Abstract
Free full text

Therapeutic potential of AAV-mediated MMP-3 secretion from corneal endothelium in treating glaucoma
Abstract
Intraocular pressure (IOP) is maintained as a result of the balance between production of aqueous humour (AH) by the ciliary processes and hydrodynamic resistance to its outflow through the conventional outflow pathway comprising the trabecular meshwork (TM) and Schlemm’s canal (SC). Elevated IOP, which can be caused by increased resistance to AH outflow, is a major risk factor for open-angle glaucoma. Matrix metalloproteinases (MMPs) contribute to conventional aqueous outflow homeostasis in their capacity to remodel extracellular matrices, which has a direct impact on aqueous outflow resistance and IOP. We observed decreased MMP-3 activity in human glaucomatous AH compared to age-matched normotensive control AH. Treatment with glaucomatous AH resulted in significantly increased transendothelial resistance of SC endothelial and TM cell monolayers and reduced monolayer permeability when compared to control AH, or supplemented treatment with exogenous MMP-3.
Intracameral inoculation of AAV-2/9 containing a CMV-driven MMP-3 gene (AAV-MMP-3) into wild type mice resulted in efficient transduction of corneal endothelium and an increase in aqueous concentration and activity of MMP-3. Most importantly, AAV-mediated expression of MMP-3 increased outflow facility and decreased IOP, and controlled expression using an inducible promoter activated by topical administration of doxycycline achieved the same effect. Ultrastructural analysis of MMP-3 treated matrices by transmission electron microscopy revealed remodelling and degradation of core extracellular matrix components. These results indicate that periodic induction, via use of an eye drop, of AAV-mediated secretion of MMP-3 into AH could have therapeutic potential for those cases of glaucoma that are sub-optimally responsive to conventional pressure-reducing medications.
Introduction
The eye is pressurised by a balance in the production of aqueous humour (AH) by the ciliary processes and resistance to its drainage through the trabecular meshwork (TM) and Schlemm’s canal (SC). Located at the apex of the iridocorneal angle, SC is a flattened circular vessel with an average meridional diameter of 233μm in humans (1). AH exits the lumen of SC into collector channels and drains into the episcleral veins that are visible on the surface of the sclera. Precise regulation of aqueous inflow together with outflow resistance is critical in maintaining an average intraocular pressure (IOP) of approximately 16
mmHg in a normal functioning eye (2). In cases of primary open-angle glaucoma (POAG), so-called because the iridocorneal angle remains open without noticeable physical obstruction, resistance to AH drainage through the TM and SC is increased by mechanisms that have yet to be fully elucidated, resulting in elevated IOP (3). This, in turn, results in deformation of the lamina cribrosa—the tissue that structurally supports the optic nerve head—(often referred to as ‘cupping’ of the optic nerve head), damaging retinal ganglion cell axons, leading to ganglion cell degeneration and irreversible blindness.
Lowering IOP remains the only effective treatment for POAG. Topical pressure reducing medications either increase the rate of aqueous outflow through the conventional or unconventional pathway, or reduce aqueous production (3). The U.S. spends $1.9 billion per annum to treat glaucoma, 38–52% of such costs being related to topical pressure reducing medications (4). However, such medications often do not reduce IOP to the desired target pressure and may induce side effects in certain patients. Such patients may then undergo surgical interventions, which have associated risks and complications. Hence, there remains an unmet clinical need for improved methods of disease treatment.
Functional studies have provided evidence that the generation of aqueous outflow resistance is most significant in the juxtacanalicular tissue (JCT—the outer layer of the TM) and inner wall endothelium of SC (5,6). In particular, the extracellular matrix (ECM) composition in the JCT region has been shown to influence outflow patterns and resistance generation (6–11). Competitive disruption or inhibition of integrin-ECM linkages that attach the cell to the ECM, or inhibition of ECM receptors have been associated with increases in endothelial monolayer permeability and transendothelial transport (12–15). This implicates features that are relevant to SC endothelial cells (SCEC) and their supporting basement membrane, such as integrin-ECM interactions, along with other inter-endothelial junctions that govern cell shape, in the control of endothelial paracellular permeability (16). TM cells play an integral role in modulating the ECM of the JCT to preserve AH flow pathways via continual and signal-initiated ECM remodelling (17). The ECM in the JCT region is comprised of a heterogeneous group of fibrous and matrical materials including collagen type IV, proteoglycans, laminin and fibronectin, all of which provide tensile strength and support to surrounding cells. The cribriform plexus, a structure composed of elastic fibres, connects the inner wall endothelium and the ciliary muscle, allowing for JCT expansion in response to IOP elevation (18). ECM reconditioning of these matrix networks can thus induce changes in the actin cytoskeleton through integrin-ECM linkages, enabling F-actin or alpha- smooth muscle actin (α-SMA) to act as markers for active ECM remodelling (19–21). ECM turnover in the conventional outflow pathway is regulated by a family of zinc-dependent endopeptidases, the matrix metalloproteinases (MMPs). These secreted proteases are responsible for the degradation of ECM proteins and cell proliferation, and are thus key components in ECM remodelling and outflow tissue homeostasis (22). MMPs are secreted as inactive protein precursors and are activated when cleaved by extracellular proteinases and other MMPs (23). Levels of MMPs, along with TIMPs (Tissue Inhibitors of Metalloproteinases), have been shown to differ in glaucomatous AH and TM tissue as compared to those from normotensive individuals (24,25). Imbalance in MMP/TIMP ratios, and reduced MMP enzymatic activity, has been correlated with the accumulation of ECM materials in the TM that ultimately leads to an increase in outflow resistance (26,27). Therefore, reduction in ECM turnover within the TM and JCT region as a result of an imbalanced latent-to-activated MMP ratio can be a contributing factor to increased outflow resistance, as observed in glaucoma. It is therefore evident that increasing ECM turnover in outflow tissues may have therapeutic significance by reducing outflow resistance. Here, we set out to develop a gene-based therapy for the delivery of MMP targeting the conventional outflow tissues, with the primary aim of reducing both outflow resistance and IOP. Introduction of MMPs into the anterior chamber of the eye has previously been shown to increase outflow facility in organ-perfused cultures, indicating their therapeutic potential (28–31). Of the many classes of MMPs, MMP-3 (stromelysin-1) presents itself as an attractive candidate for targeting the ECM of outflow tissues. MMP-3 possesses a vast proteolytic target profile including type IV collagen, fibronectin, laminin, elastin, and proteoglycans, all of which are present in the meshwork and JCT regions of the outflow tissues, making this MMP of particular interest (32–36). In addition, MMP-3 can also activate other MMPs, including MMP-1 and MMP-9 (23,37–39), further assisting in the remodelling of ECM components (40–42).
Efficient gene delivery into the anterior segment of the eye is feasible through the use of adenoviral and adeno-associated viral (AAV) vectors. In particular, self-complementary AAV vectors have been shown to have such capability (43–46). Owing to the fact that MMP-3 is a secretory enzyme synthesised in the endoplasmic reticulum, transduction of tissues of the anterior segment with AAV expressing MMP-3 will result in the secretion of the protein into the AH. This will subsequently enable MMP-3 to be delivered into outflow tissues via conventional aqueous flow, potentially facilitating targeted degradation of ECM components and thus increasing aqueous outflow. In this regard, we observed highly efficient transduction of corneal endothelial cells following a single intracameral inoculation of AAV-2/9 expressing MMP-3, and both levels and activity of MMP-3 were significantly elevated in mouse AH following such inoculation. Importantly, AAV-mediated expression of MMP-3 in corneal endothelium, either from a CMV-, or doxycycline-inducible promoter, resulted in a marked increase in outflow facility and reduction in IOP. These observations correlated with structural alterations in the ECM of the outflow tissues, suggesting a mechanism of action for MMP-3 in modulating outflow resistance.
Results
Effects of glaucomatous aqueous humour on SC endothelial and TM cell monolayers
We treated cultured human SCEC monolayers with human glaucomatous (POAG) or control (cataract) AH for 24h, and quantified levels of total secreted and activated MMP-3 in culture media. This was achieved by performing an ELISA and FRET assay, to monitor the degree of cleavage of an MMP-3 specific substrate, on cell media 24
h post-treatment. We did not observe a significant increase in the level of total (latent and active forms) secreted MMP-3 in culture media following treatment with POAG aqueous, with an increase of 0.15 [−0.35, 0.66] ng/ml (mean [95% confidence interval (CI)]) (P
=
0.45, n
=
3, Fig. 1A) over controls. However, activity assays indicated that the MMP-3 secreted in response to POAG aqueous had less enzymatic activity than that of cataract control AH, with an average change of −0.15 [−0.28, −0.02] mU/ml (P
=
0.024, n
=
9 cataract, n
=
7 POAG, Fig. 1B). These observations corroborate results obtained involving other members of the MMP family in POAG aqueous (24) in that the amount of secreted MMP may remain relatively unchanged but its proteolytic activity is reduced.

MMP-3 concentration in glaucomatous AH and the resulting effect on SCEC and HTM monolayers. (A) MMP-3 concentrations in the media of SCEC monolayers treated with either cataract (control) or POAG human AH showed no significant difference after 24h. (B) POAG aqueous-treated SC media samples from (A) were found to have an average change in MMP-3 proteolytic activity of -0.15 [−0.28, −0.02] mU/ml compared to control media. (C) Addition of POAG aqueous humour onto SC monolayers resulted in an average increase in TEER of 102% compared to controls. (D) Treatment of HTM cells with human aqueous also increased TEER value. (E,F) SCEC and HTM subjected to AH were tested for cellular permeability using a FITC-Dextran flux assay respectively. Decreased permeability to a 70
kDa dextran was observed in response to POAG rather than cataract AH. Graphs show mean with 95% CI error bars. CAT = cataract, POAG = primary open-angle glaucoma. Figures A-F were analysed with a Student’s t-test. NS = non-significant. Symbols *, ** and *** denote P values of
<
0.05, < 0.01 and < 0.001, respectively.
Effects of glaucomatous AH on the permeability of SCEC and human TM (HTM) monolayers were determined by trans-endothelial electrical resistance (TEER) and FITC-dextran flux assays. Treatment of cultured SCEC monolayers with POAG AH resulted in increased TEER by an average of 102% after 24-h treatment compared to control AH (−7%), displaying an average absolute increase of 19.82 [15.82, 23.81] Ω.cm2 (P<
0.0001, n
=
6 cataract, n
=
12 POAG, Fig. 1C). Similarly, HTM responded with an increase of 9.79 [5.55, 14.05] Ω.cm2 in response to glaucomatous AH, (P
=
0.0002, n
=
8, Fig. 1D). Glaucomatous AH also reduced paracellular flux, as measured by permeability co-efficient (Papp), to dextran of 70
kDa as compared to cataract controls, with a mean difference of 0.14 [0.05, 0.22] cm/s × 10−8 (P
=
0.009, n
=
3 cataract, n
=
3 POAG, Fig. 1E). A reduction in HTM permeability was also observed with a mean difference of 0.17 [0.09, 0.23] cm/s × 10−9 (P
=
0.005, n
=
8 cataract, n
=
7 POAG, Fig. 1F).
Treatment of outflow cell monolayers with recombinant human MMP-3 increases permeability with concomitant reductions in TEER
In contrast to the negative effects of glaucomatous AH on SCEC and HTM permeability and resistance, we observed that treatment of cultured monolayers with 10ng/ml of active recombinant human MMP-3 reduced TEER values on average by 5.62 [2.92, 8.32] Ω.cm2 greater than inactivated MMP-3 controls over the course of 24
h for SCEC (P
<
0.0001, n
=
8, Fig. 2A) and by 4.29 [0.11, 8.48] Ω.cm2 for HTM (P
=
0.0137, n
=
8, Fig. 2B) respectively. Permeability assays complemented these data as increases in paracellular flux of 70
kDa FITC-dextran by 0.14 [0.12, 0.18] cm/s × 10−9 (P
<
0.0001, n
=
8, Fig. 2C) were observed in SCEC, and 0.04 [0.01, 0.06] cm/s × 10−9 (P
<
0.01, n
=
8, Fig. 2D) in HTM monolayers when comparing treatments of MMP-3 to its inactivated counterpart control: TIMP-1 incubated with MMP-3. To rule out cytotoxicity as a reason for the observed changes in paracellular permeability, a cell viability assay was undertaken. Based on data shown in Figure 2E, for concentrations below 36
ng/ml MMP-3, the average SCEC cell viability for n
=
3 will exceed 85%. Greater tolerability was observed in HTM cases, retaining an average viability of at least 85% for MMP-3 concentrations up to 151
ng/ml (n
=
3, Fig. 2F).
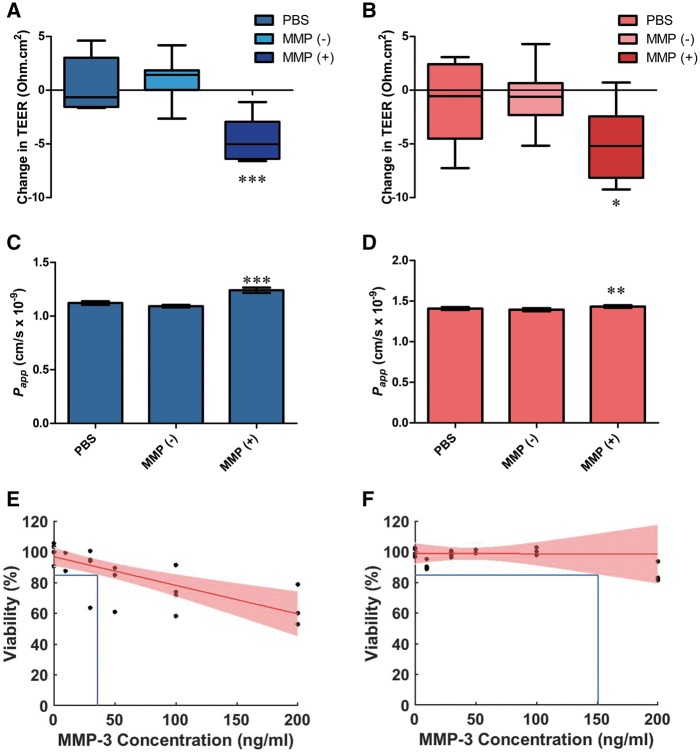
Effect of recombinant human MMP-3 on paracellular permeability in HTM and SCEC cell monolayers. SCEC and HTM cells were treated with 10ng/ml recombinant MMP-3 for 24
h, using PBS and inactivated MMP-3 (incubation with TIMP-1, MMP(−)) as vehicle and negative controls respectively. (A) SCEC and (B) HTM both show reductions in TEER values after treatment of 4.6 [2.9, 6.2] and 5 [2.2, 7.8] Ohms.cm2 respectively. Permeability to a 70
kDa dextran was increased in treated cells (MMP (+)) in both (C) SCEC and (D) HTM. (E) An average viability of 85% was expected for SCEC with MMP-3 concentrations up to 36
ng/ml. (F) 85% viability is retained on average in HTM cells at concentrations up to 151
ng/ml MMP-3. A, C and E in blue represent SCEC data, whereas B, D and F in red represent HTM data.
Treatment of SCEC and HTM monolayers with active recombinant human MMP-3 induces remodelling and degradation of ECM components
In order to attribute increases in permeability to the ECM remodelling effects associated with MMP-3, SCEC and HTM monolayers were both treated as above with 10mg/ml MMP-3 for 24
h. Following treatment, we observed changes in the staining pattern and intensity of a number of ECM proteins by immunocytochemistry. Specific collagen IV staining was localised to perinuclear areas and cytoplasm in both SCEC and HTM cells (Fig. 3A and B). In particular, we observed a decrease in the staining intensity around perinuclear areas in treated cells as compared to controls. A-SMA fibres facilitating cell-cell contacts in SCEC localised specifically to the cytoplasm and cytoskeleton, and MMP-3 treatment led to an attenuation of fibre bundles with thinning of intercellular connections (Fig. 3C). Fluorescent images of F-actin in HTM monolayers also revealed constricted actin bundles and a reduced tendency for bundle crossovers (Fig. 3D). Immunofluorescence staining of laminin in SCEC and HTM cells showed diminished cytoplasmic localisation and reduced network complexity and multiplicity in MMP-3 treated cells as compared to control staining intensity of laminin (Fig. 3E and F). To visualise fibronectin clearly without cellular interference, decellularisation was performed after MMP-3 treatment to isolate the ECM scaffold from the cell monolayer. Fluorescent images show significant perturbation of fibronectin network in treated cells as opposed to the linear cellular organisation observed in control cells (asterisk, Fig. 3G and H). To quantitatively demonstrate remodelling of these proteins, western blot analysis was performed on both cell lysate and media fractions of SC and HTM cell monolayers (Supplementary Material, Fig. S1). Specific bands were observed at 300
kDa for collagen IV, 42
kDa for α-SMA, 220
kDa for laminin and 290
kDa for fibronectin. A significant reduction of collagen IV (P
=
0.01, P
=
0.01) α-SMA (P
=
0.04, P
=
0.04) and laminin (P
=
0.04, P
=
0.03) were observed in SC and HTM whole cell lysate samples respectively (n
=
4 for all cases). Collectively, these data clearly illustrate that MMP-3 mediates remodelling of ECM components in both SCEC and HTM cell monolayers.
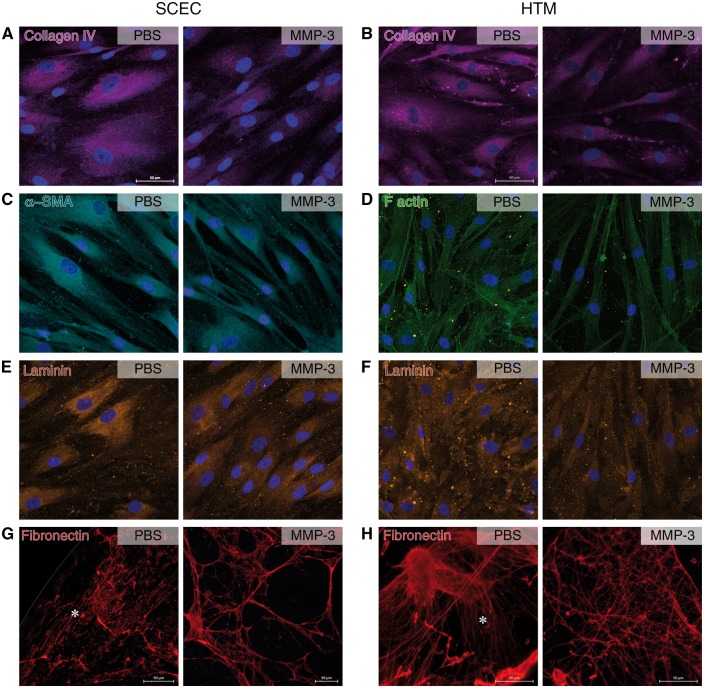
Remodelling of ECM components in SCEC and HTM cell monolayers. Immunocytochemistry shows various remodelling artefacts on core ECM components in SCEC and HTM cells in response to MMP-3 treatment. (A,B) Collagen IV appears to have reduced intensity in both cell types after treatment. Collagen IV is concentrated around cells in controls but shows reduced spread after treatment, fibrils barely protruding past the cell nuclei. (C) Alpha smooth muscle fibres extend the width of the cell towards a neighbouring cell. Treated samples show that these fibre bundles have constricted, leading to multiple thin connections between cells. (D) HTM F-actin staining depicts a slight thinning of filament bundles and a reduction of filament branching post MMP-3 treatment. (E,F) Laminin expression exhibits a modest reduction in staining intensity in both cell types, and a reduction in network complexity in TM cells. (G,H) Fibronectin was visualised after decellularisation, depicting linear and organised strands in PBS controls, as denoted by an asterisk. Treatment groups lacked a linear network, and instead showed a disjointed, porous network. Scale bars represent 50μm. Left column pairs = SCEC, right column pairs = HTM.
Intracameral inoculation of AAV-2/9 expressing a CMV-driven MMP-3 gene efficiently transduces corneal endothelium and results in elevated levels of MMP-3 in aqueous humour
AAV-mediated transduction of corneal endothelium could, in principle, serve as an efficient means of expressing and secreting MMP-3 into AH. The advantage of such an approach is that the natural flow dynamics of AH will allow transportation of secreted MMP-3 towards the outflow tissues (Fig. 4A). We evaluated the efficiency of a number of AAV serotypes with either single stranded or self-complementary genomes to deliver MMP-3 to the outflow tissues. 2µl of viral particles (2×
1012 vector genomes/ml) of each serotype, expressing a CMV-driven eGFP reporter gene (Fig. 4B) were intracamerally inoculated into wild type C57BL/6 mice and eyes examined via fluorescent microscopy at 3 weeks post-inoculation. Extensive expression of the reporter gene was observed in the corneal endothelium of eyes injected with non-self-complementary AAV-2/9 (Fig. 4C top), with no fluorescence being detectable in the outflow tissues themselves using this construct. Hence, the eGFP cDNA from AAV-2/9 was exchanged with murine MMP-3 cDNA to generate AAV-MMP-3, and similar inoculation resulted in MMP-3 expression that was prominently detected in the corneal endothelium and not in null controls (Fig. 4C, bottom). No significant difference in central corneal thickness was detected following AAV inoculation between treated (116.7 [112.5, 120.9] μm) and control eyes (116.4 [113.6, 119.1] μm) (n
=
4, Supplementary Material, Fig. S2). Corneas also appeared clear with no signs of cataracts upon visual inspection.
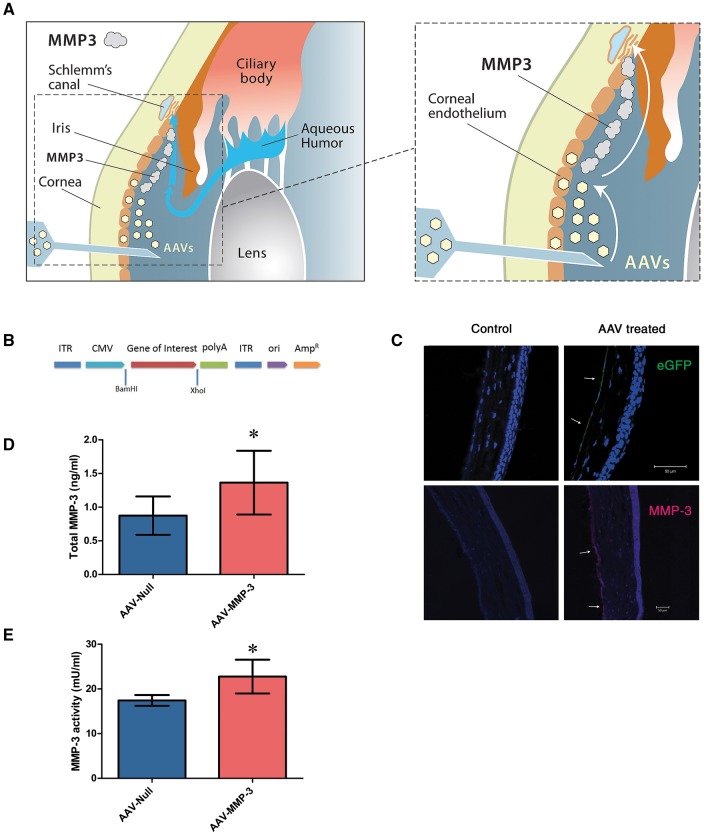
AAV-2/9 mediated MMP-3 expression in the corneal endothelium. (A) Diagrams illustrating the therapeutic concept addressed in this study. AAV-2/9 transduces the corneal endothelium upon intracameral inoculation (left). MMP-3 molecules are secreted into the AH from this location and are transported toward the outflow tissue by the natural flow of the aqueous (right). (B) A schematic diagram of the AAV-2/9 vector used for the expression of either eGFP or MMP-3. Murine MMP-3 cDNA was sub-cloned into the pAAV-MCS plasmid and constitutively driven by a CMV promoter (AAV-MMP-3). (C) Immunohistochemistry images of corneas from WT murine eyes intracamerally inoculated with AAV-2/9 expressing eGFP. AAV virus containing a CMV promoter demonstrates transduction and expression at the corneal endothelium (marked with arrows). Using the AAV-MMP-3 virus, MMP-3 was detected at the corneal endothelium in treated eyes only, denoted by arrows. (D) ELISA was performed on murine AH 4 weeks post-injection of virus. MMP-3 concentrations had increased by an average of 0.49 [0.11, 0.87] ng/ml in AAV-MMP-3 treated eyes (paired Student’s t-test). (E) Aqueous MMP-3 activity was significantly increased by an average of 5.34 [1.12, 9.57] mU in AAV-MMP-3 treated eyes. Scale bars represent 50μm. Asterisk symbol denotes a P value of
<
0.05.
The level of total MMP-3 in the AH of twelve inoculated animals was quantified using enzyme-linked immunosorbent assay (ELISA), and we observed a significant average increase in total MMP-3 protein of 56%, 1.37 [0.89, 1.84] ng/ml as compared to 0.87 [0.59, 1.12] ng/ml for control AAV (P=
0.016, n
=
12, Fig. 4D). The activity of AAV-mediated production of MMP-3 was also assessed using FRET, and a significant increase in activity of 34 [6.86, 61.14] % was observed, on average, in AAV-MMP-3 treated eyes compared to contralateral controls (P
=
0.0164, n
=
17, Fig. 4E).
Intracameral inoculation of AAV-2/9 expressing an MMP-3 gene increases outflow facility and reduces IOP in murine eyes
In order to determine the effect of AAV-mediated expression of MMP-3 from the corneal endothelium on aqueous outflow, the conventional outflow facility was measured using the recently developed iPerfusion system designed specifically to measure conventional outflow facility in mice (47). Wild type mice were intracamerally injected with 1x1011 vector genomes of AAV-MMP-3, and contralateral eyes received the same quantity of AAV-Null. Four weeks post-inoculation, eyes were enucleated and perfused in pairs over incrementing steps in applied pressure. A representative flow-pressure plot provided in Supplementary Material, Figure S3A describes the relationship between flow rate (Q) at each pressure (P) step in both AAV-MMP-3 (red) and AAV-Null (blue) eyes. Furthermore, the relative percentage difference in facility within each data pair is depicted in Supplementary Material, Figure S3B (left). The resulting facility data presented in Figure 5A and B clearly illustrate that control eyes have an average facility of 8.44 [6.14, 11.60] nl/min/mmHg with treated eyes having an average facility of 11.73 [8.05, 17.08] nl/min/mmHg. There is, therefore, an average increase in outflow facility of 39 [19, 63] % in pairs, between treated eyes and their contralateral controls (P=
0.002, n
=
8 pairs).
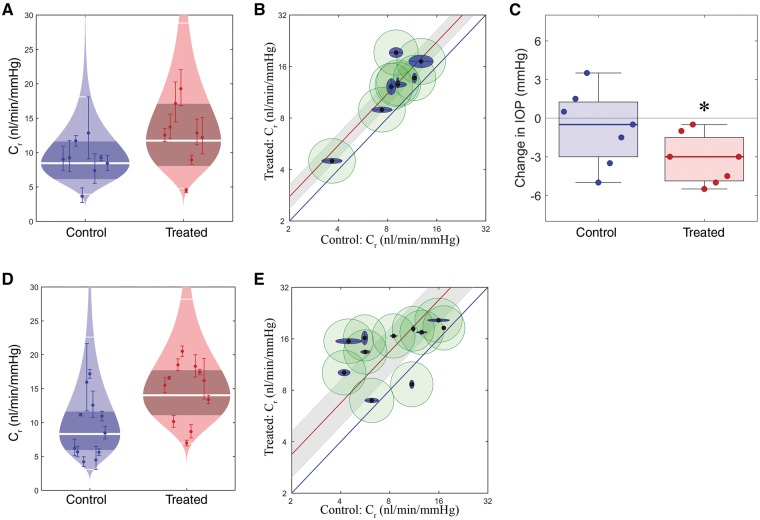
Effect of ECM remodelling on outflow facility and IOP. (A) ‘Cello’ plot depicting individual outflow facility values for eyes at 8mmHg (Cr) and statistical distribution of both control (AAV-Null) and experimental (AAV-MMP-3) groups. Each point represents a single eye with 95% CI on Cr. Log normal distribution is shown, with the central white band showing the geometric mean and the thinner white bands showing two geometric standard deviations from the mean. The shaded region represents the 95% CI on the mean. (B) Paired outflow facility plot. Each inner point represents an eye pair, with log-transformed facilities of the control eye plotted on the x axis, and treated eye on the y axis. Outer blue and green ellipses show uncertainties generated from fitting the data to a model, intra-individual and cannulation variability respectively. Average increase is denoted by the red line, enclosed by a grey 95% CI, indicating significantly increased facility (does not overlap the blue unity line). (C) Box plots showing the change in IOP in treated and control eyes. Boxes show interquartile range and error bars represent the 5th and 95th percentiles. A significant reduction in IOP is observed in AAV-MMP-3 treated eyes (Wilcoxon signed-rank test). (D-E) Cello and paired facility plots for inducible AAV data sets.
As the major pathology in POAG is IOP elevation, and an increased outflow facility was observed, tonometric IOP measurements were taken both immediately before (pre), and four weeks after (post) intracameral injection of AAV-2/9 expressing MMP-3 or a null vector in the case of the control. Differences between pre- and post-injection IOP were calculated using the non-parametric Wilcoxon matched-pairs signed rank test. Eyes treated with AAV-Null had no significant change in IOP −0.5±
2.9
mmHg (median
±
median absolute deviation (MAD), P
=
0.61, n
=
7, Wilcoxon signed-rank test with a theoretical median IOP change of 0) after treatment. In comparison, when treated with AAV-MMP-3, median IOP significantly decreased by 3.0
±
2.9
mmHg (P
=
0.022, n
=
7, Fig. 5C). The IOP difference in AAV-MMP-3 treated eyes was significantly greater than the IOP difference in the contralateral AAV-Null treated eyes by 2.5
±
0.7
mmHg (P
=
0.034, n
=
7, Fig. 5C).
Controlled periodic activation of MMP-3
To incorporate a control mechanism for the secretion of MMP-3 from corneal endothelium, we first introduced AAV-2/9 expressing eGFP under the control of a tetracycline-inducible promoter into the anterior chambers of both eyes of wild type mice. After 3 weeks, mice were treated with a regime of one drop of 0.2% doxycycline (a tetracycline derivative) two times per day (approx. 8h between each application) for 10–16 days in one eye only. PBS was administered onto the contralateral eye as a control. As illustrated in Supplementary Material, Figure S4, extensive expression of the reporter gene was observed only in the corneal endothelium, and no expression was observed in the contralateral control. Following this, we replaced the reporter cDNA with murine MMP-3 cDNA and the resulting AAV (Induc. AAV-MMP-3) was injected into the anterior chambers of animals at 1x1011 viral genomes per eye. Using the inducible eGFP virus (Induc. AAV-eGFP) as a contralateral control, expression was induced by administering doxycycline (as above) to both eyes. Contralateral eyes were perfused as above, the control group exhibiting an average facility of 8.30 [5.75, 11.26] nl/min/mmHg and the MMP-3 treatment group resulting in a facility of 14.01 [11.09, 17.72] nl/min/mmHg. Paired, these eyes exhibit an average increase in outflow facility of 68 [24, 128] % (P
=
0.004, n
=
11, Fig. 5D and E). The relative difference in facility within individual pairs is presented in Supplementary Material, Figure S3B (right). This observation strongly supports the concept that MMP-3 expression could be induced in a controlled and reversible manner, with periodic IOP measurements utilised to guide the induction of expression.
Ultrastructural analysis of AAV-MMP-3 treated eyes
In order to evaluate whether the AAV-MMP-3 treatment affects the morphology of the eye and the TM including the inner wall of SC, ultrastructural investigation was performed in four pairs of mouse eyes. Corneas appeared translucent and healthy on visual inspection during enucleation. Semi-thin sections clearly demonstrated that there were no signs of an inflammatory reaction, either in the TM or in the cornea, uvea or retina (Fig. 6A and B). Ultrastructural analysis of control eyes revealed normal outflow structural morphology, cell-matrix attachments and cell-cell connections between the SC and TM. The inner wall endothelial cells formed foot-like connections with subendothelial TM cells, as well as connections to underlying elastic fibres and discontinuous basement membrane (Fig. 6C). However, in some regions of treated eyes, especially those with a prominent SC lumen and scleral spur-like structure typical of the nasal quadrant (48), there appeared to be more optically empty space directly underlying the inner wall endothelium of SC, compared to AAV-Null controls (Fig. 6D). In these optically empty spaces, foot-like extensions of the inner wall to the sub-endothelial layer were absent or disconnected from the subendothelial cells or elastic fibres (Fig. 6D and E). Occasionally, we observed an accumulation of ECM clumps beneath the inner wall that were not observed within the controls (Fig. 6F) and may represent remnants of digested material.

Transmission electron microscopy (TEM) analysis of ECM remodelling in outflow tissues. Semi-thin sections of the iridocorneal angle in mouse eyes treated with either (A) AAV-Null or (B) AAV-MMP-3. AAV-MMP-3 treated eyes show greater inter-trabecular spaces in outer trabecular meshwork (TM) than controls. Scale bar denotes 50μm. (C,D) Transmission electron micrograph of the inner wall of Schlemm’s Canal (SC) and the outer TM. (C) Control eye illustrating normal attachment between foot-like extensions of the inner wall endothelium and subendothelial cells (arrowheads), as well as with the discontinuous basement-membrane material underlying the inner wall endothelium (arrows). (D) Representative TEM image of an MMP-3 treated eye showing a disconnection of the inner wall endothelium from the subendothelial cells and the ECM (arrowheads). The widened subendothelial region lacks basement-membrane material and other ECM components. (E,F) Higher magnification of the inner wall of a treated eye. (E) Foot-like extensions of the inner wall endothelium (E) have disconnected from the subendothelial cells and the ECM (arrowheads), and the lack of ECM in this region is shown. (F) In other regions of treated eyes, clumps of presumably degraded ECM-material are localised underneath the inner wall of SC (asterisk). Such clumps of ECM are not present in controls. Scale bars are denoted on each image. CB = ciliary body, I = iris, R = retina. (G). Morphometric measurements of the optically empty space immediately underlying SC from four regions of contralateral eyes treated with AAV-MMP-3 (red data points) or AAV-Null (blue data points). Bars indicate average values for each eye. Contralateral eyes are presented immediately next to one another.
We quantified the optically empty length directly underlying the inner wall of SC. In control eyes, the percent optically empty length in any one region ranged from 19 to 49% with an average of 37%. In the treated eyes, the equivalent range was 39–76% with an average of 59% (Fig. 6G). The differences between control and experimental eyes for each pair ranged from 16 to 26%, which corresponded to a statistically significant increase in the proportion of open space underlying the inner wall with AAV-MMP-3 relative to AAV-Null (P=
0.002, n
=
4; paired Student’s t-test). These data indicate that reduced ECM material in the TM and along the inner wall of SC is associated with AAV-MMP-3 treatment and may explain the enhanced outflow facility and IOP reduction. Furthermore, these morphological changes, because they were absent from controls, could not be attributed to an inflammatory or lytic response to AAV alone.
Discussion
Matrix metalloproteinases are key regulators in the remodelling of extracellular matrices in the JCT region of the TM. Dysregulation of MMP expression and loss of MMP/TIMP homeostasis in glaucomatous AH have been associated with abnormal fibrillary ECM accumulation in the JCT region of POAG eyes (49–53). Furthermore, perfusion of anterior segments with purified MMPs increased outflow facility, while the use of metalloproteinase inhibitors (TIMP, minocycline) reduced outflow rates (30). Consistent with these findings, upregulation of MMPs following clinical laser treatment has been associated with the ocular hypotensive effect of trabeculoplasty (54,55). It is therefore apparent that the reduction in ECM turnover within the outflow tissues contributes to increased outflow resistance, and strategies specifically targeting outflow ECM may be effective in reducing outflow resistance. In this study, we focused on the development of a gene-based therapy for the delivery of MMP-3 into outflow tissues to facilitate aqueous outflow and reduce IOP.
It has been reported that the activity of a range of regulatory cytokines and growth factors found in AH directly impacts permeability in the outflow tissue, and many of these are known to be dysregulated in POAG AH (56,57). In particular, cytokines such as IL-1, TGF and TNF are known to influence the expression and secretion of ECM modulators, including MMPs in outflow tissues (58–60). It was therefore of interest to assess how POAG AH may affect the MMP-3 secretion and their relative activity in outflow cell culture systems.
We performed permeability assays with AH-treated SCEC and HTM monolayers to demonstrate that dysregulated MMP’s in POAG AH influence monolayer permeability via modified ECM remodelling. (12,61). SCEC monolayers showed decreased permeability in vitro in response to glaucomatous AH, and this decrease was associated with a reduction in extracellular MMP-3 activity. HTM cells exhibited similar reductions in permeability. In contrast, treatment of cell monolayers exclusively with recombinant MMP-3 elevated monolayer permeability in comparison to controls, suggesting that MMP-3 could correct the permeability lowering effects of POAG aqueous. Because MMP-3 has previously been associated with apoptotic behaviour in Chinese hamster ovary cells and osteoclasts (62,63), we evaluated the effect of a wide concentration range of MMP-3 on SCEC and HTM viability. This way, we confirm that increases in paracellular permeability were not related to an MMP-3 cytotoxic effect, but rather its proteolytic activities, which these data support. More importantly, even lower concentrations of MMP-3 were detected in vivo in murine AH after AAV-mediated MMP-3 secretion (1.37 [0.89, 1.84] ng/ml, Fig. 4D) than those used in in vitro experiments. Collectively, these results indicate that a plausible target for MMP-3 activity is likely to be the ECM of TM and the inner wall endothelium of SC. This further contributes to the body of evidence demonstrating that the molecular pathological effects of glaucomatous AH are due, in part, to dysregulations of MMP-mediated remodelling and that induced elevations in MMP-3 expression in outflow tissues will have an enhancing effect on increasing aqueous outflow.
Previously, perfusion of explants of human anterior segments with a mixture of MMP-3, -2, -9 has been shown to result in an increase in outflow facility to 160% of baseline level (30). It has also been demonstrated that increases in IOP lead to an up-regulation of MMP-2, -3 and -14 through mechanical stretching of the TM and a reduction in TIMP2 (31,64–68). Such observations support the concept that controlled expression of MMPs within the anterior chamber holds therapeutic potential in regard to facilitating aqueous outflow. The inner wall endothelium of SC along with its basement membrane and JCT modulate the resistance to outflow. The interconnections between all components responsible for the outflow resistance generation is essential to maintain the homeostasis of outflow drainage (11). Thus, targeting the ECM for remodelling in the JCT region using MMPs may effectively increase permeability of surrounding cells, thus increasing AH outflow rate and lowering IOP.
Owing to the fact that MMP-3 is a secretory enzyme, transduction of the corneal endothelium with AAV-MMP-3 will result in the secretion and delivery of the protein to the outflow tissues by following the natural flow of AH in the anterior chamber. Ex vivo studies in human corneas or corneal fibroblasts have demonstrated the potential and efficiency of delivering AAV to this tissue type (69,70). Successful transfection of different layers in the rabbit cornea by recombinant AAV further supports the potential of recombinant/exogenous protein delivery from corneal cells (71). Thus, it is likely that secreted MMP-3, expressed by corneal endothelia, will be directed toward the outflow tissue and activated with the aid of existing endogenous MMP-3 after which it will then be available for remodelling a range of ECM components. Attaining exclusive AAV expression in the cornea was obtained by using a single stranded AAV-2/9, as although single stranded AAV may enter other cells such as the TM, self-complementary viruses are required for sufficient DNA replication, and hence transduction of these cells (72).
The current data provide a direct proof of concept that AAV-mediated expression of MMP-3 from corneal endothelium decreases IOP with a concomitant increase in the rate of AH outflow through the drainage channels ex vivo. A number of parameters will require further refinement in order to address the translational feasibility of this approach. Although significant elevations in transient MMP-3 were found in murine AH post-treatment with AAV-MMP-3, these were well within tolerable limits as defined by our in vitro experiments (Fig. 2E and F). These MMP-3 elevations in AH (Fig. 4D), along with visually translucent corneas of normal thickness (Supplementary Material, Fig. S2), suggests that MMP-3 is preferentially secreted apically into the AH. MMP-3 expressed in the corneal endothelial cell layer is in the inactive form, which requires secretion for cleavage-induced activation, and is therefore unlikely to induce remodelling or damage to the endothelium itself. Activation is likely to occur in the AH after secretion, or extracellularly within the outflow tissues in the presence of other proteases. The observed elevation in aqueous MMP-3 activity indicates that activation at least begins in the AH (Fig. 4E). However, sustained expression of MMP-3, as would occur following permanent transfection of cells of the anterior chamber, could result in off-target proteolysis over time. A potentially more effective approach will be to employ an inducible promoter to drive MMP-3 expression on a periodic basis once the virus has been introduced into the anterior segment tissues of the eye. It is of note that the use of glucocorticoid-inducible promoters has been explored in this regard in adenoviral and AAV delivery systems to express MMP-1 in tissues of the TM (46). However, the use of a steroid response promoter may not be ideally suited from a therapeutic standpoint in humans, as activation of the promoter would require continuous exposure to steroid components, which can lead to abnormal IOP elevation (73–75). Glucocorticoids have also been shown to influence gene expression, which may play a pathogenic role in developing hypertension (76). Hence, we explored the effectiveness of a tetracycline-inducible system to express MMP-3 from the corneal endothelia, which allows for controllable and reversible activation by topically applied eye drops. We noted that activation of AAV for over 10 days was sufficient to significantly increase outflow facility ex vivo in mice and suggest that incorporation of other tetracycline derivatives may further enhance the effectiveness of the promoter and hence MMP-3 production. Our observed increase in facility of 68 [24, 128] % rivals that of conventional prostaglandin analogues which are in current use to treat glaucoma, noted to have an increase of 56 [−4, 154] % in the case of PDA205, also using the iPerfusion system (47).
To date, in studies utilising MMPs for increasing outflow or reducing IOP little emphasis has been given to the mechanism of action. Here, we show that MMP-3-mediated remodelling of specific ECM components is likely responsible for increased outflow, and hence, decreased IOP. Reductions in intensity and distribution of core ECM materials including collagen IV and laminin were observed in vitro, along with the disorganisation of the fibronectin meshwork and constrictions in the actin skeleton. These modifications suggest the development of a porous nature within the ECM of these monolayers. Semi-quantitation via western blot analysis coincides with these results, showing significant reductions in collagen IV, α-SMA and laminin proteins in the cell lysate fraction, where ECM proteins are likely to reside as no significant changes were displayed in media samples. It is reasonable to assume that these extracellular changes contribute to the observed alterations in electrical resistance and paracellular flux. Ultrastructural analysis of AAV-MMP-3 treated mouse eyes also showed reductions in ECM material at the sub-endothelial/JCT region, including areas of degraded ECM and widened inter-trabecular spaces. Upon quantification, these areas optically lacking ECM material were found to be consistently increased in response to MMP-3 both between regions of the anterior chamber and between treated eyes. Tight junctions remained intact after incubation with AAV-MMP-3 (Supplementary Material, Fig. S5), contrary to previous studies which have shown tight junction degradation by MMPs (77,78); validating that MMP-3 may primarily augment cell monolayer permeability via other mechanisms such as alteration of ECM components. These data indicate that a reduction in ECM material in the TM and inner wall of SC is responsible for the enhancement of outflow facility and consequently lowering of IOP in the treated eyes. The data also show that the reduction in ECM is not due to an inflammatory response that secondarily induces lytic enzymes in the treated eyes but most likely to the induction of MMP-3 through the treatment directly.
We show here for the first time that a topical eye drop regime can control the expression of a gene therapy vector utilised to reduce outflow resistance and IOP through ECM remodelling. The current approach may hold substantial potential as an effective human therapy should long-term safety and efficacy prove successful in non-human primates.
Materials and Methods
Cell culture
Human SCEC were isolated, cultured and fully characterised according to previous protocols (79–81). Briefly, cells were isolated from the SC lumen of human donor eyes using a cannulation technique. Isolated cells were tested for positive expression of VE-cadherin and fibulin-2, but absence of myocilin induction upon treatment with 100nM dexamethasone for 5 days. Confluent cells displayed a characteristic linear fusiform morphology, were contact inhibited and generated a net transendothelial electrical resistance (TEER) greater than 10 Ω.cm2. TEER values were confirmed again prior to MMP-3 treatments. SCEC strains used were SC82 and SC83 between passages 2 and 7. Dulbecco’s modified eagle medium (Gibco, Life Sciences) 1% Pen/Strep/glutamine (Gibco, Life Sciences) and 10% foetal bovine serum (FBS) performance plus (Gibco, Life Sciences) was used as culture media in a 5% CO2 incubator at 37
°C. Cells were passaged with trypsin-EDTA (Gibco-BRL) and seeded into 12 well or 24 well transwell plates (Costar, Corning). Human trabecular meshwork (HTM) cells were isolated and fully characterised according to the procedures described in (82–85). TM tissue is removed from human donor eyes using a blunt dissection technique, and TM cells are dissociated from the tissue using a collagenase digestion protocol as previously described (82). Isolated cells are characterised by their dramatic induction of myocilin protein following treatment with dexamethasone (100
nM) for 5 days as detailed before (79). HTM123 and HTM134 cells were cultured similar to SCEC’s and matured for one week in 1% FBS media prior to treatment.
Human AH samples (detailed below) were added 1:10 to fresh media for cellular treatment for use with TEER and permeability assays as described below.
Recombinant human active MMP-3 (ab96555, Abcam) was added to cell media at a concentration of 10ng/ml for TEER, permeability assays, western blotting and immunocytochemistry as described below. Inactivated MMP-3 controls were achieved by incubating active MMP-3 (10
ng/ml) with recombinant human active TIMP-1 (100
ng/ml, ab82104, Abcam) in cell media for 1
h prior to treatment.
Animals
Animals and procedures used in this study were carried out in accordance with regulations set out by The Health Products Regulatory Authority (HPRA), responsible for the correct implementation of EU directive 2010/63/EU. 8–11-week-old male and female C57BL/6 mice were used in all experimentation outlined in this study. Animals were bred and housed in specific-pathogen-free environments in the University of Dublin, Trinity College and all injections and IOP measurements complied with the HPRA project authorisation number AE19136/P017.
Patient aqueous humour samples
Human aqueous was obtained from the Mater Misericordiae Hospital, Dublin, Ireland. Upon informed consent, AH samples were collected from both POAG and control patients undergoing routine cataract surgery. The criteria for POAG was defined as the presence of glaucomatous optic disc cupping with associated visual field loss in an eye with a gonioscopically open anterior drainage channel, with an intraocular pressure>
21
mmHg (86). The samples were taken immediately prior to corneal incision at the start of the procedure using a method described previously (87). Human AH collection conformed to the WMA Declaration of Helsinki and was approved by the Mater Misericordiae University Hospital Research Ethics Committee.
TEER measurement
Electrical resistance values were used as a representative of the integrity of the endothelial cell-cell junctions. Cells grown on Costar transwell-polyester membrane inserts with a pore size of 0.4μm were treated with 10
ng/ml MMP-3 as described above. TEER readings were measured before and 24
h after treatment. An electrical probe (Millicell ERS-2 Voltohmmeter, Millipore) was placed into both the apical and basal chambers of the transwells and a current was passed through the monolayers, reported as a resistance in Ω.cm2. A correction was applied for the surface area of the membrane (0.33
cm2) and for the electrical resistance of the membrane (blank transwell).
Permeability assessment by FITC-dextran flux
The extent of monolayer permeability was assessed by the basal to apical movement of a tracer molecule through the monolayer. Measures of permeability were taken 24h after treatment immediately after TEER values, keeping experimental set-up identical to that of TEER readings. The permeability protocol was repeated as described in (88). A 70kDa fluorescein isothiocyanate (FITC)-conjugated dextran (Sigma) was added to the basal compartment of the transwell. Fresh medium was applied to the apical chamber and aliquots of 100
μl were taken every 15
min for a total of 120
min, replacing with fresh media. Sample aliquots were analysed for FITC fluorescence (FLUOstar OPTIMA, BMG Labtech) at an excitation wavelength of 492
nm and emission wavelength of 520
nm. Relative fluorescent units (RFU) were converted to their corresponding concentrations by interpolating from a known standard curve. Corrections were made for background fluorescence and the serial dilutions generated over the experiments time course. Papp values were calculated representing the apparent permeability coefficient for control (PBS) and treatment (10
ng/ml MMP-3). This was achieved via the following equation:
Where dM/dT is the rate of appearance of FITC-dextran (FD) (μg/s) in the apical chamber from 0 to 120min after the introduction of FD into the basal chamber. A is the effective surface area of the insert (cm2) and C0 is the initial concentration of FD in the basal chamber.
Cell viability
Cultured cells were treated with increasing concentrations of recombinant human MMP-3 (ab96555, Abcam) from 0 to 200ng/ml. Cell viability was assessed 24
h post-treatment with MMP-3 using a CellTitre 96® AQueous One Solution Cell Proliferation Assay (Promega). Cell media was aspirated and a 1 in 6 dilution of the supplied reagent in media was added to the cell surface. Cells were incubated at 37
°C for 1
h and the media/reagent was transferred to a 96-well plate for reading by spectrophotometry (Multiskan FC, Thermo Scientific) at 450
nm. Standard in vitro viability calculations fail to consider sample size and the biological significance of the data. Hence, a modified approach was taken to determine at which concentration SCEC’s show a reduced tolerability to MMP-3. This was defined at an average of 85% viability over three cell samples. This conservative value ensures that a cell population would remain viable and still be able to proliferate. Anything lower should be regarded as MMP-3 intolerability i.e. reduced cell proliferation or cell death. Control samples (0
ng/ml MMP-3) were normalised to 100% viability and a linear model fitted to the normalised data. The MMP-3 concentration at which cells had an average of 85% viability was interpolated from the lower 95% confidence bound from this linear model. This value represents the concentration of MMP-3 at which the average of three cell samples would have a 97.5% chance of retaining a greater to or equal than 85% viability.
Immunocytochemistry (cell monolayers)
Immunocytochemistry was performed to visualise changes in ECM composition in response to MMP-3. Human SCEC and HTM were grown on chamber slides (Lab-Tek II) and fixed in 4% paraformaldehyde (pH 7.4) for 20min at room temperature and then washed with PBS for 15
min. Cell monolayers were blocked in PBS containing 5% normal goat serum (10658654, Fischer Scientific) and 0.1% Triton X-100 (T8787, Sigma) at room temperature for 30
min. Primary antibodies of collagen IV (ab6586, Abcam), α-SMA (ab5694, Abcam), laminin (ab11575, Abcam) and F-actin (A12379, ThermoFisher Scientific) were diluted at 1:100 in blocking buffer and incubated overnight at 4
°C. Secondary antibodies (ab6939, Abcam) were diluted at 1:500 in blocking buffer and then incubated for 2
h at room temperature. Following incubation, chamber slides were mounted with aqua-polymount (Polyscience) after nuclei-counterstaining with DAPI. Fluorescent images of SCEC monolayers were captured using a confocal microscope (Zeiss LSM 710), and processed using imaging software ZEN 2012.
For clear fibronectin (ab23750, Abcam) staining, cells were grown on cover slips and subsequently decellularised, leaving only the ECM material. Round cover slips (15 mm Diameter, Sparks Lab Supplies) were silanised before cell seeding to enhance binding to ECM products. This was achieved by initially immersing slips in 1% acid alcohol (1% concentrated HCL, 70% ethanol, 29% dH2O) for 30 mins. Slips were washed in running water for 5min, immersed in dH2O twice for 5
min, immersed in 95% ethanol twice for 5
min and let air dry for 15
min. Cover slips were then immersed in 2% APES (3-aminopropyl triethoxysilane (A3648, Sigma) in acetone (Fisher Chemical)) for 1
min. Slips were again washed twice in dH2O for 1
min and dried overnight at 37
°C. Cells were grown to confluency on these cover slips and, following treatment, were decellularised. This was achieved by consecutive washes in Hank's Balanced Salt Solution (HBSS), 20
mM ammonium hydroxide (Sigma) with 0.05% Triton X-100, and finally HBSS again. Matrices were fixed and stained as described above with chamber slides.
Western blotting
Cells were treated with 10ng/ml MMP-3 for 24
h in serum-free media. Media supernatants were aspirated and mixed 1:6 with StrataClean resin (Agilent). After centrifugation, the supernatant was removed and the pellet was resuspended in NP-40 lysis buffer containing 50
mM Tris pH 7.5, 150
mM NaCL, 1% NP-40, 10% SDS, 1X protease inhibitor (Roche). Cells were lysed using NP-40 lysis buffer for protein collection. Samples were centrifuged at 10,000
rpm for 15
min (IEC Micromax microcentrifuge) and supernatant was retained. Protein samples were loaded onto a 10% SDS-PAGE gel at 30–50μg per well. Proteins were separated by electrophoresis over the course of 150
min at constant voltage (120 V) under reducing conditions and subsequently electro-transferred onto methanol-activated PVDF membranes at constant voltage (12 V). Gels intended for use with Collagen IV antibodies were run under native conditions. Membranes were blocked for 1 h at room temperature in 5% non-fat dry milk and incubated overnight at 4
°C with a rabbit primary antibodies to collagen IV, α-SMA, laminin and fibronectin as previously stated at concentrations of 1 in 1000 but 1 in 500 for laminin. Membrane blots were washed 3x5
min in TBS and incubated at room temperature for 2
h with horse radish peroxidase-conjugated anti-rabbit secondary antibody (Abcam). Blots were again washed and treated with a chemiluminescent substrate (WesternBright ECL, Advansta) and developed on a blot scanner (C-DiGit, LI-COR). The membranes containing cell lysate samples were re-probed with GAPDH antibody (ab9485, Abcam) for loading control normalisation. Media samples were normalised against their total protein concentration as determined by a spectrophotometer (ND-1000, NanoDrop). A total of four replicate blots were quantified for each cell lysate sample antibody, and 2–3 replicates for a media sample. Band images were quantified using Image J software. Fold change in band intensity was represented in comparison to vehicle control treatments of PBS.
AAV
AAV-2/9 containing the enhanced green fluorescent protein (eGFP) reporter gene (Vector Biolabs) was initially used to assess viral transduction and expression in the anterior chambers of wild type mice (C57/BL6). Murine MMP-3 cDNA was incorporated into Bam HI/Xhol sites of the pAAV-MCS vector (Cell Biolabs Inc) for constitutive expression of MMP-3. A null virus was used as contralateral control using the same capsid and vector. The inducible vector was designed by cloning MMP-3 cDNA into a pSingle-tTS (Clontech) vector. This vector was then digested with BsrBI and BsrGI and the fragment containing the inducible system and MMP-3 cDNA was ligated into the Not1 site of expression vector pAAV-MCS, to incorporate left and right AAV inverted terminal repeats (L-IRT and R-ITR). AAV-2/9 was generated using a triple transfection system in a stable HEK-293 cell line (Vector Biolabs). For animals injected with the inducible virus, after a 3-week incubation period, 0.2% doxycycline (D9891, Sigma) in PBS was administered twice daily to the eye for 10–16 days to induce viral expression. A similar inducible virus expressing eGFP was used as a control in the inducible study.
Intracameral injection
Animals were anaesthetised by intra-peritoneal injection of ketamine (Vetalar V, Zoetis) and domitor (SedaStart, Animalcare) (66.6 and 0.66mg/kg, respectively). Pupils were dilated using one drop of tropicamide and phenylephrine (Bausch & Lomb) on each eye. 2
μl of virus at a stock titre of 5x1013 vector genomes per ml was initially back-filled into a glass needle (ID1.0
mm, WPI) attached via tubing (ID-1.02 mm, OD-1.98 mm, Smiths) to a syringe pump (PHD Ultra, Harvard Apparatus). An additional 1
μl of air was then withdrawn into the needle. Animals were injected intracamerally just above the limbus. Viral solution was infused at a rate of 1.5
μl/min for a total of 3
μl to include the air bubble. Contralateral eyes received an equal volume and titre of either AAV-MMP-3 or AAV-Null. The air bubble prevented the reflux of virus/aqueous back through the injection site when the needle was removed. Fucidic gel (Fucithalmic Vet, Dechra) was applied topically following injection as an antibiotic agent. To counter anaesthetic, Antisedan (atipamezole hydrochloride, SedaStop, Animalcare) was intra-peritoneally injected (8.33
mg/kg) and a carbomer based moisturising gel (Vidisic, Bausch & Lomb) was applied during recovery to prevent corneal dehydration.
Immunohistochemistry (mouse eyes)
Eyes were enucleated 4 weeks post-injection of virus and fixed in 4% paraformaldehyde overnight at 4°C. The posterior segment was removed by dissection and anterior segments were washed in PBS and placed in a sucrose gradient of incrementing sucrose concentrations containing 10%, 20% and finally 30% sucrose in PBS. Anterior segments were frozen in O.C.T compound (VWR Chemicals) in an isopropanol bath immersed in liquid nitrogen and cryosectioned (CM 1900, Leica Microsystems) at 12
μm thick sections. Sections were gathered onto charged Polysine® slides (Menzel-Gläser) and blocked for 1
h with 5% normal goat serum (10658654, Fischer Scientific) and 0.1% Triton X-100 in PBS. Slides were incubated overnight at 4
°C in a humidity chamber with a 1:100 dilution of primary antibody. Antibodies used were MMP-3 (ab52915, Abcam) and GFP (Cell Signalling). Sections were washed three times in PBS for 5
min and incubated with a Cy-3 conjugated anti-rabbit IgG antibody (ab6936, Abcam) at a 1:500 dilution for 2 h at 37
°C in a humidity chamber. Slides were washed as before and counter stained with DAPI for 30 s. Slides were mounted using Aquamount (Hs-106, National Diagnostics) with coverslips (Deckgläser) and visualised using a confocal microscope (Zeiss LSM 710).
Total MMP-3 quantification
MMP-3 concentration was quantified using enzyme-linked immunosorbent assay (ELISA) kits for both human SC monolayers (DMP300, R&D Systems) and murine aqueous (RAB0368-1KT, Sigma) according to the manufacturer’s protocol. SC monolayers were cultured and treated with a 1 in 10 dilution of human cataract and POAG AH, a method previously described (87). Media was taken from the monolayers 24h post-treatment and assayed for total MMP-3.
To measure the secretion of MMP-3 by AAV-2/9 into the AH, animals were inoculated with virus as described previously via intracameral injection. Four weeks post-injection, the animals were sacrificed and AH was collected. This was achieved by the cannulation of the cornea with a pulled glass needle (1B100-6, WPI) and gentle pressing of the eye until it was deflated. Aqueous was expelled from the needle (approximately 5μl) by the attachment of a 25ml syringe connected via barb fitting and tubing (Smiths Medical) and a gradual push of the syringe plunger. Aqueous was assayed using the previously mentioned ELISA kit.
MMP-3 activity assay (FRET)
Enzymatic activity of secreted MMP-3 was quantified using fluorescence resonance energy transfer (FRET). A fluorescent peptide consisting of a donor/acceptor pair remains quenched in its intact state. This peptide contains binding sites specific to MMP-3. Once cleavage occurs through MMP-3 mediated proteolysis, fluorescence is recovered by the transfer of energy from the donor to the acceptor, resulting in an increase in the acceptor’s emission intensity. Cleavage of substrate, and therefore fluorescence, was monitored on a FLUOstar OPTIMA (BMG Labtech) over the course of 2.5h at 37
°C, to allow ample time for substrate cleavage. Media samples were collected from treated SC monolayers and combined with a 1:100 dilution of an MMP-3 specific substrate (ab112148, Abcam). Levels of active MMP-3 were interpolated from a standard curve defined by ELISA. For murine aqueous MMP-3 activity, aqueous was retrieved four weeks post-injection of AAV-MMP-3 or AAV-Null as described above. Aqueous samples were processed through an activity kit (abe3730, Source Bioscience), selected for its high sensitivity and specificity, according to the manufacturer’s protocol.
Enzymatic activity was calculated as described in MMP-3 activity Assay Kit’s (ab118972, Abcam) protocol:
Where B is the level of MMP-3 interpolated from the standard curve, T1 is the time (min) of the initial reading, T2 is the time (min) of the second reading and V is the sample volume (ml) added to the reaction well. The units ‘nmol/min/ml’ are equivalent to ‘mU/ml’.
Measurement of outflow facility
Animals were sacrificed for outflow facility measurement 4 weeks after injection of virus. Eyes were enucleated for ex vivo perfusion using the iPerfusion™ system as described in (47). Contralateral eyes were perfused simultaneously using two independent but identical iPerfusion systems. Each system comprises an automated pressure reservoir, a thermal flow sensor (SLG64-0075, Sensiron) and a wet-wet differential pressure transducer (PX409, Omegadyne), in order to apply a desired pressure, measure flow rate out of the system and measure the intraocular pressure respectively. Enucleated eyes were secured to a pedestal using a small amount of cyanoacrylate glue in a PBS bath regulated at 35°C. Perfusate was prepared (PBS including divalent cations and 5.5mM glucose) and filtered (0.2
μm, GVS Filter Technology) before use. Eyes were cannulated using a bevelled needle (NF33BV NanoFilTM, World Precision Instruments) with the aid of a stereomicroscope and micromanipulator (World Precision Instruments). Eyes were perfused for 30
min at a pressure of ~8
mmHg in order to acclimatise to the environment. Incrementing pressure steps were applied from 4.5 to 21
mmHg, while recording flow rate and pressure. Flow (Q) and pressure (P) were averaged over 4
min of steady data, and a power law model of the form
was fit to the data using weighted power law regression, yielding values of Cr, the reference facility at reference pressure Pr=
8
mmHg (corresponding to the physiological pressure drop across the outflow pathway), and β, a nonlinearity parameter characterising the pressure-dependent increase in facility observed in mouse eyes (47).
IOP
IOP measurements were performed by rebound tonometry (TonoLab, Icare) both prior to intracameral injection and 4 weeks post-injection. Readings, which were the average IOP values after five tonometric events, were taken 10min after the intra-peritoneal administration of mild general anaesthetic (53.28
mg/kg ketamine and 0.528
mg/kg domitor). Two readings were taken for one eye, then the other. This was repeated for a total of four readings per eye. Due to a minimum reading of 7
mmHg by the tonometer, a non-parametric approach was taken in the analysis of the readings. The median IOP was calculated for each eye, and MAD (median absolute deviation) values were used as a measure of dispersion. For comparing median values in a paired population, the Wilcoxon matched-pairs signed-rank test was employed to test for changes in IOP pre- and post-injection, and also for changes between contralateral eyes.
Analysis of central corneal thickness
Enucleated mouse eyes transduced with AAV-MMP-3 or its contralateral control, AAV-Null, were fixed overnight in 4% PFA and washed in PBS. Posterior segments were removed by dissection under the microscope and anterior segments were embedded in medium (Tissue-Tek OCT Compound). Serial sectioning was performed on each eye and five frozen sections (12μm) were transferred to a Polysine slide (Thermo Scientific) for staining with DAPI and mounted with aqua-polymount (Polyscience). Corneal sections were judged to be central by qualitatively taking the same distance from both iridocorneal angles. For quantitation, we measured the corneal thickness of sections on five consecutive slides by light and confocal microscopy (Zeiss LSM 710). A total of 25 measurements were taken from each eye to represent mean central corneal thickness (μm) using the NIH ImageJ software.
Transmission electron microscopy
Ultrastructural investigation was performed by transmission electron microscopy (TEM) in four pairs of mouse eyes. One eye of each pair was injected with AAV-Null, the other with AAV-MMP-3, as described above. Four weeks after injection, the eyes were enucleated and immersion fixed in Karnovsky’s fixative (2.5% PFA, 0.1M cacodylate, 2.25% glutaraldehyde and dH2O) for 1h. Eyes were then removed from fixative and the cornea pierced using a 30-gauge needle (BD Microlance 3, Becton Dickinson). Eyes were placed back into fixative overnight at 4
°C, washed 3x10
min, stored in 0.1M cacodylate and sent to Erlangen.
Here the eyes were cut meridionally through the centre of the pupil, the lens carefully removed, and the two halves of each eye embedded in Epon. Semi-thin sagittal and then ultrathin sections of Schlemm´s canal (SC) and trabecular meshwork (TM) were cut from one end of each half, and then the other approximately 0.2–0.3mm deeper. The location of the superficial and deeper cut ends was alternated for the second half of the eye such that all four regions examined were at least 0.2–0.3
mm distant from one another. The ultrathin sections contained the entire anterior posterior length of the inner wall and the TM.
In four regions of each eye, we measured the length of optically empty space immediately underlying the inner wall endothelium of SC (Supplementary Material, Fig. S6). We also measured the inner wall length in contact with ECM, including basement membrane material, elastic fibres, or amorphous material. The optically empty length divided by the total length (optically empty+
ECM lengths) was calculated and defined as the percentage of optically empty length for that region. All measurements were performed at 10,000x magnification, with each region including approximately 100 individual lengths of ECM or optically empty space. The measurements were performed by the two authors: ELD and CFK.
Statistical analysis
For TEER values, activity units (mU/ml) and concentrations (ng/ml), statistical differences were analysed by using unpaired two-tailed Student’s t-tests. Differences in Papp values (cm/s) were determined by a one way ANOVA with Tukey’s correction for multiple comparisons, where appropriate. ELISA standard curve concentrations were log-transformed and absorbance values were fitted to a sigmoidal dose response curve with variable slope for interpolation. Fold change of western blot data was log-transformed and investigated for significance using a one-sample t-test against a theoretical mean of 0. To measure MMP-3 concentration and activity in the AH of wild type (WT) mice, a paired two-tailed t-test was carried out for contralateral samples. Outflow facility was analysed using a weighted paired t-test performed in MATLAB as described in (47), incorporating both system and biological uncertainties. For IOP data, median values were obtained to reflect the non-parametric nature of the tonometer, and the Wilcoxon matched-pairs signed rank test was used to compare changes in paired populations. For morphology, the distribution of values representing the percent optically empty length was first examined using a Shapiro-Wilk and Anderson-Darling tests to detect for deviations from a normal distribution. The percent optically empty length between contralateral eyes was then analysed using a paired Student’s t-test. Statistical significance was inferred when P<
0.05 in all experimentation. Results were depicted as ‘mean, [95% Confidence Intervals]’ unless otherwise stated in the results section.
Acknowledgements
We wish to acknowledge Caroline Woods and Charles Murray for animal husbandry. We would also like to thank Elke Kretzschmar, Britta Bäckermann and Andrea Eichnorn for preparing the semi-and ultrathin sections and Marco Gößwein for his assistance in finalizing the TEM images for publication.
Conflict of Interest statement. None declared.
Funding
Research at the Ocular Genetics Unit at the University of Dublin, Trinity College was supported by the European Research Council [ERC-2012-AdG 322656-Oculus]. We also acknowledge an equipment grant from Science Foundation Ireland in support of this project [12/ERC/B2539]. Research at Imperial College London was supported by the US National Institutes of Health (EY022359 and EY019696), the UK Engineering and Physical Sciences Research Council (EP/J010499/1), and Fight for Sight UK (Ref 1385). Work at Duke University was also supported by the US National Institutes of Health (EY022359). Funding to pay the Open Access publication charges for this article was provided by the European Research Council.
References
Articles from Human Molecular Genetics are provided here courtesy of Oxford University Press
Full text links
Read article at publisher's site: https://doi.org/10.1093/hmg/ddx028
Read article for free, from open access legal sources, via Unpaywall:
https://academic.oup.com/hmg/article-pdf/26/7/1230/13729464/ddx028.pdf
Citations & impact
Impact metrics
Citations of article over time
Alternative metrics
Smart citations by scite.ai
Explore citation contexts and check if this article has been
supported or disputed.
https://scite.ai/reports/10.1093/hmg/ddx028
Article citations
Sustained and Efficient Delivery of Antivascular Endothelial Growth Factor by the Adeno-associated Virus for the Treatment of Corneal Neovascularization: An Outlook for Its Clinical Translation.
J Ophthalmol, 2024:5487973, 09 Sep 2024
Cited by: 0 articles | PMID: 39286553 | PMCID: PMC11405113
Review Free full text in Europe PMC
Integrated Bioinformatics-Based Identification and Validation of Neuroinflammation-Related Hub Genes in Primary Open-Angle Glaucoma.
Int J Mol Sci, 25(15):8193, 26 Jul 2024
Cited by: 0 articles | PMID: 39125762 | PMCID: PMC11311784
AAV-mediated gene therapies for glaucoma and uveitis: are we there yet?
Expert Rev Mol Med, 26:e9, 15 Apr 2024
Cited by: 1 article | PMID: 38618935 | PMCID: PMC11062146
Review Free full text in Europe PMC
AAV for Gene Therapy in Ocular Diseases: Progress and Prospects.
Research (Wash D C), 6:0291, 22 Dec 2023
Cited by: 1 article | PMID: 38188726 | PMCID: PMC10768554
Review Free full text in Europe PMC
Recently Approved Drugs for Lowering and Controlling Intraocular Pressure to Reduce Vision Loss in Ocular Hypertensive and Glaucoma Patients.
Pharmaceuticals (Basel), 16(6):791, 26 May 2023
Cited by: 1 article | PMID: 37375739 | PMCID: PMC10304251
Review Free full text in Europe PMC
Go to all (42) article citations
Data
Data behind the article
This data has been text mined from the article, or deposited into data resources.
BioStudies: supplemental material and supporting data
Similar Articles
To arrive at the top five similar articles we use a word-weighted algorithm to compare words from the Title and Abstract of each citation.
Open-angle glaucoma: therapeutically targeting the extracellular matrix of the conventional outflow pathway.
Expert Opin Ther Targets, 21(11):1037-1050, 05 Oct 2017
Cited by: 29 articles | PMID: 28952395
Review
Morphological and hydrodynamic correlates in monkey eyes with laser induced glaucoma.
Exp Eye Res, 89(5):748-756, 09 Jul 2009
Cited by: 29 articles | PMID: 19591828
The aqueous humor outflow pathways in glaucoma: A unifying concept of disease mechanisms and causative treatment.
Eur J Pharm Biopharm, 95(pt b):173-181, 07 May 2015
Cited by: 92 articles | PMID: 25957840
Review
Intracameral Delivery of AAV to Corneal Endothelium for Expression of Secretory Proteins.
Methods Mol Biol, 1950:263-270, 01 Jan 2019
Cited by: 3 articles | PMID: 30783979
Funding
Funders who supported this work.
Engineering and Physical Sciences Research Council (1)
Grant ID: EP/J010499/1
European Research Council (1)
A radical approach for improved glaucoma treatment. (Oculus)
Prof Peter Humphries, Trinity College
Grant ID: 322656
Fight for Sight (1)
Grant ID: 1385/86
NEI NIH HHS (2)
Grant ID: R01 EY022359
Grant ID: R01 EY019696