1α regulates a number of myokines in skeletal muscle7. The effects of such known myokines on M1 and M2 cytokine expression were tested by treating RAW macrophages with irisin, IL-15, brain-derived neurotrophic factor (BDNF), the chemokine C-X-C motif ligand 1 (CXCL1), CXCL2 or CXCL5 (the latter three are mouse IL-8 homologues). These different myokines only induced mild changes in M1 and M2 expression levels and were thereby not likely to account for the strong effects observed after CM treatment (Fig. S1A).
To identify other factors secreted upon PGC-1α expression, mass spectrometry of CM was performed to screen the muscle cell secretome in an unbiased manner. This proteomic approach identified several proteins that potentially contribute to the cross-talk between muscle cells and macrophages (Table S2). Several chemokines (CCL2/MCP-1, CCL7, CXCL1, CXCL5 and CXCL10), of which an immunomodulatory role is already known, were among the candidate proteins (Table 1). Their relative gene expression was verified in C2C12 myotubes and as expected, the transcript levels of these chemokines were strongly induced (Fig. 3A). In vivo however, only MCP-1 and CCL7 expression was higher in MCKα mice compared to WT animals (Fig. 3B).
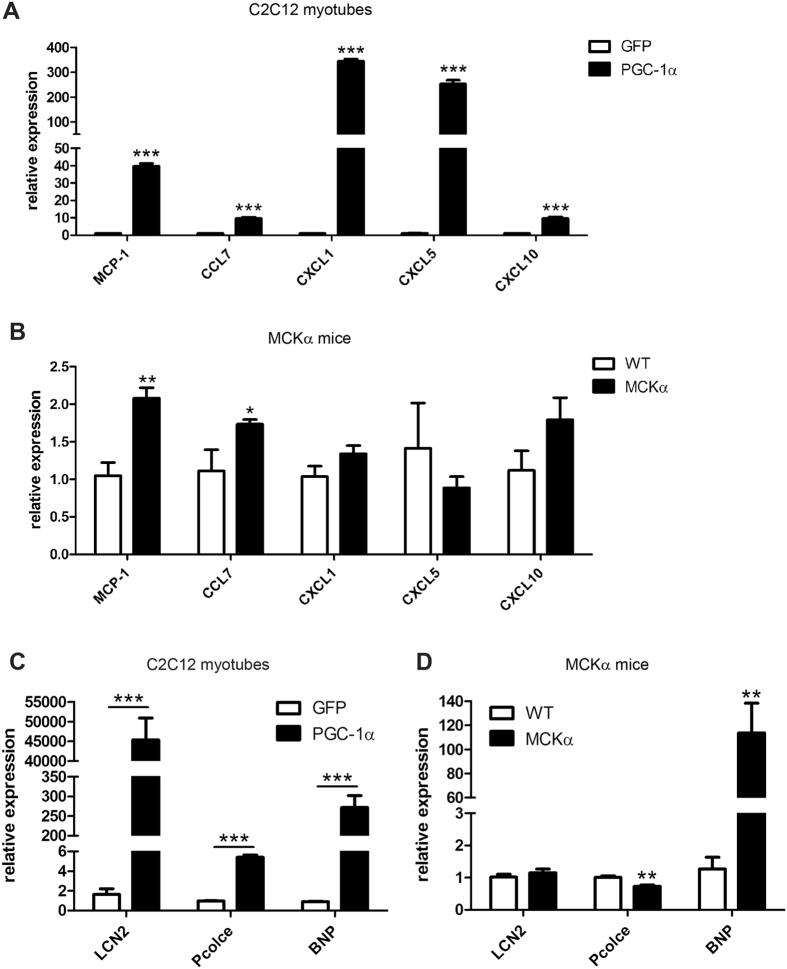
(A,B) mRNA expression levels of chemokines identified in the CM of C2C12 myotubes overexpressing PGC-1α by mass spectrometry were validated in C2C12 myotubes overexpressing PGC-1α (A) and MCKα mice (B). (C,D) Other identified factors from the CM potentially involved in the muscle-macrophage cross-talk were validated by RT-PCR. mRNA expression levels of LCN2, Pcolce and BNP were determined in C2C12 myotubes adenovirally overexpressing PGC-1α or GFP (C) and in WT and MCKα mice (D). Values represent the mean of at least 3 independent experiments or at least 6 animals +SEM. *P<
0.05; **P
<
0.01; ***P
<
0.001; PGC-1α versus GFP, MCKα versus WT.
Table 1
Gene symbol | Gene name | Number of peptides used for quantification | Fold-change protein PGC-1α/GFP | q-value protein PGC-1α/GFP |
---|---|---|---|---|
CCL2/MCP-1 | C-C motif chemokine 2 | 2 | 42.38*** | 0.015 |
CCL7 | C-C motif chemokine 7 | 6 | 20.18*** | 0.028 |
CXCL1 | C-X-C motif chemokine 1 | 4 | 69.99*** | 0.015 |
CXCL5 | C-X-C motif chemokine 5 | 6 | 26.34*** | 0.015 |
CXCL10 | C-X-C motif chemokine 10 | 2 | 62.43** | 0.102 |
LCN2 | Neutrophil gelatinase-associated lipocalin | 1 | 115.45*** | 0.025 |
Pcolce | Procollagen C-endopeptidase enhancer 1 | 22 | 5.22** | 0.110 |
Nppb/BNP | Natriuretic peptides B | 2 | 10.62** | 0.146 |
**p<
0.01; ***p
<
0.001.
In addition to these chemokines, our analysis of CM from PGC-1α-overexpressing myotubes revealed other, non-chemokine proteins (Table 1). Among these, the 3 most interesting candidate proteins within the secretome were lipocalin 2 (LCN2), procollagen C-endopeptidase enhancer 1 (Pcolce) and B-type (or brain) natriuretic peptide (BNP, gene name Nppb). The induction of these candidate genes by PGC-1α was validated in cultured myotubes and mice (Fig. 3C and D). In contrast to the elevation of all three genes in C2C12 myotubes overexpression PGC-1α, only BNP expression was enhanced in muscle-specific PGC-1α transgenic mice in vivo. To compare whether these candidates would elicit a similar response as CM from PGC-1α-overexpressing myotubes, RAW macrophages where directly treated with recombinant LCN2, Pcolce or BNP. Of these three candidates, BNP pronouncedly increased the expression of M1 and M2 cytokines, whereas LCN2 and Pcolce had no effect (Fig. 4A and B). Importantly, BNP-induced cytokine expression was dose-dependent and both qualitatively and quantitatively closely resembled CM treatment (Fig. S2). These results indicate that BNP plays an important role in stimulating the expression of M1 and M2 cytokines in macrophages.
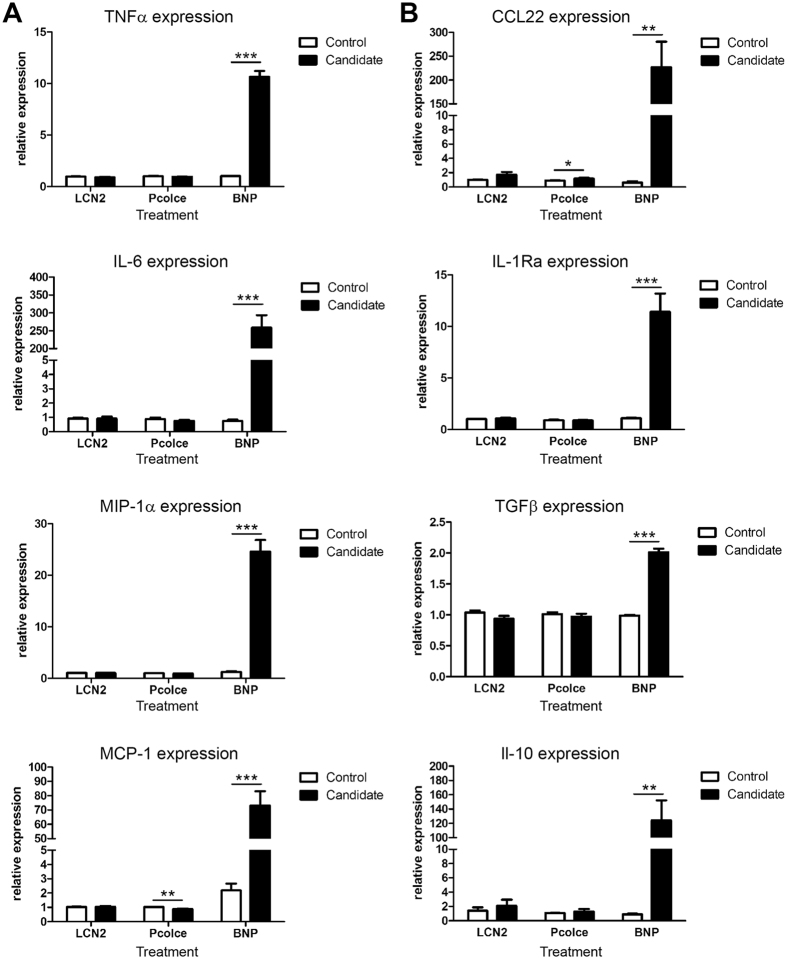
(A,B) RAW macrophages were treated for 4h with LCN2, Pcolce or BNP. Relative expression of pro- (A) and anti-inflammatory (B) cytokines was determined by RT-PCR. Values represent the mean of at least 3 independent experiments +SEM. **P
<
0.01; ***P
<
0.001; candidate versus control. See also Figure S2.
BNP is a PGC-1α-dependent myokine
Our results show that BNP may be responsible for para-crine effects of PGC-1α on macrophages. The high transcript levels of BNP in C2C12 myotubes overexpressing PGC-1α strongly suggest that BNP is indeed regulated by PGC-1α. To further elucidate the epistasis between these two genes, the BNP promoter region was scanned for potential PGC-1α response elements based on previous work on the PGC-1α-controlled transcriptional network in muscle cells12. The activator protein-1 (AP-1) transcription factor protein complex, which binds to Fos-Jun-like motifs, has two predicted binding sites in the genomic region flanking the BNP transcriptional start site (TSS), and is co-activated by PGC-1α12. Accordingly, siRNA-based silencing of Fos and Jun strongly reduces the ability of PGC-1α to induce BNP expression12.
Several PGC-1α peaks, located upstream of the BNP TSS were identified using ChIPseq data of global PGC-1α DNA recruitment to the mouse genome in muscle cells12 (Fig. 5A). Intriguingly, the absence of a PGC-1α peak in the proximal promoter region of BNP suggests that the AP-1 binding site in this region is indirectly activated by PGC-1α. Within the most prominent peak that is located ~7 kilo base (kb) upstream of the BNP TSS, two binding motifs for estrogen-related receptor α (ERRα, ESRRA motif) and one binding site for AP-1 were predicted. Analyses of ERRα ChIP-Seq data13 revealed an overlap of a PGC-1α and an ERRα peak at this genomic location, strongly suggesting co-recruitment of these two proteins. Potent inhibition of ERRα with shRNA and the inverse agonist XCT79014 resulted in a marked reduction of PGC-1α-mediated BNP expression establishing a functional relationship between PGC-1α and ERRα in the control of BNP transcription (Fig. 5B). Collectively, these results suggest that PGC-1α regulates BNP gene expression in conjunction with AP-1 and ERRα, similarly to what has been described for vascular endothelial growth factor and other PGC-1α target genes8.
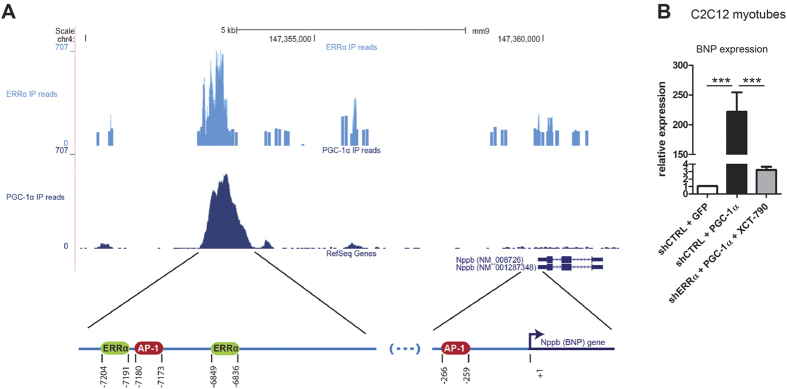
(A) Overlapping ChIP-Seq peaks of ERRα (light blue) and PGC-1α (dark blue) are located ~7kb upstream of the Nppb (BNP) RefSeq transcripts and depicted as read densities. Predicted binding sites for AP-1 and ERRα are represented by red and light green circles, respectively, and their genomic positions are referred to the BNP transcription start site (TSS) (B) Relative expression levels of BNP in response to PGC-1α overexpression and shERRα knock-down, in addition to the inverse agonist XCT-790 were determined by RT-PCR. Values represent the mean of at least 3 independent experiments +SEM. ***P
<
0.001.
BNP is regulated by PGC-1α and exercise in muscle in vivo
We next studied the regulation and function of BNP in skeletal muscle in vivo. As shown in Fig. 3D, muscle-specific overexpression of PGC-1α in mouse muscle strongly promoted BNP gene expression. Then, we assessed the potential of BNP to modulate the activation of tissue-resident macrophages in muscle by injecting recombinant BNP into TA muscles of WT animals (or Pcolce and PBS as negative and vehicle control, respectively). Recapitulating the in vitro data, BNP injection increased the expression of TNFα, IL-6, MIP-1α, MCP-1 and of all tested M2 cytokines (Fig. 6A and B). In contrast, IL-12 expression was unaffected by BNP treatment, which reinforces the hypothesis of IL-12 to be inhibited by PGC-1α in muscle cells. As observed in vitro, Pcolce did not have any effect on M1 or M2 cytokine expression levels. Notably, the chemokines identified in the CM were also strongly elevated in the BNP-injected TA (Fig. 6C), implying a central role for BNP in the hierarchical control of chemokine expression in muscle.
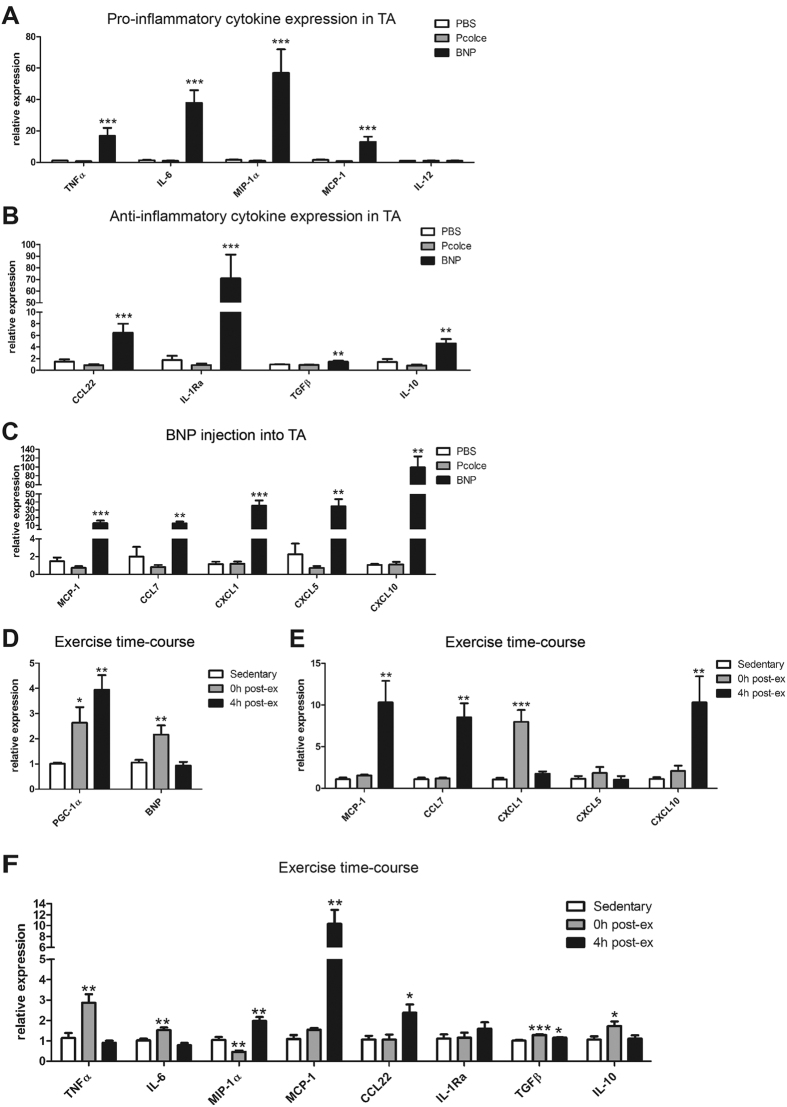
(A,B) Four hours after i.m. injection of BNP, Pcolce or PBS into TA muscle, mRNA expression of pro- (A) and anti-inflammatory (B) cytokines was determined. (C) Chemokine expression levels were also measured after i.m. injections and were largely increased. (D–F) An exercise test to exhaustion was performed and quadriceps muscle was analyzed immediately (0h post-exercise) and 4
h post-exercise. Time courses of PGC-1α and BNP (D), chemokines and pro- and anti-inflammatory cytokines (F) expression were determined. Values represent the mean of at least (E) 6 animals +SEM. *P
<
0.05; **P
<
0.01; ***P
<
0.001.
Finally, we investigated whether exercise, a physiological context of PGC-1α induction, also increases the expression of BNP in skeletal muscle in vivo. For that purpose, an exhaustion exercise test was performed and BNP levels analyzed in quadriceps muscle. In exercised animals, the induction of PGC-1α gene expression was paralleled by an increase in BNP transcript levels immediately post-exercise (Fig. 6D). While BNP expression was reduced to baseline levels 4h post-exercise, PGC-1α levels further increased and possibly activated other gene programs. Intriguingly, the chemokines CCL7, CXCL1 and CXCL10, which were identified in the CM of C2C12 myotubes overexpressing PGC-1α, also exhibited a time-dependent elevation post-exercise (Fig. 6E). Since the activity of M1 and M2 macrophages is tightly coordinated for proper muscle repair and regeneration post-exercise, we also determined how the expression levels of M1 and M2 cytokines were regulated immediately and 4
h after exercise cessation (Fig. 6F). Immediately after exercise, TNFα, IL-6, TGFβ and IL-10 were also elevated. The expression of the cytokines that were induced immediately after exercise dropped back to baseline levels after 4
h, while the expression of MIP-1α, MCP-1 and CCL22 only started to rise at this later time point. This time course experiment demonstrates that several M1 and M2 cytokines are differentially induced in response to exercise, and only some follow the immediate early transcription pattern of PGC-1α, most notable BNP.
Discussion
Exercise, muscle regeneration and metabolism are intrinsically linked to inflammatory events. We now demonstrate that muscle PGC-1α, a key regulator of skeletal muscle plasticity in endurance training, not only modulates inflammation in muscle fibers, but also in tissue-resident immune cells by controlling paracrine myokine signaling. Interestingly, a PGC-1α-dependent reduction in muscle cell inflammation was not only shown in previous experiments in cultured muscle cells10, but is further highlighted by the specific and strong inhibition of IL-12 in muscle fibers observed in the present study. In addition to the cell autonomous effects, muscle PGC-1α triggers a paracrine signaling to activate tissue-resident macrophages. Importantly, even though a strong anti-inflammatory pattern of cytokine expression representing an M2-type polarization is observed, the less robust increase in M1, pro-inflammatory cytokine suggests that muscle PGC-1α could generally prime macrophages for rapid action, in which an M1 to M2 transition has to be tightly orchestrated to ensure proper repair and regeneration. An increased regenerative capacity in muscles overexpressing PGC-1α has recently been postulated15. We have shown that in addition to the increase of PGC-1α expression immediately and 4h post-exercise, the expression of several chemokines and cytokines was also elevated 0
h and 4
h after exercise. The time-dependent changes in expression levels of these cytokines suggest that tissue macrophages are activated immediately after an acute bout of exercise. However, the exact time course of macrophage infiltration in response to endurance exercise is not well established. Interestingly, sustained overexpression of PGC-1α in muscle stimulates macrophage infiltration, as demonstrated by the higher number of tissue-resident macrophages in muscles of MCKα mice with a predominant M2 phenotype16,17. Muscle PGC-1α could thereby be indicative of the higher resistance of trained muscle fibers against damage and the more efficient repair and regeneration process18,19 in a physiological context with consistently elevated PGC-1α gene expression. Indeed, the higher proliferative rate of satellite cells in MCKα mice further supports this hypothesis15. However, the exact chain of events will have to be elucidated in a temporal analysis in animal models in vivo after acute exercise and chronic training that both reflect the pulsatile induction of PGC-1α after each exercise bout and the persistent increase in basal PGC-1α expression linked to the fiber-type switch after prolonged endurance training20.
PGC-1α regulates a number of myokines in skeletal muscle7, at least some of which were not able to activate cultured macrophages. Based on an unbiased secretome analysis of conditioned media obtained from cultured skeletal muscle cells overexpressing PGC-1α, candidate proteins were tested and BNP identified as a strong mediator of macrophage activation, both in terms of M1 and M2 prototypical cytokine expression. BNP is mainly known to be synthesized and secreted by cardiac ventricles in response to stretch in order to initiate signaling to reduce blood pressure and blood volume21,22,23. The administration of BNP can be used to treat patients with heart failure to reduce additional stress and possibly also fibrosis in the heart24. Importantly however, BNP also affects non-cardiac tissue, such as the liver, fat and skeletal muscle tissue25,26,27,28. Similar to the observations in the heart, transgenic overexpressing of BNP is able to reduce liver fibrosis28, indicating a more general role for BNP in inflammation linked to tissue repair and regeneration. An immunomodulatory role of BNP in macrophages is further implied by experiments demonstrating that stimulation of THP-1 macrophages with BNP results in increased ROS, NO2 and leukotriene B4 production29. Notably, besides the pro-inflammatory effects, the anti-inflammatory prostaglandin 2 and IL-10 levels were also increased by BNP in this context29, which is reminiscent of our findings in skeletal muscle. Our data now suggest that the PGC-1α-induced elevation of BNP plays a role in the cross-talk between skeletal muscle and macrophages and could thereby also influence inflammation, repair and regeneration of this tissue after exercise.
In a different study, the secreted phosphoprotein 1 (SPP1) was identified as a mediator of muscle PGC-1α-triggered macrophage activation leading to endothelial remodeling and neovascularization17. Curiously, SPP1 was not found in our mass spectrometric secretome analysis. It is unclear whether the divergent results are based on differences in the experimental models or if engagement of BNP and SPP1 might be context-dependent and geared towards macrophage activation in exercise-linked repair and regeneration vs. vascularization, respectively. However, BNP and SPP1 seem to have a similar effect on macrophages, as treatment of macrophages with BNP or SPP1 both increased the expression of MCP-1. It is obviously conceivable that both myokines are induced by muscle PGC-1α in a redundant manner to ensure robust activation of immune cells.
Furthermore, BNP was shown to induce a browning phenotype and enhanced lipolysis in cultured adipocytes26,30, increase energy expenditure26, as well as to reduce the increase in fat mass in response to a high fat diet (HFD) in mice27. In addition, chronic BNP infusion improved glucose tolerance and insulin signaling in HFD-fed and obese mice, respectively due to a reduction in lipotoxicity within the muscle31. Therefore, it is not surprising that BNP levels are inversely correlated with BMI32, indicating that the possible protective effects of BNP against obesity and diabetes are blunted in obese individuals with lower circulating BNP33. This large variety of effects of BNP suggests that BNP plays a role in mediating some of the health beneficial effects observed in response to exercise. In humans, plasma BNP levels are increased immediately after exercise34,35. Due to the much higher expression of BNP in cardiac compared to skeletal muscle, the bulk of the circulating BNP could originate from the heart. However, 8hours post-exercise, mRNA expression of the natriuretic peptide receptor A (NPR-A) is elevated in skeletal muscle25. Moreover, a myokine named musclin was recently described to be induced by exercise and to activate the same pathway as natriuretic peptides36. In particular, due to the binding of musclin to the clearance receptor NPR-C, plasma levels of NPs remain higher after exercise and can thereby elicit their positive effects on whole body metabolism in a more potent manner36. Intriguingly, musclin function has also been linked to exercise-induced PGC-1α gene expression in skeletal muscle. Together, these results underline the significance of a PGC-1α-BNP axis in paracrine signaling in exercised skeletal muscle that we postulate. Based on our findings and those published previously, it is conceivable that BNP is one important component of a larger cocktail of PGC-1α-controlled myokines with paracrine immunomodulatory function in skeletal muscle (Fig. 7). Whether a link between PGC-1α and BNP in muscle has systemic consequences remains to be elucidated. However, such a regulation might also occur in cardiac tissue with high PGC-1α expression and could thereby be important for the metabolic effects of BNP on liver, adipose tissue and potentially other metabolic organs.
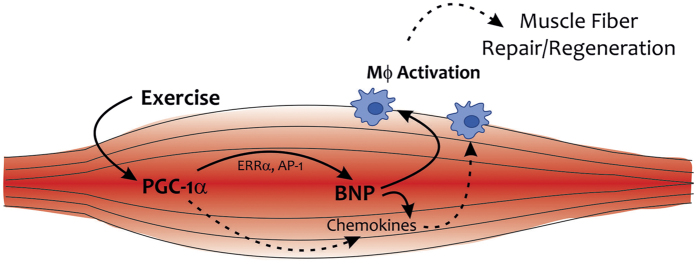
PGC-1α increases BNP expression by co-activating ERRα and/or AP1. BNP subsequently induces a chemokine cocktail in muscle fibers, and activates macrophages in a local, paracrine manner, which then may contribute to the enhanced repair and regeneration potential of trained muscles.
In conclusion, we now describe a novel regulation and function of BNP in exercised skeletal muscle to activate tissue-resident macrophages when induced by PGC-1α and ERRα. Collectively, our results highlight a direct mechanism by which skeletal muscle fibers engage tissue macrophages after contraction. This novel fundamental pathway illustrates how muscle fibers orchestrate macrophage activation and thereby also provides an explanation for the enhanced capacity of trained skeletal muscle to respond to contractile damage. Importantly, this active engagement of immune cells by muscle fibers not only is of high importance for exercise adaptation, but could also be leveraged in the many different muscle diseases that are associated with aberrant inflammation. This axis could be of therapeutic importance in the treatment of muscle wasting diseases to accelerate and improve fiber repair and muscle regeneration.
Methods
Mouse experiments
C57BL/6 mice expressing PGC-1α9 (MCKα) under the control of the muscle creatine kinase (MCK) promoter were bred with respective WT mice to obtain WT and transgenic littermates. Male mice were maintained on a standard rodent chow with 12h light/dark cycle and euthanized at 12 weeks of age when quadriceps muscles were collected for further analysis. Animals within the injection experiment were 15–18 weeks of age. 400ng of BNP (Cloud-Clone Corp) or Pcolce (R&D Systems) in 30
μl PBS were injected i.m. into the tibialis anterior (TA) and PBS as a control on the contralateral side. After 4
h, animals were euthanized and TAs collected for further analysis. Animals performing exhaustion exercise were at the age of 20 weeks. The incremental exercise at a 5° inclination started at a velocity of 10
m/min and was increased every 15
min by 2
m/min until exhaustion. Tissue collection was performed immediately after or 4
h after exercise. All methods were carried out in accordance with relevant guidelines and regulations, and all animal experiments were approved by the Kantonales Veterinäramt Basel-Stadt.
Cell culture
The mouse myoblast cell line C2C12 was maintained below confluence in Dulbecco’s Modified Eagle Medium (DMEM) supplemented with 10% fetal calf serum and 1x Penicillin/Streptomycin (Invitrogen). For differentiation into myotubes, growth medium was exchanged for DMEM supplemented with 2% horse serum (Invitrogen) for 3–4 days. PGC-1α or GFP were overexpressed from recombinant adenoviral constructs 48h prior to harvesting the cells. 24
hours after infection the medium was exchanged for differentiation medium. The knock-down experiment is described elsewhere12.
Conditioned medium was obtained from mouse C2C12 myotubes. After 3–4 days of differentiation, myotubes were infected with PGC-1α or GFP using a recombinant adenoviral construct for 24h. Infection medium was exchanged after 24
h, which was exchanged again after another 24
h for differentiation medium that was used for the conditioned medium. The conditioned medium was removed after 20
h, filtered and stored at −20
°C.
The mouse RAW 264.7 macrophage cell line was maintained in DMEM supplemented with 10% fetal calf serum and 1x Penicillin/Streptomycin. At 80–90% confluence, the RAW macrophages were treated with conditioned medium of C2C12 myotubes for 4h and then dissolved in Trizol for RNA extraction. RAW macrophages were treated for 4
h with 100
ng/ml irisin (AdipoGen), 10
ng/ml IL-15 (R&D Systems), 100
ng/ml BDNF (R&D Systems), 100
ng/ml CXCL1 (R&D Systems), 100
ng/ml CXCL2 (R&D Systems), 100
ng/ml CXCL5 (R&D Systems), 100
ng/ml LCN2 (Cell Signaling Technology), 200
ng/ml Pcolce (R&D Systems) or 1
nM, 2
nM or 4
nM BNP (Cloud-Clone Corp). After the 4
h treatment, the cells were dissolved in Trizol for RNA extraction.
Semi-quantitative real-time PCR
Muscle tissue was homogenized using matrix particles (Qbiogene) in a FastPrep FP120 cell disrupter (Thermo Scientific) and RNA isolated with Trizol (Invitrogen) while residual DNA contamination was removed by DNase I (Invitrogen) digestion. 1μg of RNA was reverse transcribed with SuperScript II (Invitrogen) and the resulting cDNA used as template for RT-PCR. To detect relative expression levels cDNA was amplified with the SYBR Green Master mix (Applied Biosystems) and analyzed on a StepOnePlus RT-PCR System (Applied Biosystems). The respective primer pairs are listed in the Supplemental Table S1. All values were normalized to the expression of TATA-Box binding protein (TBP) and expressed as fold induction over GFP-expressing control cells or WT animals.
Proteomics analysis
For mass spectrometry analyses, serum-free medium was conditioned as described above. Serum-free CM had the same effect on RAW macrophages concerning M1 and M2 cytokine expression as CM with serum (data not shown).
Supernatants of 3 independent experiments containing the secreted proteins were collected and concentrated using ultrafiltration 5kDa cut-off cartridges (Sartorius, VIVASPIN 500) according to the manufacture instructions. Protein samples were washed twice with 400
μl digestion buffer (1
M urea, 0.1
M ammoniumbicarbonate) and concentrated to a final volume of 100
μl. Proteins were reduced with 5
mM TCEP for 60
min at 37
°C, alkylated with 10
mM iodoacetamide for 30
min in the dark at room temperature and digested with trypsin (Promega) at 37
°C overnight (protein to trypsin ratio: 50:1). After digestion, the samples were supplemented with TFA to a final concentration of 0.5%. Then, peptides were desalted on C18 reversed phase spin columns according to the manufacturer’s instructions (Macrospin, Harvard Apparatus), dried under vacuum and stored at −80
°C until further processing.
1µg of peptides of each sample were subjected to LC–MS analysis using a dual pressure LTQ-Orbitrap Velos mass spectrometer connected to an electrospray ion source (both Thermo Fisher Scientific) as described recently with a few modifications37. In brief, peptide separation was carried out using an EASY nLC-1000 system (Thermo Fisher Scientific) equipped with a RP-HPLC column (75
μm
×
30
cm) packed in-house with C18 resin (ReproSil-Pur C18–AQ, 1.9
μm resin; Dr. Maisch GmbH, Ammerbuch-Entringen, Germany) using a linear gradient from 95% solvent A (0.15% formic acid, 2% acetonitrile) and 5% solvent B (98% acetonitrile, 0.15% formic acid) to 28% solvent B over 120
min at a flow rate of 0.2
μl/min. The data acquisition mode was set to obtain one high resolution MS scan in the FT part of the mass spectrometer at a resolution of 60,000 full width at half-maximum (at m/z 400) followed by MS/MS scans in the linear ion trap of the 20 most intense ions using rapid scan speed. The charged state screening modus was enabled to exclude unassigned and singly charged ions and the dynamic exclusion duration was set to 30
s. The ion accumulation time was set to 300
ms (MS) and 25
ms (MS/MS).
For label-free quantification, the generated raw files were imported into the Progenesis LC-MS software (Nonlinear Dynamics, Version 4.0) and analyzed using the default parameter settings. MS/MS-data were exported directly from Progenesis LC-MS in mgf format and searched against a decoy database the forward and reverse sequences of the predicted proteome from mus musculus (UniProt, download date: 22/05/2013, total of 33,984 entries) using MASCOT (version 2.4.). The search criteria were set as follows: full tryptic specificity was required (cleavage after lysine or arginine residues); 3 missed cleavages were allowed; carbamidomethylation (C) was set as fixed modification; oxidation (M) as variable modification. The mass tolerance was set to 10ppm for precursor ions and 0.6
Da for fragment ions. Results from the database search were imported into Progenesis LC-MS and the final peptide measurement list containing the peak areas of all identified peptides, respectively, was exported. This list was further processed and statistically analyzed using our in-house developed SafeQuant R script to obtain protein relative abundances37. This analysis included global data normalization by equalizing the total MS1 peak areas across all LC-MS runs, summation of MS1 peak areas per protein and LC-MS/MS run, followed by calculation of protein abundance ratios. The summarized protein expression values were used for statistical testing of between condition differentially abundant proteins. Here, empirical Bayes moderated t-Tests were applied, as implemented in the R/Bioconductor limma package. The resulting per protein and condition comparison p-values were adjusted for multiple testing using the Benjamini-Hochberg method. The peptide and protein false discovery rate (FDR) was set to 1% using the number of reverse hits in the dataset. All quantified proteins together with their identification and quantification details are listed in Supplemental Table S2.
Data analyses and binding site prediction
Using ChIP-Seq data on PGC-1α occupancy in skeletal muscle cells from a previous study12, a 1.2 kilo base (kb) long region located ~7kb upstream of the BNP gene (on chromosome 4 from 147’352’500 to 147’353’700 base pairs (bp), mm9 genome assembly) was identified as highly enriched in PGC-1α IP reads. In order to account for the conservation of transcription factor binding sites (TFBSs) across related species, this 1.2
kb long region was aligned, using the software T-Coffee38, to its orthologous regions from 6 mammalian species: human (hg18), opossum (monDom4), dog (canFam2), rhesus macaque (rheMac2), horse (equCab1) and cow (bosTau3). The software MotEvo39 was used on these alignments to predict TFBSs for a collection of 190 mammalian regulatory motifs that was downloaded from the SwissRegulon database40.
Statistical analysis
Data were analyzed with either one-way ANOVA followed by Bonferroni post-hoc analyses or Student’s t test. P<
0.05 was considered statistically significant. Data are presented as mean
±
SEM.
Additional Information
How to cite this article: Furrer, R. et al. Paracrine cross-talk between skeletal muscle and macrophages in exercise by PGC-1α-controlled BNP. Sci. Rep. 7, 40789; 10.1038/srep40789 (2017).
Publisher's note: Springer Nature remains neutral with regard to jurisdictional claims in published maps and institutional affiliations.
Supplementary Material
Acknowledgments
We thank Dr. Silvia Salatino, Barbara Kupr and Dr. Mario Baresic for their help with the bioinformatics and the ChIPseq and knock-down experiments as well as Fabienne Battilana for the feedback on the manuscript. This project was funded by the Swiss National Science Foundation, the European Research Council (ERC) Consolidator grant 616830-MUSCLE_NET, the Swiss Society for Research on Muscle Diseases (SSEM), SystemsX.ch, the “Novartis Stiftung für Medizinisch-Biologische Forschung” and the University of Basel. This manuscript is dedicated to the memory of our coworker and friend Markus Beer (deceased on Aug 24, 2015).
Footnotes
Author Contributions Conceptualization: R.F., P.S.E. and C.H.; Methodology: R.F., P.S.E. and C.H.; Investigation: R.F., P.S.E., A.S. and M.B; Writing – Original Draft: R.F., P.S.E., C.H.; Funding Acquisition: C.H.; Supervision: C.H.
References
- Eisele P. S. & Handschin C. Functional crosstalk of PGC-1 coactivators and inflammation in skeletal muscle pathophysiology. Semin Immunopathol 36, 27–53, 10.1007/s00281-013-0406-4 (2014). [Abstract] [CrossRef] [Google Scholar]
- Pedersen B. K. & Saltin B. Exercise as medicine - evidence for prescribing exercise as therapy in 26 different chronic diseases. Scand J Med Sci Sports 25 Suppl 3, 1–72, 10.1111/sms.12581 (2015). [Abstract] [CrossRef] [Google Scholar]
- Handschin C. & Spiegelman B. M. The role of exercise and PGC1alpha in inflammation and chronic disease. Nature 454, 463–469, 10.1038/nature07206 (2008). [Europe PMC free article] [Abstract] [CrossRef] [Google Scholar]
- Booth F. W., Roberts C. K. & Laye M. J. Lack of exercise is a major cause of chronic diseases. Compr Physiol 2, 1143–1211, 10.1002/cphy.c110025 (2012). [Europe PMC free article] [Abstract] [CrossRef] [Google Scholar]
- Catoire M. & Kersten S. The search for exercise factors in humans. FASEB J 29, 1615–1628, 10.1096/fj.14-263699 (2015). [Abstract] [CrossRef] [Google Scholar]
- Pedersen B. K. & Febbraio M. A. Muscle as an endocrine organ: focus on muscle-derived interleukin-6. Physiol Rev 88, 1379–1406, 10.1152/physrev.90100.2007 (2008). [Abstract] [CrossRef] [Google Scholar]
- Schnyder S. & Handschin C. Skeletal muscle as an endocrine organ: PGC-1alpha, myokines and exercise. Bone 80, 115–125, 10.1016/j.bone.2015.02.008 (2015). [Europe PMC free article] [Abstract] [CrossRef] [Google Scholar]
- Kupr B. & Handschin C. Complex Coordination of Cell Plasticity by a PGC-1alpha-controlled Transcriptional Network in Skeletal Muscle. Front Physiol 6, 325, 10.3389/fphys.2015.00325 (2015). [Europe PMC free article] [Abstract] [CrossRef] [Google Scholar]
- Lin J. et al.. Transcriptional co-activator PGC-1 alpha drives the formation of slow-twitch muscle fibres. Nature 418, 797–801, 10.1038/nature00904 (2002). [Abstract] [CrossRef] [Google Scholar]
- Eisele P. S., Salatino S., Sobek J., Hottiger M. O. & Handschin C. The peroxisome proliferator-activated receptor gamma coactivator 1alpha/beta (PGC-1) coactivators repress the transcriptional activity of NF-kappaB in skeletal muscle cells. J Biol Chem 288, 2246–2260, 10.1074/jbc.M112.375253 (2013). [Europe PMC free article] [Abstract] [CrossRef] [Google Scholar]
- Eisele P. S., Furrer R., Beer M. & Handschin C. The PGC-1 coactivators promote an anti-inflammatory environment in skeletal muscle in vivo. Biochem Biophys Res Commun 464, 692–697, 10.1016/j.bbrc.2015.06.166 (2015). [Europe PMC free article] [Abstract] [CrossRef] [Google Scholar]
- Baresic M., Salatino S., Kupr B., van Nimwegen E. & Handschin C. Transcriptional network analysis in muscle reveals AP-1 as a partner of PGC-1alpha in the regulation of the hypoxic gene program. Mol Cell Biol, 10.1128/MCB.01710-13 (2014). [Europe PMC free article] [Abstract] [CrossRef] [Google Scholar]
- Salatino S., Kupr B., Baresic M., van Nimwegen E. & Handschin C. The Genomic Context and Corecruitment of SP1 Affect ERRalpha Coactivation by PGC-1alpha in Muscle Cells. Mol Endocrinol 30, 809–825, 10.1210/me.2016-1036 (2016). [Europe PMC free article] [Abstract] [CrossRef] [Google Scholar]
- Mootha V. K. et al.. Erralpha and Gabpa/b specify PGC-1alpha-dependent oxidative phosphorylation gene expression that is altered in diabetic muscle. Proc Natl Acad Sci USA 101, 6570–6575, 10.1073/pnas.0401401101 (2004). [Europe PMC free article] [Abstract] [CrossRef] [Google Scholar]
- Dinulovic I. et al.. Muscle PGC-1alpha modulates satellite cell number and proliferation by remodeling the stem cell niche. Skelet Muscle 6, 39, 10.1186/s13395-016-0111-9 (2016). [Europe PMC free article] [Abstract] [CrossRef] [Google Scholar]
- Dinulovic I. et al.. PGC-1alpha modulates necrosis, inflammatory response, and fibrotic tissue formation in injured skeletal muscle. Skelet Muscle 6, 38, 10.1186/s13395-016-0110-x (2016). [Europe PMC free article] [Abstract] [CrossRef] [Google Scholar]
- Rowe G. C. et al.. PGC-1alpha induces SPP1 to activate macrophages and orchestrate functional angiogenesis in skeletal muscle. Circ Res 115, 504–517, 10.1161/CIRCRESAHA.115.303829 (2014). [Europe PMC free article] [Abstract] [CrossRef] [Google Scholar]
- Goh J. & Ladiges W. C. Exercise enhances wound healing and prevents cancer progression during aging by targeting macrophage polarity. Mech Ageing Dev 139, 41–48, 10.1016/j.mad.2014.06.004 (2014). [Abstract] [CrossRef] [Google Scholar]
- Beiter T. et al.. Exercise, skeletal muscle and inflammation: ARE-binding proteins as key regulators in inflammatory and adaptive networks. Exerc Immunol Rev 21, 42–57 (2015). [Abstract] [Google Scholar]
- Hawley J. A., Hargreaves M., Joyner M. J. & Zierath J. R. Integrative biology of exercise. Cell 159, 738–749, 10.1016/j.cell.2014.10.029 (2014). [Abstract] [CrossRef] [Google Scholar]
- Nishikimi T., Maeda N. & Matsuoka H. The role of natriuretic peptides in cardioprotection. Cardiovasc Res 69, 318–328, 10.1016/j.cardiores.2005.10.001 (2006). [Abstract] [CrossRef] [Google Scholar]
- He Q., Wang D., Yang X. P., Carretero O. A. & LaPointe M. C. Inducible regulation of human brain natriuretic peptide promoter in transgenic mice. Am J Physiol Heart Circ Physiol 280, H368–376 (2001). [Abstract] [Google Scholar]
- Sudoh T., Kangawa K., Minamino N. & Matsuo H. A new natriuretic peptide in porcine brain. Nature 332, 78–81, 10.1038/332078a0 (1988). [Abstract] [CrossRef] [Google Scholar]
- Wang W., Ou Y. & Shi Y. AlbuBNP, a recombinant B-type natriuretic peptide and human serum albumin fusion hormone, as a long-term therapy of congestive heart failure. Pharm Res 21, 2105–2111 (2004). [Abstract] [Google Scholar]
- Engeli S. et al.. Natriuretic peptides enhance the oxidative capacity of human skeletal muscle. J Clin Invest 122, 4675–4679, 10.1172/JCI64526 (2012). [Europe PMC free article] [Abstract] [CrossRef] [Google Scholar]
- Bordicchia M. et al.. Cardiac natriuretic peptides act via p38 MAPK to induce the brown fat thermogenic program in mouse and human adipocytes. J Clin Invest 122, 1022–1036, 10.1172/JCI59701 (2012). [Europe PMC free article] [Abstract] [CrossRef] [Google Scholar]
- Miyashita K. et al.. Natriuretic peptides/cGMP/cGMP-dependent protein kinase cascades promote muscle mitochondrial biogenesis and prevent obesity. Diabetes 58, 2880–2892, 10.2337/db09-0393 (2009). [Europe PMC free article] [Abstract] [CrossRef] [Google Scholar]
- Sonoyama T. et al.. Inhibition of hepatic damage and liver fibrosis by brain natriuretic peptide. FEBS Lett 583, 2067–2070, 10.1016/j.febslet.2009.05.025 (2009). [Abstract] [CrossRef] [Google Scholar]
- Chiurchiu V. et al.. Brain Natriuretic Peptide (BNP) regulates the production of inflammatory mediators in human THP-1 macrophages. Regul Pept 148, 26–32, 10.1016/j.regpep.2008.02.009 (2008). [Abstract] [CrossRef] [Google Scholar]
- Sengenes C., Berlan M., De Glisezinski I., Lafontan M. & Galitzky J. Natriuretic peptides: a new lipolytic pathway in human adipocytes. FASEB journal: official publication of the Federation of American Societies for Experimental Biology 14, 1345–1351 (2000). [Abstract] [Google Scholar]
- Coue M. et al.. Defective natriuretic peptide receptor signaling in skeletal muscle links obesity to type 2 diabetes. Diabetes, 10.2337/db15-0305 (2015). [Abstract] [CrossRef] [Google Scholar]
- Das S. R. et al.. Impact of body mass and body composition on circulating levels of natriuretic peptides: results from the Dallas Heart Study. Circulation 112, 2163–2168, 10.1161/CIRCULATIONAHA.105.555573 (2005). [Abstract] [CrossRef] [Google Scholar]
- Wang T. J. et al.. Impact of obesity on plasma natriuretic peptide levels. Circulation 109, 594–600, 10.1161/01.CIR.0000112582.16683.EA (2004). [Abstract] [CrossRef] [Google Scholar]
- Huang W. S. et al.. Circulating brain natriuretic peptide values in healthy men before and after exercise. Metabolism 51, 1423–1426 (2002). [Abstract] [Google Scholar]
- Ohba H. et al.. Effects of prolonged strenuous exercise on plasma levels of atrial natriuretic peptide and brain natriuretic peptide in healthy men. Am Heart J 141, 751–758, 10.1067/mhj.2001.114371 (2001). [Abstract] [CrossRef] [Google Scholar]
- Subbotina E. et al.. Musclin is an activity-stimulated myokine that enhances physical endurance. Proc Natl Acad Sci USA, 10.1073/pnas.1514250112 (2015). [Europe PMC free article] [Abstract] [CrossRef] [Google Scholar]
- Glatter T. et al.. Large-scale quantitative assessment of different in-solution protein digestion protocols reveals superior cleavage efficiency of tandem Lys-C/trypsin proteolysis over trypsin digestion. J Proteome Res 11, 5145–5156, 10.1021/pr300273g (2012). [Abstract] [CrossRef] [Google Scholar]
- Notredame C., Higgins D. G. & Heringa J. T-Coffee: A novel method for fast and accurate multiple sequence alignment. J Mol Biol 302, 205–217, 10.1006/jmbi.2000.4042 (2000). [Abstract] [CrossRef] [Google Scholar]
- Arnold P., Erb I., Pachkov M., Molina N. & van Nimwegen E. MotEvo: integrated Bayesian probabilistic methods for inferring regulatory sites and motifs on multiple alignments of DNA sequences. Bioinformatics 28, 487–494, 10.1093/bioinformatics/btr695 (2012). [Abstract] [CrossRef] [Google Scholar]
- Pachkov M., Balwierz P. J., Arnold P., Ozonov E. & van Nimwegen E. SwissRegulon, a database of genome-wide annotations of regulatory sites: recent updates. Nucleic Acids Res 41, D214–220, 10.1093/nar/gks1145 (2013). [Europe PMC free article] [Abstract] [CrossRef] [Google Scholar]
Articles from Scientific Reports are provided here courtesy of Nature Publishing Group
Full text links
Read article at publisher's site: https://doi.org/10.1038/srep40789
Read article for free, from open access legal sources, via Unpaywall:
https://www.nature.com/articles/srep40789.pdf
Citations & impact
Impact metrics
Citations of article over time
Alternative metrics
Smart citations by scite.ai
Explore citation contexts and check if this article has been
supported or disputed.
https://scite.ai/reports/10.1038/srep40789
Article citations
Control of muscle satellite cell function by specific exercise-induced cytokines and their applications in muscle maintenance.
J Cachexia Sarcopenia Muscle, 15(2):466-476, 20 Feb 2024
Cited by: 2 articles | PMID: 38375571 | PMCID: PMC10995279
Review Free full text in Europe PMC
Potential mechanisms of exercise for relieving inflammatory pain: a literature review of animal studies.
Front Aging Neurosci, 16:1359455, 08 Feb 2024
Cited by: 1 article | PMID: 38389561 | PMCID: PMC10881774
Review Free full text in Europe PMC
Harmonizing Magnetic Mitohormetic Regenerative Strategies: Developmental Implications of a Calcium-Mitochondrial Axis Invoked by Magnetic Field Exposure.
Bioengineering (Basel), 10(10):1176, 10 Oct 2023
Cited by: 6 articles | PMID: 37892906 | PMCID: PMC10604793
Review Free full text in Europe PMC
Intravital microscopy of satellite cell dynamics and their interaction with myeloid cells during skeletal muscle regeneration.
Sci Adv, 9(42):eadi1891, 18 Oct 2023
Cited by: 0 articles | PMID: 37851799 | PMCID: PMC10584350
Plasma-derived extracellular vesicles released after endurance exercise exert cardioprotective activity through the activation of antioxidant pathways.
Redox Biol, 63:102737, 18 May 2023
Cited by: 10 articles | PMID: 37236143 | PMCID: PMC10220283
Go to all (20) article citations
Data
Data behind the article
This data has been text mined from the article, or deposited into data resources.
BioStudies: supplemental material and supporting data
Similar Articles
To arrive at the top five similar articles we use a word-weighted algorithm to compare words from the Title and Abstract of each citation.
PGC-1α induces SPP1 to activate macrophages and orchestrate functional angiogenesis in skeletal muscle.
Circ Res, 115(5):504-517, 09 Jul 2014
Cited by: 59 articles | PMID: 25009290 | PMCID: PMC4524357
Exercise induces TFEB expression and activity in skeletal muscle in a PGC-1α-dependent manner.
Am J Physiol Cell Physiol, 314(1):C62-C72, 18 Oct 2017
Cited by: 52 articles | PMID: 29046293 | PMCID: PMC5866381
Thyroid hormone activation by type 2 deiodinase mediates exercise-induced peroxisome proliferator-activated receptor-γ coactivator-1α expression in skeletal muscle.
J Physiol, 594(18):5255-5269, 18 Aug 2016
Cited by: 27 articles | PMID: 27302464
PGC-1alpha-mediated adaptations in skeletal muscle.
Pflugers Arch, 460(1):153-162, 19 Apr 2010
Cited by: 143 articles | PMID: 20401754
Review
Funding
Funders who supported this work.
European Research Council (1)
Coactivator-controlled transcriptional networks regulating skeletal muscle cell plasticity (MUSCLE-NET)
Prof Christoph Handschin, University of Basel
Grant ID: 616830