Abstract
Free full text

Mitotic checkpoint function in the formation of gross chromosomal rearrangements in Saccharomyces cerevisiae
Abstract
The accumulation of gross chromosomal rearrangements (GCRs) is characteristic of cancer cells. Multiple pathways that prevent GCRs, including S-phase cell cycle checkpoints, homologous recombination, telomere maintenance, suppression of de novo telomere addition, chromatin assembly, and mismatch repair, have been identified in Saccharomyces cerevisiae. However, pathways that promote the formation of GCRs are not as well understood. Of these, the de novo telomere addition pathway and nonhomologous end-joining are the best characterized. Here, we demonstrate that defects in the mitotic checkpoint and the mitotic exit network can suppress GCRs in strains containing defects that increase the GCR rate. These data suggest that functional mitotic checkpoints can play a role in the formation of genome rearrangements.
Genomic instability is a characteristic of cancer cells (1–4). Different types of genome rearrangements and mutations, including base substitution and frameshift mutations, translocations, deletions, inversions, and terminal deletion of chromosome arms, have been observed in many cancers. It has been suggested that cancer cells may acquire defects that destabilize the genome, drive cancer progression, and account for the high frequency of genetic changes in cancer cells (3, 5). Consistent with this, a number of inherited cancer-susceptibility syndromes associated with apparently increased gross chromosomal rearrangements (GCRs) have been identified (6–13). Examples are the ATM gene in ataxia telangiectasia, the NBS1 gene in Nijmegen breakage syndrome, the MRE11 gene in ataxia telangiectasia-like disorder, the CHK2 gene in Li–Fraumeni syndrome, the BLM and WRN genes in Bloom and Werner syndromes, and BRCA1 and BRCA2 in inherited breast cancer syndromes. Defects in these and other cancer-susceptibility genes have been linked to aberrant DNA repair and/or DNA damage responses.
At least seven different types of pathways play a role in suppression of GCRs in Saccharomyces cerevisiae (3): (i) the DNA damage and replication checkpoints that function during DNA replication (14–20), (ii) recombination pathways (21), (iii) a pathway that suppresses de novo telomere addition (21, 22), (iv) pathways that promote chromatin assembly during DNA replication (23), (v) DNA mismatch repair that prevents recombination between divergent DNA sequences (24, 25), (vi) pathways that prevent chromosome ends from being joined to each other and to broken DNAs (21, 26, 27), and (vii) pathways that prevent oxidative damage to DNA (28, 29).
Checkpoints act to ensure correct transmission of genetic information during cell division (30). There are a number of checkpoints that respond to DNA damage as well as to the formation of aberrant DNA structures that occurs when DNA replication is blocked (30, 31). The DNA damage checkpoint arrests the cell cycle in either G1 or G2 in response to DNA damage and slows DNA replication when DNA damage occurs during S phase (32–34); this latter checkpoint response is sometimes called the intra-S checkpoint. The DNA replication checkpoint arrests cell cycle progression and suppresses the firing of late replication origins in response to blocked DNA replication (35, 36). The mitotic checkpoints respond to the failure of spindle assembly and arrest the cell cycle at M phase (37, 38). Defects in various DNA damage and S-phase checkpoints also result in differing degrees of increased spontaneous GCR rates, increased chromosome loss, and increased recombination (14, 15, 39). However, compared with the significant increases in GCR rates caused by defects in S-phase checkpoints, defects in the mitotic checkpoint did not appear to increase GCR rates (14).
The mitotic checkpoint, also known as the spindle checkpoint, ensures proper chromosome segregation by arresting the cell cycle at mitosis by responding to improper or incomplete spindle assembly (37, 38). In S. cerevisiae (40–43), the genes that function in the mitotic checkpoint include MAD1, MAD2, MAD3, BUB1, BUB3, and MPS1, and these, in part, function through the anaphase-promoting complex. Bub2 functions in the mitotic exit network (MEN) by inhibiting degradation of mitotic cyclins and other regulators of exit from mitosis (44–46). Mitotic exit is achieved by inactivation of Tem1 by the Bub2 and Bfa1 GTPase-activating proteins (47, 48). Mutations in genes encoding mitotic-checkpoint and MEN proteins lead to increased missegregation of chromosomes, even in the absence of spindle damage (49) and failure of mitotic cell cycle arrest in response to spindle-depolymerizing drugs (42, 50). The mitotic checkpoints and the DNA damage and replication checkpoints also interact to arrest the cell cycle (51–53). In the present study, we have investigated interactions between mitotic checkpoint defects and different defects that cause increased genome instability.
Materials and Methods
General Genetic Methods. Methods for propagation of strains and determining GCR rates were as described in refs. 14 and 15. All S. cerevisiae strains were propagated at 30°C, except those carrying the rfc5-1 mutation, which were propagated at 23°C. All strains were made by standard PCR-based gene disruption methods, and correct gene disruptions were verified by PCR as described in refs. 14 and 54. The sequences of primers used to generate disruption cassettes and confirm disruptions are available upon request. All strains were derived from the S288c parental strain RDKY3615 (MATa, ura3-52, leu2Δ1, trp1Δ63, his3Δ200, lys2ΔBgl, hom3-10, ade2Δ1, ade8, and hxt13::URA3). Relevant genotypes of these strains are as follows: RDKY3615 wild type; RDKY3727 rfc5-1; RDKY3735 sml1::KAN mec1::HIS3; RDKY3739 dun1::HIS3; RDKY3717 bub3::TRP1; RDKY4893 rfc5-1 bub3::HIS3; RDKY 4895 sml1::KAN mec1::HIS3 bub3::TRP1; RDKY4897 dun1::HIS3, bub3::TRP1; RDKY4584 mad2::HIS3; RDKY4944 rfc5-1 mad2::HIS3; RDKY4946 sm1l::KAN mec1::HIS3 mad2::TRP1; RDKY4948 dun1::HIS3 mad2::TRP1; RDKY3715 mad3::TRP1; RDKY4928 rfc5-1 mad3::HIS3; RDKY4930 sml1::KAN mec1::HIS3 mad3::TRP1; RDKY4932 dun1::HIS3 mad3::TRP1; RDKY3633 mre11::HIS3; RDKY3617 rfa1-t33; RDKY3630 rad27::KAN; RDKY4421 rad52::HIS3; RDKY4343 pif1-m2; RDKY4899 bub3::TRP1 mre11::HIS3; RDKY4901 rfa1-t33 bub3::TRP1; RDKY4903 rad27::KAN bub3::TRP1; RDKY4924 bub3::TRP1 rad52::HIS3; RDKY4926 pif1-m2 bub3::TRP1; RDKY4950 mad2::HIS3 mre11::TRP1; RDKY4952 rfa1-t33 mad2::TRP1; RDKY4954 rad27::KAN mad2::HIS3; RDKY4956 mad2::HIS3 rad52::TRP1; RDKY4958 pif1-m2 mad2::HIS3; RDKY4934 mad3::TRP1 mre11::HIS3; RDKY4936 rfa1-t33 mad3::HIS3; RDKY4938 rad27::KAN mad3::TRP1; RDKY4940 mad3::TRP1 rad52::HIS3; RDKY4942 pif1-m2 mad3::TRP1; RDKY4960 bub2::TRP1; RDKY4962 rfc5-1 bub2::HIS3; RDKY4964 sml1::KAN mec1::HIS3 bub2::TRP1; RDKY4966 dun1::HIS3 bub2::TRP1; RDKY4968 bub2::TRP1 mre11::HIS3; RDKY4970 rfa1-t33 bub2::HIS3; RDKY4972 rad27::KAN bub2::TRP1; RDKY4974 bub2::TRP1 rad52::HIS3; RDKY4976 pif1-m2 bub2::TRP1; RDKY4980 mad2::HIS3 bub2::TRP1; RDKY 4982 mad2::HIS3 bub2::TRP1 mre11::KAN; RDKY5155 bub1::TRP1; RDKY5156 sml1::KAN mec1::HIS3 bub1::HYG; RDKY5157 dun1::HIS3 bub1::HYG; RDKY5161 bub1::TRP1 mre11::HIS3; RDKY5162 rfa1-t33 bub1::HYG; RDKY5163 bub1::TRP1 rad27::HIS3; RDKY5164 bub1::TRP1 rad52::HIS3; RDKY5165 pif1-m2 bub1::TRP1; RDKY5172 ctf8::HIS3; RDKY5173 ctf18::HIS3; RDKY5174 dcc1::HIS3; RDKY5175 pif1-m2 ctf8::HIS3; RDKY5176 pif1-m2 ctf18::HIS3; RDKY5177 pif1-m2 dcc1::HIS3; RDKY5178 sml1::KAN mec1::TRP1 ctf8::HIS3; RDKY5179 sml1::KAN mec1::TRP1 ctf18::HIS3; and RDKY5180 sml1::KAN mec1::TRP1 dcc1::HIS3.
DNA-Damaging Agents and Temperature Sensitivity. Strains of interest were cultured at 30°C until saturation. Then, cells were diluted 1:50 and incubated at 30°C for4hwith shaking. Cells were serially diluted (1:10), spotted onto plates containing DNA-damaging agents, and incubated at 30°C for 3 days or at 37°C for 2 days. For the UV irradiation sensitivity experiment, after spotting cells on yeast extract/peptone/dextrose (YPD) plates, the plates were UV-irradiated and incubated at 30°C for 3 days in complete darkness. The induction of GCR frequency after treatment with methyl methanesulfonate (MMS) was determined as described in ref. 15.
Characterization of GCR Rates and Breakpoints. All GCR rates were determined independently by fluctuation analysis two or more times by using either 5 or 11 cultures, and the average value was reported. The sequences of independent rearrangement break-points were determined and classified as described in refs. 14, 28, and 54. Statistical tests were performed by using the Mann–Whitney test and programs available at http://faculty.vassar.edu/lowry/vshome.html.
Results
Mutations in Mitotic Checkpoint Genes Reduce the Increased GCR Rates Caused by S-Phase Checkpoint Defects. To investigate genetic interactions between defects in the S-phase checkpoints and the mitotic checkpoints, the rfc5-1 mutation that causes defects in the DNA replication checkpoint (55), a mec1 mutation that eliminates the central checkpoint kinase, and a dun1Δ mutation that eliminates a factor that acts downstream of Mec1 (56–58) were combined with different mutations in mitotic checkpoint genes, and the GCR rates of the resulting strains were determined. These three mutations were tested because they each result in a 100- to 200-fold increase in the spontaneous GCR rate and are in the same pathway with regard to suppression of spontaneous GCRs (14). Surprisingly, the GCR rates of the rfc5-1 bub3, mec1 bub3, or dun1 bub3 double mutants were reduced to the level of the bub3 single mutant and the wild-type control strain (Tables (Tables11 and and2).2). This reduction suggests that the BUB3 gene product is required for the GCRs that occur in strains defective for S-phase checkpoints. A bub1 mutation partially reduced the GCR rates caused by the mec1 and dun1 mutations (rfc5-1 was not tested), although possibly not to the same extent as that seen for a bub3 mutation. Mutations in other mitotic checkpoint genes showed different patterns of genetic interaction with the rfc5-1, mec1, and dun1 mutations. Mutations in either MAD2 or MAD3 genes did not reduce the GCR rate caused by a mec1 mutation. In contrast, the GCR rate of the dun1 mad3 double mutant but not the dun1 mad2 double mutant was reduced compared with the dun1 single mutant, with essentially complete suppression observed for the dun1 mad3 double mutant. The interactions with rfc5-1 were different with a mad2 mutation, which reduced the GCR rate to close to wild-type levels; a mad3 mutation had no effect. To investigate whether there is redundancy between MAD2 and MAD3, both the MAD2 and MAD3 genes were mutated in a mec1 strain, and the GCR rate was determined (Tables (Tables11 and and2).2). The GCR rate of the mad2 mad3 mec1 triple-mutant strain was reduced compared with the mec1 strain to a level similar to that of the wild-type strain. In no case was a reduction in plating efficiency of a double-mutant strain observed relative to the respective single mutants.
Table 1.
Wild type
| bub1Δ
| bub3Δ
| mad2Δ
| mad3Δ
| bub2Δ
| |||||||
---|---|---|---|---|---|---|---|---|---|---|---|---|
Relevant genotype | Strain no. | Mutation rate (Canr 5-FOAr) | Strain no. | Mutation rate (Canr 5-FOAr) | Strain no. | Mutation rate (Canr 5-FOAr) | Strain no. | Mutation rate (Canr 5-FOAr) | Strain no. | Mutation rate (Canr 5-FOAr) | Strain no. | Mutation rate (Canr 5-FOAr) |
Wild type | 3615 | 3.5 × 10-10 (1) | 5155 | 4.7 × 10-10 (2) | 3717 | 3.9 × 10-10 (1) | 4584 | 5.6 × 10-10 (2) | 3715 | 2.4 × 10-10 (1) | 4960 | 3.4 × 10-10 (1) |
rfc5-1 | 3727 | 5.5 × 10-8 (157) | ND | 4893 | 2.2 × 10-10 (1) | 4944 | 3.9 × 10-9 (11) | 4928 | 2.4 × 10-8 (69) | 4962 | 1.3 × 10-9 (4) | |
mec1Δ* | 3735 | 4.6 × 10-8 (131) | 5156 | 9.6 × 10-9 (27) | 4895 | 2.7 × 10-10 (1) | 4946 | 4.2 × 10-8 (120) | 4930 | 2.0 × 10-8 (57) | 4964 | 3.0 × 10-8 (86) |
dun1Δ | 3739 | 3.4 × 10-8 (97) | 5157 | 4.2 × 10-9 (12) | 4897 | 3.3 × 10-10 (1) | 4948 | 1.2 × 10-8 (34) | 4932 | 2.8 × 10-10 (1) | 4966 | 3.8 × 10-8 (109) |
mre11Δ | 3633 | 2.2 × 10-7 (657) | 5161 | 1.9 × 10-8 (54) | 4899 | 2.6 × 10-8 (74) | 4950 | 4.8 × 10-8 (137) | 4934 | 6.5 × 10-8 (186) | 4968 | 2.9 × 10-9 (8) |
rfa1-t33 | 3617 | 2.7 × 10-7 (771) | 5162 | 1.2 × 10-7 (342) | 4901 | 5.8 × 10-8 (166) | 4952 | 2.3 × 10-7 (657) | 4936 | 4.7 × 10-8 (134) | 4970 | 3.9 × 10-8 (111) |
rad27Δ | 3630 | 2.4 × 10-7 (686) | 5163 | 2.6 × 10-7 (743) | 4903 | 1.7 × 10-7 (486) | 4954 | 2.0 × 10-7 (571) | 4938 | 1.4 × 10-7 (400) | 4972 | 2.4 × 10-7 (686) |
rad52Δ | 4421 | 3.5 × 10-8 (100) | 5164 | 4.2 × 10-8 (120) | 4924 | 3.0 × 10-8 (86) | 4956 | 2.6 × 10-8 (74) | 4940 | 3.2 × 10-8 (91) | 4974 | 8.9 × 10-9 (25) |
pif1-m2 | 4343 | 4.8 × 10-8 (137) | 5165 | 6.8 × 10-8 (194) | 4926 | 9.5 × 10-9 (27) | 4958 | 7.8 × 10-9 (22) | 4942 | 6.3 × 10-9 (18) | 4976 | 1.2 × 10-9 (3) |
The numbers in parentheses indicate the GCR rate relative to the wild-type GCR rate. The GCR rate of the mad2Δ bub2Δ (RDKY4980), mec1Δ sml1Δ mad2Δ mad3Δ (RDKY4978), and mad2Δ bub2Δ mre11Δ (RDKY4982) strains were 3.3 × 10-10 (1), 6.9 × 10-10 (2), and 3.3 × 10-8 (94), respectively. ND, not determined; Canr, canavanine-resistant; 5-FOAr, 5-fluoroorotic acid-resistant.
Table 2.
bub1Δ | bub3Δ | mad2Δ | mad3Δ | bub2Δ | |
---|---|---|---|---|---|
rfc5-1 | ND | 0.6 (<0.01) | 7.0 (0.01) | 43.9 (0.13) | 2.6 (<0.01) |
mec1Δ* | 20.6 (0.03) | 0.8 (<0.01) | 91.6 (0.35) | 43.5 (0.31) | 65.7 (0.94) |
dun1Δ | 12.4 (0.02) | 1.0 (<0.01) | 35.1 (0.21) | 1.0 (<0.01) | 122.4 (0.57) |
mre11Δ | 8.2 (<0.01) | 11.3 (<0.01) | 20.9 (<0.01) | 28.3 (<0.01) | 1.2 (<0.01) |
rfa1-t33 | 44.4 (<0.01) | 21.5 (<0.01) | 85.2 (0.70) | 17.4 (<0.01) | 14.4 (<0.01) |
rad27Δ | 108.3 (0.33) | 70.9 (0.52) | 83.2 (0.24) | 58.3 (0.19) | 100.0 (0.43) |
rad52Δ | 120.0 (0.26) | 86.0 (0.35) | 74.0 (0.73) | 91.0 (0.97) | 25.0 (0.94) |
pif1-m2 | 141.6 (0.85) | 19.7 (0.01) | 16.1 (<0.01) | 13.1 (0.01) | 2.2 (<0.01) |
The number given is the fold increase of the GCR rate of the double mutant indicated by the intersection of the mutations listed along the top row and the left column relative to wild type expressed as a percentage of the fold increase of the GCR rate of the single mutant indicated along the left column relative to wild type (calculated from the data of Table 1) and is a measure of the extent of suppression observed. Numbers in parentheses are the P values (Mann—Whitney test) calculated for the significance of GCR rate reduction by the addition of each mitotic checkpoint gene mutation. ND, not determined.
These results suggest a complex set of interactions between mutations in mitotic checkpoint genes and mutations that affect DNA damage and replication checkpoints. This possibility was confirmed by performing a limited analysis of the breakpoint structure of the GCRs that occur in several of the double-mutant strains. The GCRs generated in the dun1 mad2 strain were all de novo telomere additions, and, hence, this strain had the same GCR spectrum as the dun1 single-mutant strain (Table 3). Thus, the mad2 mutation neither reduces the rate of the GCRs nor changes the spectrum of GCRs formed as a result of a dun1 mutation. In contrast, virtually all rearrangements from the rfc5-1 mad3 strain were microhomology-mediated translocations, compared with all de novo telomere additions in the rfc5-1 single mutant (Table 3). Thus, even though a mutation in the MAD3 gene did not alter the GCR rate caused by the rfc5-1 mutation, it nonetheless altered the mechanism by which the genome rearrangement occurred.
Table 3.
Relevant genotype | Strain no. | Telomere addition | Translocation* |
---|---|---|---|
Wild type† | 3615 | 5 (2.9 × 10-10) | 1, 0 (6.0 × 10-11) |
rfc5-1† | 3727 | 10 (5.5 × 10-8) | 0, 0 (<5.5 × 10-9) |
rfc5-1 mad3Δ | 4928 | 1 (2.0 × 10-9) | 0, 9‡ (2.2 × 10-8) |
rfa1-t33§ | 3617 | 5 (1.2 × 10-7) | 0, 6¶ (1.5 × 10-7) |
rfa1-t33 mad2Δ | 4952 | 16 (1.9 × 10-7) | 0, 3 (4.0 × 10-8) |
rfa1-t33 mad3Δ | 4936 | 11 (3.7 × 10-8) | 0, 3 (1.0 × 10-8) |
rfa1-t33 bub2Δ | 4970 | 13 (3.4 × 10-8) | 0, 2![]() |
rfa1-t33 bub3Δ | 4901 | 11 (4.6 × 10-8) | 0, 3 (1.2 × 10-8) |
dun1Δ† | 3739 | 10 (3.4 × 10-8) | 0, 0 (<3.4 × 10-9) |
dun1Δ mad2Δ | 4948 | 12 (1.2 × 10-8) | 0, 0 (<1.0 × 10-9) |
dun1Δ bub2Δ | 4964 | 10 (3.8 × 10-8) | 0, 0 (<3.8 × 10-9) |
The numbers reported are the actual numbers of each type of event observed.

Mutations in Mitotic Checkpoint Genes Reduce GCR Rates Induced by a Broad Spectrum of Genetic Defects. Previous studies identified a number of pathways besides the cell cycle checkpoints in which defects cause increased GCR rates (21, 54). We next studied the interaction between mitotic checkpoint defects and a selection of mutations that inactivate other genes and pathways that suppress GCRs. The mutations tested included the following: (i) an mre11Δ mutation that inactivates the Mre11–Rad50–Xrs2 complex, which functions in cycle-phase checkpoints, homologous recombination repair, telomere maintenance, and nonhomologous end-joining (59, 60); (ii) the rfa1-t33 mutation that causes a defect in replication factor A, a heterotrimeric single-stranded DNA (ssDNA)-binding protein required for various aspects of DNA metabolism, including DNA replication, recombination, repair, and checkpoint responses (54, 61, 62); (iii) a defect in the RAD27 gene, which encodes an endo/exonuclease required for Okazaki fragment processing (63, 64); (iv) a defect in the RAD52 gene, which functions in homologous recombination, including break-induced replication, single-strand annealing, and gene conversion (60); and (v) a defect in the PIF1 gene, which encodes an inhibitor of telomerase that functions in suppressing the de novo telomere addition-type of GCRs (21, 65).
Mutations in BUB1, BUB3, MAD2, and MAD3 caused a partial reduction in the increased GCR rates caused by the mre11 mutation (Tables (Tables11 and and2).2). Partial suppression of the GCR rate was also observed with the rfa1-t33 mutation, and subsequent analysis of the GCRs formed indicated that the mitotic checkpoint defects in the rfa1-t33 mutant reduced the rate of formation of translocations 4- to 15-fold and reduced the rate of telomere additions up to 3-fold (Table 3). Similar partial suppression was observed with the pif1-m2 mutation, with the exception that a bub1 mutation did not appear to affect the increased GCR rate caused by the pif1-m2 mutation (Tables (Tables11 and and2).2). In contrast, the increased GCR rates in rad27 and rad52 mutants were not reduced by mutations in BUB1, BUB3, MAD2, and MAD3. The reasons for the difference in behavior of the rad27 and rad52 mutations vs. the mre11, rfa1-t33, and pif1-m2 mutations are unclear. However, it should be noted that the rad27 and rad52 mutations might be expected to increase the steady-state levels of broken chromosomes because of increased replication errors and decreased recombination, whereas the mre11, rfa1-t33, and pif1-m2 mutations might be expected to alter the cellular responses to normal levels of broken chromosomes by causing checkpoint defects or defects in suppression of de novo telomere addition.
Defects in the MEN Interact with Genetic Defects, Causing Increased GCR Rates. At the end of mitosis, the MEN functions in S. cerevisiae to ensure correct chromosome segregation (37). Bub2 and Bfa1, which are GTPase-activating proteins, function in the MEN. To determine whether the MEN plays a role in the formation of GCRs, the effect of a mutation in the BUB2 gene on the increased GCR rates caused by a variety of genetic defects was investigated. A bub2-null mutation did not affect the GCR rate caused by null mutations in MEC1 or DUN1, nor did it alter the GCR spectrum caused by loss of Dun1 (Tables (Tables1,1, ,2,2, and and3).3). However, the bub2 mutation did reduce the GCR rate caused by the rfc5-1 mutation. The variable effects of the bub2 mutation, when combined with the mec1, dun1, and rfc5-1 mutations, may be related to the fact that Dun1 and Mec1 may act in the MEN (Tables (Tables11 and and2)2) (51). The bub2 mutation did not reduce the GCR rate caused by the rad27 and rad52 mutations, similar to all of the other mitotic checkpoint defects tested. The bub2 mutation reduced the GCR rates caused by the mre11 and pif1-m2 mutations to essentially wild-type levels and partially reduced the increased GCR rate caused by the rfa1-t33 mutation (Tables (Tables11 and and2).2). As observed with the other mitotic checkpoint defects, the bub2 mutation reduced the translocation class of GCRs caused by the rfa1-t33 mutation to a greater extent than that seen for telomere additions (Table 3). These results suggest that, like the mitotic checkpoint defects, MEN defects can also modify the GCR rates and spectra caused by defects that increase the accumulation of spontaneous GCRs.
Defects in Establishing Sister Chromatid Cohesion Suppress Genome Instability. The establishment of sister chromatid cohesion and microtubule tension are sensed by the mitotic checkpoints. We therefore tested whether defects in these processes showed genetic interactions with mutations that cause increased GCR rates. The CTF8, CTF18, and DCC1 gene products are part of the cohesion complex required to establish sister chromatid cohesion (66). Mutations in each of these three genes alone did not change the GCR rate, but they each partially reduced the GCR rates caused by the mec1 and pif1-m2 mutations (Table 4); the effects on the mec1 mutant were of borderline significance (Table 5). These results suggest that defects in establishment of sister chromatid cohesion can also suppress the increased GCR rates caused by different mutator mutations.
Table 4.
Wild type
| pif1-m2
| mec1Δ*
| ||||
---|---|---|---|---|---|---|
Relevant genotype | Strain no. | Mutation rate (Canr 5-FOAr) | Strain no. | Mutation rate (Canr 5-FOAr) | Strain no. | Mutation rate (Canr 5-FOAr) |
Wild type | 3615 | 3.5 × 10-10 (1) | 4343 | 4.8 × 10-8 (137) | 3735 | 4.6 × 10-8 (131) |
ctf8Δ | 5172 | 3.5 × 10-10 (1) | 5175 | 6.2 × 10-9 (18) | 5178 | 1.3 × 10-8 (36) |
ctf18Δ | 5173 | 3.2 × 10-10 (1) | 5176 | 5.3 × 10-9 (15) | 5179 | 1.4 × 10-8 (40) |
dcc1Δ | 5174 | 4.1 × 10-10 (1) | 5177 | 3.3 × 10-9 (9) | 5180 | 1.2 × 10-8 (34) |
Numbers in parentheses indicate the GCR rate relative to the wild-type GCR rate.
Table 5.
ctf8Δ | ctf18Δ | dcc1Δ | |
---|---|---|---|
mec1Δ* | 27.5 (0.05) | 30.5 (0.19) | 26.0 (0.05) |
pif1-m2 | 13.1 (<0.01) | 11.0 (0.01) | 6.6 (0.01) |
The number given is the fold increase of the GCR rate of the double mutant indicated by the intersection of the mutations listed along the top row and the left column expressed relative to wild type as a percentage of the fold increase of the GCR rate of the single mutant indicated along the left column relative to wild type (calculated from the data of Table 4) and is a measure of the extent of suppression observed. Numbers in parentheses are the P values (Mann-Whitney test) calculated for the significance of GCR rate reduction by the addition of each cohesion gene mutation.
Mutator Mutants with Mutations in Different Mitotic Checkpoint Genes Display Enhanced Sensitivity to DNA-Damaging Agents. The formation of GCRs represents a form of DNA repair, albeit misrepair. One hypothesis for the observed suppression of GCR rates by mitotic checkpoint defects is that when the mitotic checkpoints are functional, this allows for misrepair to occur. In the absence of functional mitotic checkpoints, the cells continue to cycle with damaged DNAs, and this leads to death. To gain further insight into this idea, mre11 bub2, mre11 bub3, rad52 bub2, and rad52 bub3 double mutants were tested for sensitivity to increased temperature (a bub3 mutation causes temperature sensitivity) and treatment with MMS and UV radiation (Fig. 1). Compared with the single mutants, the mre11 bub3 double mutant showed enhanced temperature sensitivity, and both the mre11 bub2 and mre11 bub3 double mutants showed enhanced sensitivity to MMS and UV irradiation. Compared with the single mutants, the rad52 bub3 double mutant showed enhanced temperature sensitivity, and both the rad52 bub2 and rad52 bub3 double mutants showed enhanced sensitivity to MMS and UV irradiation. These results indicate that, at least in the case of the rad52 and mre11 mutations that by themselves cause sensitivity to DNA-damaging agents, this sensitivity is enhanced by mitotic checkpoint and MEN defects.
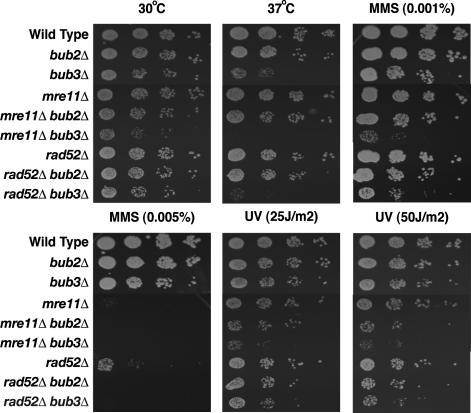
Enhanced sensitivity to temperature and DNA-damaging agents caused by defects in the mitotic checkpoint and the MEN. Strains with the indicated genotypes were tested for sensitivity to temperature, MMS, or UV irradiation as described in Materials and Methods. Each row from left to right shows 1:10 serial dilutions.
We previously showed that treatment of a wild-type strain with MMS increased the frequency of GCRs (15). Consequently, we tested the effect of mutations in mitotic checkpoint genes on MMS-induced GCRs. Similar to their effect on the increased GCR rates caused by the rfc5-1, mec1, and dun1 mutations, the bub1 and bub3 mutations significantly reduced the inductions of GCRs by MMS treatment (data not shown). Mutations in BUB2 and MAD2 caused only a small reduction in MMS-induced GCRs, and a mad3 mutation did not appear to have any effect on MMS-induced GCRs.
Discussion
In previous studies, we demonstrated that defects in at least three different S-phase checkpoints (the DNA replication checkpoint and two intra-S checkpoints) resulted in increased GCR rates, whereas defects in the mitotic checkpoint and the MEN did not (14, 15). Here, we investigated genetic interactions between a series of mutations that cause defects in different pathways that suppress the accumulation of GCRs and mutations that cause defects in either the mitotic checkpoint or the MEN. The data presented here indicate that, in many cases, defects in the mitotic checkpoint and the MEN either partially or completely suppressed the accumulation of GCRs, and, consistent with this, defects in the establishment of sister chromatid cohesion also partially suppressed the formation of GCRs. In addition, mitotic checkpoint and MEN defects enhanced the DNA-damaging agent sensitivity caused by the mre11 and rad52 mutations. Collectively, these results support the hypothesis (Fig. 2) that in some genetic backgrounds, the damaged DNA molecules that result in GCRs activate the mitotic checkpoint and the MEN, resulting in cell cycle delay, during which repair and misrepair to form GCRs occur. In the absence of a functional mitotic checkpoint and MEN, the cells continue to cycle in the presence of damaged DNAs, resulting in increased lethality and, hence, apparent suppression of GCRs.
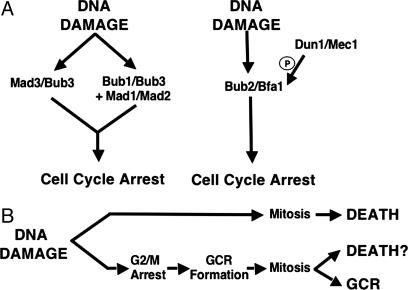
Model for the relationship between the mitotic checkpoint and MEN genes and the formation of GCRs. (A) Models illustrate genetic interactions among checkpoint genes. P, phosphorylation. (B) Model for the interaction between checkpoint activation and either the formation of GCRs or lethality.
The extent of suppression of GCRs by mitotic checkpoint defects varied, depending on the GCR mutator mutant analyzed (Tables (Tables11 and and2).2). The increased GCR rates caused by the rfc5-1, mec1, and dun1 mutations, whose affects have been previously ascribed to defects in the replication checkpoint (14), were completely suppressed by a bub3 mutation that completely blocks the mitotic checkpoint. In the case of the most completely analyzed mutation, mec1, partial suppression was seen with the bub1, mad2, and mad3 mutations, and the mad2 mad3 double-mutation combination caused complete suppression; these results are consistent with the view that both of the proposed subbranches of the mitotic checkpoint are important for the formation of GCRs in a mec1 mutant (Fig. 2). Analysis of the rfc5-1 and dun1 mutations yielded similar results, with the exception that for dun1 the MAD3 subbranch may be more important than the MAD2 subbranch. This pattern of suppression suggests that because damaged DNAs cannot cause cell cycle arrest because of the replication checkpoint defects, some sort of activation of the mitotic checkpoint by the damaged DNAs occurs or that mitotic checkpoint components also function during interphase. Consistent with the former idea, it is known that telomerase activity increases at the G2/M-phase boundary (67), correlating with the preponderance of de novo telomere addition-type GCRs in replication checkpoint mutants (21). In contrast to replication checkpoint defects, mitotic checkpoint defects did not cause any suppression of the increased GCR rate caused by the rad27 and rad52 mutations (Tables (Tables11 and and2).2). Mutations in RAD27 cause defects in Okazaki fragment processing, resulting in increased levels of double-strand breaks (DSBs), whereas defects in RAD52 cause defects in DSB repair that might result in higher levels of spontaneous DSBs (60, 63). In both cases, the DNA damage checkpoints are intact and would result in cell cycle arrest or delay at G2/M, and hence the mitotic checkpoint might not be as important. Finally, mitotic checkpoint defects caused partial suppression of the increased GCR rate caused by the rfa1-t33, pif1-m2, and mre11 mutations. These mutations cause replication defects (rfa1-t33); increased de novo telomere addition (pif1-m2); a defect in recombination, nonhomologous end-joining, and telomere maintenance; and a defect in S-phase checkpoint responses seen primarily when Mec1 is inactivated (mre11) (15, 21, 22, 60, 61). In these cases, it is possible that the damaged DNAs that arise in these mutant strains either do not fully activate the mitotic checkpoint because other checkpoints are intact or possibly do not cause as much lethality in the absence of checkpoint-dependent repair.
Like mitotic checkpoint defects, MEN defects also resulted in the suppression of GCRs in different mutant backgrounds (Tables (Tables11 and and2).2). There were both similarities and differences in mitotic checkpoint and MEN defects. The MEN defect tested, bub2, completely suppressed the rfc5-1 mutant but not the mec1 or dun1 mutations. However, it should be noted that the activity of the MEN is regulated by phosphorylation by Dun1, which is in turn regulated by Mec1 (Fig. 2) (51). Thus, it is possible that the mec1 and dun1 mutants are already at least partially deregulated for the MEN, and, hence, bub2 mutations cause less suppression in these mutants compared with the rfc5-1 mutant. Like mitotic checkpoint defects, MEN defects caused no suppression of rad52 or rad27 (Tables (Tables11 and and2).2). Surprisingly, although MEN defects caused about the same level of suppression of the rfa1-t33 mutation as did the mitotic checkpoint defects, MEN defects caused almost complete suppression of the mre11 and pif1-m2 mutations, compared with less suppression by mitotic checkpoint defects (Tables (Tables11 and and2).2). These results suggest that the MEN is more sensitive to the types of damaged DNAs that arise in the mre11 and pif1-m2 mutants than is the mitotic checkpoint but seem to be inconsistent with the idea that the damaged DNAs that arise in these mutants cause only limited lethality in the absence of the checkpoints. Overall, these results support the view that the MEN, like the mitotic checkpoint, can be activated by damaged DNAs and alter their repair.
The mitotic checkpoint and MEN function to maintain cell cycle delay until the spindle has properly assembled. In S. cerevisiae, the mitotic checkpoint and MEN are not essential functions, but in their absence there is significantly increased missegregation of chromosomes during mitosis (49). It is possible that chromosome missegregation caused by mutations in mitotic checkpoint and MEN genes might contribute to the reduced GCR rate seen in many of the double mutants examined. We previously suggested that spontaneous GCRs arise as the result of errors during DNA replication (3). Thus, when cells containing a rearranged chromosome reach mitosis, it is possible that the increased chromosome missegregation caused by mitotic checkpoint and MEN gene mutations could result in missegregation of the rearranged chromosome V with an intact copy of chromosome V. As a result, cell division would produce two cells: one containing two chromosome Vs (one that is intact and one that is the GCR chromosome, which would die because of selection in the GCR assay) and a second lacking a chromosome V, which would also be lethal. It is also worth noting that some mutations that result in increased GCR rates, including checkpoint and recombination defects, also increase chromosome missegregation (39), and this increase might contribute to lethality because of chromosome missegregation due to mitotic checkpoint and MEN defects. Whether such effects could account for the complete suppression of GCRs in some cases and the partial suppression in others is unclear. Thus, we tend to favor the view that activation of the mitotic checkpoint and MEN directly or a function of the mitotic checkpoint and MEN genes in interphase contributes to the formation of GCRs, rather than increased chromosome segregation defects being totally responsible for the suppression of GCRs.
The accumulation of multiple mutations and genome rearrangements is a hallmark of cancer cells (3, 5). Considerable evidence suggests that the formation of GCRs is an early step in carcinogenesis (2–4). It has been suggested that mitotic checkpoint defects might underlie chromosome loss and accumulation of chromosomal aberrations in cancer cells (37). Rare mutations in mitotic checkpoint genes and reduced expression of mitotic checkpoint proteins have been found in some cancers, and deletion of one allele of several mitotic checkpoint genes in mice leads to increased spontaneous and carcinogen-induced cancers (68–74). However, other studies have shown that loss-of-function mitotic checkpoint defects can cause both cell-lethal and embryonic-lethal phenotypes (75–84). Interestingly, it has been observed that a mitotic checkpoint defect both cooperates with a Brca2 defect in the development of tumors in mutant mice and reduces the level of chromosome aberrations observed (85). Along with these observations, our data support the idea that mitotic checkpoint defects could play two different roles in carcinogenesis: the induction of some types of chromosome instability and the partial suppression of the deleterious affects of mutations that result in increased genome instability.
Acknowledgments
We thank S. Banerjee, D. Cleveland, M.-E. Huang, J.-Y. Hwang, A. Motegi, V. Pennaneach, and K. Schmidt for helpful discussions and comments on the manuscript, and J. Weger, D. Cassel, and M. Tresierras for DNA sequencing. This work was supported by National Institutes of Health Grant GM26017 (to R.D.K.), a fellowship from the Cancer Research Fund of the Damon Runyon–Walter Winchell Foundation (to K.M.), and a National Human Genome Research Institute intramural research grant (to K.M.).
Notes
Abbreviations: Canr, canavanine-resistant; 5-FOAr, 5-fluoroorotic acid-resistant; GCR, gross chromosomal rearrangement; MEN, mitotic exit network; MMS, methyl methanesulfonate.
References
Articles from Proceedings of the National Academy of Sciences of the United States of America are provided here courtesy of National Academy of Sciences
Full text links
Read article at publisher's site: https://doi.org/10.1073/pnas.0407010101
Read article for free, from open access legal sources, via Unpaywall:
https://europepmc.org/articles/pmc528767?pdf=render
Citations & impact
Impact metrics
Citations of article over time
Article citations
NEK2 plays an essential role in porcine embryonic development by maintaining mitotic division and DNA damage response via the Wnt/β-catenin signalling pathway.
Cell Prolif, 57(8):e13626, 01 Mar 2024
Cited by: 2 articles | PMID: 38426218 | PMCID: PMC11294417
Signification and Application of Mutator and Antimutator Phenotype-Induced Genetic Variations in Evolutionary Adaptation and Cancer Therapeutics.
J Microbiol, 61(12):1013-1024, 15 Dec 2023
Cited by: 0 articles | PMID: 38100001
Review
DNA polymerase ε relies on a unique domain for efficient replisome assembly and strand synthesis.
Nat Commun, 11(1):2437, 15 May 2020
Cited by: 14 articles | PMID: 32415104 | PMCID: PMC7228970
Esc2 orchestrates substrate-specific sumoylation by acting as a SUMO E2 cofactor in genome maintenance.
Genes Dev, 35(3-4):261-272, 14 Jan 2021
Cited by: 7 articles | PMID: 33446573 | PMCID: PMC7849368
Comparative gene expression profiling between optic nerve and spinal cord injury in Xenopus laevis reveals a core set of genes inherent in successful regeneration of vertebrate central nervous system axons.
BMC Genomics, 21(1):540, 05 Aug 2020
Cited by: 7 articles | PMID: 32758133 | PMCID: PMC7430912
Go to all (40) article citations
Data
Similar Articles
To arrive at the top five similar articles we use a word-weighted algorithm to compare words from the Title and Abstract of each citation.
DNA repair pathway selection caused by defects in TEL1, SAE2, and de novo telomere addition generates specific chromosomal rearrangement signatures.
PLoS Genet, 10(4):e1004277, 03 Apr 2014
Cited by: 16 articles | PMID: 24699249 | PMCID: PMC3974649
Mutator genes for suppression of gross chromosomal rearrangements identified by a genome-wide screening in Saccharomyces cerevisiae.
Proc Natl Acad Sci U S A, 101(24):9039-9044, 07 Jun 2004
Cited by: 112 articles | PMID: 15184655 | PMCID: PMC428469
Revisiting the role of the spindle assembly checkpoint in the formation of gross chromosomal rearrangements in Saccharomyces cerevisiae.
Genetics, 228(3):iyae150, 01 Nov 2024
Cited by: 0 articles | PMID: 39268895 | PMCID: PMC11538403
Pathways and Mechanisms that Prevent Genome Instability in Saccharomyces cerevisiae.
Genetics, 206(3):1187-1225, 01 Jul 2017
Cited by: 35 articles | PMID: 28684602 | PMCID: PMC5500125
Review Free full text in Europe PMC
Funding
Funders who supported this work.
NIGMS NIH HHS (3)
Grant ID: R01 GM026017
Grant ID: GM 26017
Grant ID: R37 GM026017