Abstract
Free full text

Interaction between Smad7 and β-Catenin: Importance for Transforming Growth Factor β-Induced Apoptosis†
Abstract
Members of the transforming growth factor β (TGF-β) and Wnt/wingless superfamilies regulate cell fate during development and tissue maintenance. Here we report that Smad7 interacts with β-catenin and lymphoid enhancer binding factor 1/T-cell-specific factor (LEF1/TCF), transcriptional regulators in Wnt signaling, in a TGF-β-dependent manner. Smad7 was found to be required for TGF-β1-induced accumulation of β-catenin and LEF1 in human prostate cancer (PC-3U) cells as well as in human keratinocytes (HaCaT cells). Moreover, when the endogenous Smad7 was repressed by specific small interfering RNA, TGF-β-induced increase of activated p38, Akt phosphorylated on Ser473, glycogen synthase kinase 3β phosphorylated on Ser9 was prevented, as well as the TGF-β-induced association between β-catenin and LEF1. Notably, the observed physical association of Smad7 and β-catenin was found to be important for TGF-β-induced apoptosis, since suppression of β-catenin expression by small interfering RNA decreased the apoptotic response to TGF-β.
Signaling molecules downstream of transforming growth factor β ( TGF-β) and Wnt receptors regulate both cell fate and proliferation during development and tissue homeostasis (5, 53). TGF-β exerts its cellular effects by forming a complex with its type II and type I serine/threonine kinase receptors, in which the type I receptor is phosphorylated and activated by the constitutively active type II receptor. The Smad proteins transduce signaling from the activated receptor complex to the nucleus, where they act as modulators of gene transcription. The family of Smad proteins is divided into three different groups, the receptor-activated Smads (R-Smads), the common mediator Smad (Co-Smad; Smad4), and the inhibitory Smads (I-Smads; Smad6 and Smad7). The R- and Co-Smads contain a conserved N-terminal Mad homology (MH)1 domain which is not present in I-Smads, whereas a C-terminal MH2 domain is present in all Smads. R-Smads (Smad2 and Smad3 in the TGF-β pathway) are phosphorylated in their extreme C-terminal parts by the activated type I receptor, whereby the affinity of the R-Smads for Smad4 is increased and heteromeric Smad complexes are formed and accumulate in the nucleus. Both Smad3 and Smad4 have DNA-binding motifs in their MH1 domains (25, 53).
The expression of I-Smads is induced by TGF-β and BMP (24, 30, 43). They are part of negative feedback loops by binding to the activated receptor complex and thereby competing with R-Smads. Moreover, Smad7 interacts with the ubiquitin ligases Smurf1 and Smurf2; Smad7-mediated docking of Smurfs to the receptors causes ubiquitination of the TGF-β and BMP receptors, which mark them for degradation by the proteasome pathway (17, 35). Smad7 has been found to shuttle between the nucleus and the cytoplasm in cells. The localization is regulated in a cell-type dependent manner, by stimulation of the cells with TGF-β, BMP, and the extracellular matrix proteins such as fibronectin, as well as by interaction with Smurf (17, 18, 23, 32, 35, 59). Smad7 counteracts most of the well-known TGF-β-regulated responses in cells, like growth inhibition via p21WAF1/CIP1 and production of extracellular matrix proteins (24, 46), while the expression of Smad7 is required for TGF-β-induced apoptosis in epithelial cells (16, 39).
Members of the lymphoid enhancer factor 1/T-cell factor (LEF1/TCF) family of transcription factors have together with β-catenin been demonstrated to be nuclear effectors in the Wnt signaling pathway (5). Wnt/Wingless proteins belong to a large family of secreted signaling molecules which have been shown to control embryonic patterning and cell-fate decisions during development. The subcellular localization and level of β-catenin is under strict control (47). β-Catenin is either bound to the intracellular domain of the adhesion molecule cadherin and the actin cytoskeleton at the plasma membrane, or targeted for degradation through the interaction with the adenomatous polyposis coli (APC), axin and glycogen synthase kinase 3β (GSK-3β) complex in the cytoplasm. Phosphorylation of β-catenin by GSK-3β causes degradation of β-catenin by the ubiquitin-proteasome pathway. Binding of the Wnt family ligands to the transmembrane receptor Frizzled leads to increased stability of β-catenin (56 and Pignatelli 2002), and accumulation of β-catenin in the nucleus. The nuclear accumulation has been shown to be dependent on both LEF1/TCF and an active Wnt signal, however, β-catenin lacks a canonical nuclear localization sequence (NLS). Moreover, it has been shown that increased level of β-catenin and LEF1 results in spontaneous retention of β-catenin in the nucleus (5, 26). The signals downstream of wingless (wg) and decapentaplegic (dpp, a TGF-β superfamily member), converge on the regulation of the homeotic gene Ultrabithorax (Ubx) during induction of endoderm. Recently, Smad3 and Smad4 have been shown to be directly associated with LEF1/TCFs while their association with β-catenin was found to be indirect (44). The Smad3 and Smad4 proteins have together with LEF1 synergistic effects on Xtwn promoters, in the presence of TGF-β, while β-catenin was found to promote responses on Xtwn promoters, only in the presence of both Wnt and TGF-β signals (28).
Activation of the Wnt signaling pathway has been reported to be important in colorectal cancer cells, where mutations of β-catenin or APC results in accumulation of β-catenin in the cytoplasm and in the nucleus. Interestingly, c-myc and cyclin D1 have been identified as target genes for the activated β-catenin-TCF-4 complex (5, 55), while TGF-β via Smad3 and Smad4 represses the expression of c-myc (11). Recently, Smad3 was found in a ternary complex with either LEF1 or TCF-4, and β-catenin on the human c-myc promoter. Interestingly, when LEF1 was present in the complex, cells becomes refractory to TGF-β-induced c-myc repression (51). During the last years several groups have reported that β-catenin is involved in cell cycle regulation and apoptosis in Drosophila (1, 33) and in mammalian cells (2, 21, 36, 45).
We have earlier demonstrated that Smad7, which is a direct target gene for TGF-β, is required for TGF-β-induced apoptosis in human prostate PC-3U cancer cells and in human HaCaT keratinocytes (39). Recently, we identified Smad7 as a positive regulator of the TGF-β-TAK1-MKK3-p38 mitogen-activated protein (MAP) kinase pathway leading to apoptosis in PC-3U cells (18). Moreover, overexpression of Smad7 in mink lung epithelial cells (MvLu1) and Madin-Darby canine kidney cells (MDCK) has been shown to cause activation of the SAPK/JNK pathway, leading to apoptosis (41). These reports suggest that Smad7 can positively regulate both the p38 and SAPK/JNK MAP kinase pathways downstream of the activated TGF-β receptor complex.
In the present study, we report that TGF-β promotes the physical interaction of Smad7 with β-catenin and LEF1/TCF, and accumulation of β-catenin in a p38 MAP kinase-dependent manner. Moreover, small interfering RNA (siRNA) for β-catenin abolished TGF-β-induced apoptosis in malignant and immortalized human epithelial cell lines (PC-3U and HaCaT), indicating that β-catenin is required for TGF-β-induced apoptosis. Thus, these results provide a molecular basis for the diversity of biological signaling evoked by TGF-β to determine cell fate during embryonal development and cancer.
MATERIALS AND METHODS
Cell culture.
The human prostate carcinoma cell line PC-3U, originating from PC-3 cells (16), PC-3U cells stably transfected with pRKV5 antisense Smad7 (PC-3U/AS-S7 cells), and the stably transfected pMEP4-Smad7 (PC-3U/pMEP4-Smad7) cells were routinely grown in RPMI 1640 with 10% fetal bovine serum in the presence of their specific antibiotics to maintain selection pressure, as previously described (39). PC-3U/pMEP4-Smad7 cells were stimulated with 1 μM CdCl2 for the indicated time periods to induce expression of Flag-Smad7. PC-3U cells treated with 1 μM CdCl2 for similar time periods were used as the control. COS-1, HaCaT, and HepG2 cells, originating from American Type Culture Collection, and mouse embryonic fibroblasts (MEFs), wild type and p38α−/−, a kind gift from A. R. Nebreda, were maintained in Dulbecco's modified Eagle's medium with 10% fetal bovine serum. In all assays, TGF-β1 stimulation was performed at 10 ng/ml in medium containing 1% fetal bovine serum.
Antibodies and reagents.
The following antibodies were used; mouse monoclonal anti-α-actin, β-tubulin, anti-Flag M5 and M2, anti-Myc 9E10 (Sigma Chemical Company), anti-β-catenin (BD Transduction Laboratories), anti-LEF1/TCF (Kamiya Biomedical Company), and anti-M30 (Roche). Polyclonal rabbit anti-phospho-Akt Ser473, anti-GSK-3β total and phospho-Ser9, anti-p38 total and phospho-Thr180/Tyr182, lamin A antibodies (Cell Signaling Technology), anti-Smad7 (affinity-purified KAF antiserum, described in reference 8) anti-Akt (akt1,2), and goat anti-Smad7 (Santa Cruz). TGF-β1 was from R&D Systems. Complete and Pefabloc were from Roche. The phosphatidylinositol 3-ki-nase inhibitor 2-(4-morpholinyl)-8-phenyl-4H-1-benzopyran-4-one (LY294002) and the p38 mitogen-activated protein kinase α and β inhibitor 4-(4-fluorophenyl)-2-(4-methylsulfinylphenyl)-5-(4-pyridyl)1H-imidazole (SB203580) were purchased from Calbiochem, used at a concentration of 10 μM, and added to cells 1 h before TGF-β treatment.
Western blotting, immunofluorescence, and in vitro and in vivo protein interaction assays.
Cells grown on 10-cm dishes were starved at least 12 h and then treated with TGF-β1 for the indicated times, washed once with ice-cold phosphate-buffered saline, and lysed in ice-cold lysis buffer (150 mM NaCl, 50 mM Tris [pH 8.0], 0.5% deoxycholate, 1% NP-40, 10% glycerol, 1 mM aprotinin, 1 mM Pefabloc, 1 mM phenylmethylsulfonyl fluoride). After centrifugation, supernatants were collected and protein concentrations were measured by using the Bio-Rad protein assay kit. Equal amounts of proteins were used for immunoprecipitations and subjected to sodium dodecyl sulfate gel electrophoresis in 10 or 12% polyacrylamide gels and blotted onto polyvinylidene difluoride membranes as previously described (39).
Immunofluorescence and transient transfections were performed as previously described (18, 32).
Subcellular fractionation.
Cells grown on 10-cm dishes were starved at least 12 h and then treated with TGF-β1 for the indicated times, washed twice with ice-cold phosphate-buffered saline, and scraped in ice-cold phosphate-buffered saline. Thereafter the cells were centrifuged 5 min at 500 × g at 4°C and the supernatant was discarded. The cells were resuspended in ice-cold lysis buffer containing 10 mM morpholineethanesulfonic acid (MES; pH 6.2), 10 mM NaCl, 1.5 mM MgCl2, 1 mM EDTA, 5 mM dithiothreitol, 1% Triton X-100, and protease inhibitors. After a second centrifugation step the supernatant containing the cytoplasmic part was taken away. The remaining nuclear pellet was washed once with washing buffer (same as lysis buffer but without Triton X-100), then lysed in an ice-cold extraction buffer (25 mM Tris-HCl [pH 10.5], 1 mM EDTA, 0.5 M NaCl, 5 mM β-mercaptoethanol, 0.5% Triton X-100). Both the cytoplasmic and nuclear fractions were then centrifuged at 15,000 × g for 30 min at 4°C.
Again, the supernatants were collected and protein concentrations were measured by using the Bio-Rad protein assay kit. Equal amounts of proteins were subjected to SDS-gel electrophoresis as described above. Lamin A and β-tubulin antibodies were used to verify the separation of nucleus and cytoplasmic fractions.
Plasmids and DNA transfections.
The expression vectors for HA-tagged ALK5, Myc-tagged Smad proteins, Flag-tagged Smad7, Smad7ΔN (amino acids 204 to 402), and Smad7ΔC (amino acids 2 to 261) in the mammalian expression vector pcDNA3 (Invitrogen) were kind gifts from P. ten Dijke. The corresponding glutathione S-transferase (GST)-tagged Smad7 constructs were generated by subcloning into pGEX4T-1 (Amersham-Pharmacia Biotech). Fusion constructs of the DNA-binding domain of Gal4 with wild-type Smad7 has been described previously (48a).
RNA preparation.
Twenty-one-base-pair siRNA duplexes for Smad7 and β-catenin were synthesized by Dharmacon Research (Lafayette, Colo.). The targeted sequence for Smad7 siRNA duplex (5′ AA GCU CAA UUC GGA CAA CAA G 3′) or a nonspecific duplex oligonucleotide as a negative control were used in transient transfections of cells as described below for β-catenin siRNA. The targeted sequence for β-catenin siRNA duplex (5′ AAG UCC UGU AUG AGU GGG AAC 3′) or a nonspecific duplex oligonucleotide as a negative control (5′ AAC AGU CGC GUU UGC GAC UGG3′) (12.8 μg/100-mm plate) were transfected with Lipofectamine (Invitrogen) at a ratio of 1 μg of RNA to 3 μl of Lipofectamine. TGF-β was added 24 h after transfection, and the immunofluorescence experiments were carried out after an additional 24 h.
RESULTS
Smad7 interacts with β-catenin and TCF-4 in a TGF-β-dependent manner.
The TGF-β and Wnt signaling pathways are known to act synergistically in the regulation of specific genes and β-catenin has been shown to be involved in apoptotic regulation. β-Catenin act as a coregulator for gene transcription in a complex together with LEF1/TCF transcription factors. We therefore examined whether Smad7, which mediates TGF-β-induced apoptosis, interacts with β-catenin and LEF1/TCF-4 with coimmunoprecipitation techniques. We found that endogenous β-catenin could be immunoprecipitated together with Myc-tagged Smad7 in transiently transfected COS1 cells. The interaction was enhanced by cotransfection of a constitutively active TGF-β type I receptor (ALK-5) (our unpublished data). In PC-3U cells stably transfected with pMEP4-FlagSmad7 (PC-3U/pMEP4-S7 cells), Smad7 expression is under the control of the CdCl2-inducible metallothionine promoter, Flag-Smad7 was found to interact with endogenous β-catenin (our unpublished data).
We next analyzed which domain of Smad7 that interacts with β-catenin, by transient transfections of COS1 cells with Flag-tagged wild-type Smad7 (Flag-S7 wt) and Smad7 deletion mutants (48a). We observed coimmunoprecipitation of endogenous β-catenin with wild-type Smad7 as well as with the N-terminal part of Smad7 (Flag-S7ΔC), however, the interaction between β-catenin and the C-terminal part of Smad7 (Flag-S7ΔN) was significantly weaker (Fig. (Fig.1A),1A), suggesting that β-catenin bound to the N terminus of Smad7. In order to investigate if Smad7 might act as an adaptor for β-catenin and LEF1/TCF proteins, 293T cells were transfected with wild-type Flag-S7 or Flag-S7ΔN together with either hemagglutinin (HA)-LEF1 or HA-TCF-4. We observed coimmunoprecipitation between Flag-S7C and HA-LEF1 or HA-TCF-4 (Fig. (Fig.1B1B).
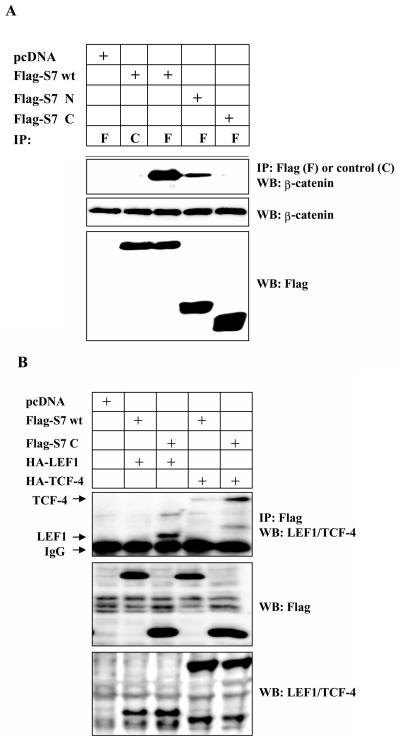
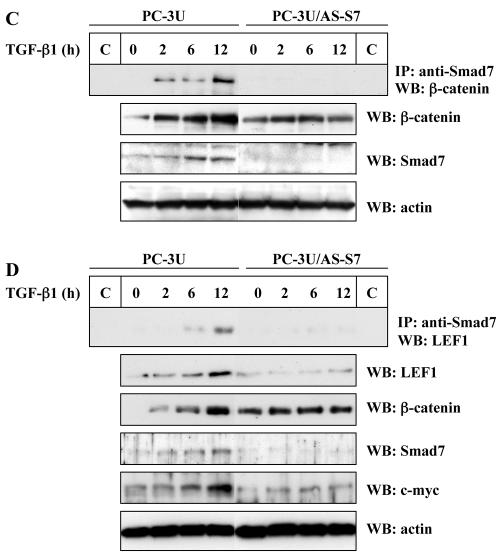
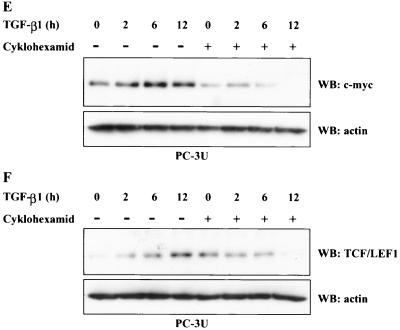
TGF-β-induced association between β-catenin, LEF1, and Smad7. (A) Coprecipitation of endogenous β-catenin with wild-type (wt) or different Flag-Smad7 deletion mutants in transiently transfected COS1 cells. Lysates of transfected cells were subjected to immunoprecipitation with Flag M2 (F) or nonimmune, control (C) antibodies and then analyzed for coprecipitated Flag-Smad7 by immunoblotting with a β-catenin antibody. (B) Coprecipitation of HA-LEF1 or HA-TCF-4 with wild-type Flag-Smad7 or a Flag-Smad7 deletion mutant (delta-N terminal; C) in transiently transfected 293T cells. Lysates of transfected cells were subjected to immunoprecipitation with polyclonal Flag antibodies and then analyzed for coprecipitated HA-LEF1/HA-TCF-4 by immunoblotting with a LEF1/TCF-4 mouse monoclonal antibody. (C and D) Coprecipitation of endogenous β-catenin and LEF1/TCF with endogenous Smad7 in PC-3U or PC-3U/AS-S7 cells treated with TGF-β for the indicated time periods. Lysates from cells were subjected to immunoprecipitation with antisera against Smad7 or control antibodies (C) and then analyzed by immunoblotting for coprecipitated β-catenin (C) or coprecipitated LEF1 (D). Immunoblots from the corresponding total cell lysate were incubated with antibodies specific for β-catenin, Smad7, LEF1/TCF-4, c-Myc, or actin. Lysates from PC-3U cells treated with TGF-β in the absence or presence of cycloheximide for the indicated time periods were investigated for the amount of endogenous c-Myc (E) or LEF1 (F). The same filters were stripped and reprobed with antibodies against actin to confirm equal loading of proteins.
Thereafter we examined if endogenous β-catenin and LEF1/TCF interacts with endogenous Smad7 in cell lysates from TGF-β1-treated PC-3U cells. When lysates from cells treated with TGF-β for different time periods were subjected to immunoprecipitation with a Smad7 antiserum followed by immunoblotting with β-catenin antibodies, a band corresponding to β-catenin was detected (Fig. (Fig.1C).1C). The interaction was clearly ligand-dependent and detected after 2 h up to 12 h of TGF-β stimulation. No coimmunoprecipitated band was observed in TGF-β-treated cells expressing antisense Smad7 (PC-3U/AS-S7) and thus having a reduced level of Smad7. Immunoblotting of whole cell lysates with Smad7 antibodies showed that TGF-β treatment increased the amount of Smad7 in PC-3U cells (Fig. (Fig.1C),1C), in line with our previous observations (18, 39).
The PC-3U/AS-S7 cells expressed significantly lower amounts of Smad7. Interestingly, the amount of β-catenin increased in PC-3U cells treated with TGF-β, compared to actin, which was used as a control for equal loading of proteins, while the amount of β-catenin was not significantly affected in PC-3U/AS-S7 cells (Fig. (Fig.1C;1C; see further below).
To investigate if endogenous Smad7 could be coimmunoprecipitated with endogenous LEF1/TCF-4 in a TGF-β-dependent manner, again PC-3U and PC-3U/AS-S7 where treated with TGF-β for different time periods as indicated. Coimmunoprecipitation was performed with a goat Smad7 antibody followed by immunoblotting with LEF1/TCF-4 mouse monoclonal antibodies (Fig. (Fig.1D).1D). As c-Myc is one of the target genes for the β-catenin LEF-1/TCF-4 complex, the amount of c-Myc in total cell lysates from the same experiment was also determined by immunoblotting. TGF-β treatment of PC-3U cells caused an increase of c-Myc after 6 h to 12 h, while this was not observed in PC-3U/AS-S7 (Fig. (Fig.1D1D).
To further investigate if the TGF-β-induced increase in endogenous c-Myc was due to an increase in protein stability or due to an increase in protein synthesis, TGF-β treatment was performed in the absence or presence of cycloheximide. When PC-3U cells were simultaneously treated with cycloheximide and TGF-β, the observed increase of c-Myc induced by TGF-β treatment were repressed (Fig. (Fig.1E).1E). As LEF1 has been shown to be positively regulated by β-catenin and TCF complexes (2), the same cell lysates were analyzed by immunoblotting for the expression of endogenous LEF1. We observed an increase of LEF1 in TGF-β treated PC-3U cells, which was repressed by simultaneous treatment with cycloheximide (Fig. (Fig.1F).1F). The results obtained indicate that the increase of c-Myc and LEF1 after TGF-β treatment is an effect of increased protein synthesis.
In conclusion, our data suggest that TGF-β treatment leads to a complex consisting of Smad7, β-catenin, and LEF1. The observed protein complex might increase the transcription of c-Myc and LEF1.
Expression of Smad7 facilitates the TGF-β-induced β-catenin and LEF1 complex.
To confirm the importance of Smad7 for the observed effects of TGF-β on β-catenin which resulted in increased amount of endogenous c-Myc, we next investigated the possibility that Smad7 is required for the TGF-β-induced activation of the β-catenin-LEF1 complex, we transiently transfected PC-3U and HaCaT cells with control or specific siRNA for Smad7, treated cells with TGF-β for different time periods and conducted coimmunoprecipitations with a polyclonal β-catenin antibody and immunoblotted for endogenous LEF1. We observed a TGF-β-induced β-catenin-LEF1 complex in both cell lines transfected with control siRNA, while it was not present in cells transfected with specific siRNA for Smad7 (Fig. (Fig.2A).2A). The functionality of the TGF-β-induced β-catenin-Smad7-LEF1 complex was shown by the lack of TGF-β induced increase of c-Myc in Smad7 siRNA-transfected cells compared to control siRNA-transfected cells (Fig. (Fig.2B).2B). Thus, our results suggest that the presence of Smad7 might contribute to the TGF-β-induced interaction between β-catenin and LEF1.
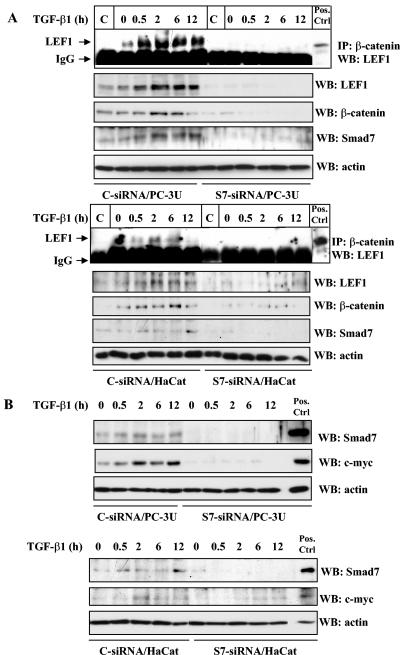
Smad7 is required for TGF-β-induced association between β-catenin and LEF1. (A) Lysates from PC-3U (upper panel) and HaCaT cells (lower panel), transiently transfected with control (C-siRNA) or specific Smad7 siRNAs (S7-siRNA), were treated or not with TGF-β for the indicated time periods and the total cell lysates were subjected to coimmunoprecipitation with a polyclonal antibody for β-catenin or a nonimmune, control (C) antibody and then analyzed for coprecipitated LEF1. Cell lysates from 293T cells transiently transfected with HA-LEF1 were used as a positive control (Pos.Ctrl.) to verify the identity of endogenous LEF1. The cell lysates were investigated for the amount of endogenous LEF1, β-catenin, and Smad7. The filters were then stripped, blocked, and reprobed with actin antibodies to confirm equal loading of proteins. (B) The same cell lysates from PC-3U and HaCaT cells were also investigated for the amount of endogenous Smad7 and c-Myc by immunoblotting. Total cell lysates from cells ectopically expressing Flag-Smad7 (PC-3U/pMEP4-Flag-S7) were used as positive control for Smad7 (Pos. Ctrl). The filters then were stripped, blocked, and reprobed with actin antibodies to confirm equal loading of proteins.
One of the target genes for Wnt signaling is c-myc (5), a proto-oncogene implicated in cell proliferation as well as apoptosis (19). We therefore investigated whether Smad7 could activate the c-myc promoter, using a luciferase reporter construct containing nucleotides −2329 to +510 of the human c-myc promoter in a pGL3-basic vector pHx-luc, in transiently transfected PC-3U cells. Smad7 significantly increased (three- to fivefold) the luciferase activity compared to mock-transfected cells (see Fig. S1a in the supplemental material). As expected, transfection of β-catenin also potently increased the luciferase activity. Notably, the basal activity of the pHx-luc reporter was lower in PC-3U/AS-S7 cells than parental PC-3U cells, while serum stimulation significantly enhanced the luciferase activity (our unpublished data). Together, our results suggest that Smad7 has a positive effect on the c-myc promoter activity. However, careful examinations on the effects of Smad7 alone or in combination with β-catenin and LEF1/TCF-4 are required before the data can be validated.
Thereafter we examined the levels of endogenous β-catenin and c-Myc in the stably transfected pMEP4-Smad7 (PC-3U/pMEP4-Smad7) cells and used PC-3U cells as the control. Cell lysates from CdCl2-treated PC-3U and PC-3U/pMEP4-Smad7 cells were subjected to immunoblotting with antibodies against β-catenin, c-Myc and Smad7. We observed increased levels of endogenous β-catenin and c-Myc in PC-3U/pMEP4-Smad7 cells compared to PC-3U cells. We have previously observed high levels of Smad7 in the absence of CdCl2 treatment which is probably due to a leaky promoter and therefore decided to use siRNA for Smad7 in the following experiments (see Fig. S1b in the supplemented material).
In order to investigate the functional consequences of the observed interaction of Smad7 with LEF1/TCF and β-catenin, TCF-4, β-catenin, and a luciferase reporter construct consisting of five Gal4 DNA-binding sites fused to Smad7 (Gal4-Smad7) were cotransfected into a human hepatocyte (HepG2) cell line. β-Catenin alone or TCF-4 and β-catenin together were found to activate the Gal4-Smad7 luciferase reporter. These data therefore suggest that β-catenin and TCF-4 regulate transcription together with Smad7, forced to bind to DNA (see Fig. S2 in the supplemental material).
To further investigate whether Smad7 cooperates with LEF1/TCF and β-catenin in transcriptional regulation, we also examined the effects of Smad7 on a synthetic TCF-4 promoter (TOPFLASH). As expected, TCF-4 and β-catenin together increased the luciferase reporter activity of TOPFLASH in PC-3U cells four- to fivefold compared to mock transfections (pcDNA3). Transfection of Smad7 alone had no effect, however, transfection of Smad7 together with TCF-4 and β-catenin resulted in an additional increase of the luciferase reporter activity; no activation was seen in cells transfected with the control reporter FOPFLASH (see Fig. S3 in the supplemental material). TGF-β treatment for 16 h caused a decrease of the luciferase-activity (data not shown), in line with previous reports (27). Thus, our data suggests that Smad7 enhances the effects of β-catenin on a synthetic TCF-4 responsive promoter in PC-3U cells.
Smad7 colocalizes with β-catenin after TGF-β treatment and is required to promote accumulation of β-catenin.
β-Catenin is a multifunctional protein and its level, as well as localization, are important for the function of the protein. Following our demonstration of a physical interaction between β-catenin and Smad7, we next investigated the localization of the TGF-β-induced Smad7-β-catenin complex, using immunofluorescence staining in PC-3U and PC-3U/AS-S7 cells treated with TGF-β for 2 to 12 h. We found that endogenous Smad7 was localized both in the nucleus and in the cytoplasm of PC-3U cells in nontreated cells, while β-catenin was mainly localized at cell-cell contacts. After TGF-β treatment, a portion of Smad7 was found to be colocalized with β-catenin mainly in the perinuclear compartment. Some β-catenin was also colocalized with Smad7 in the nucleus (Fig. (Fig.3A3A).
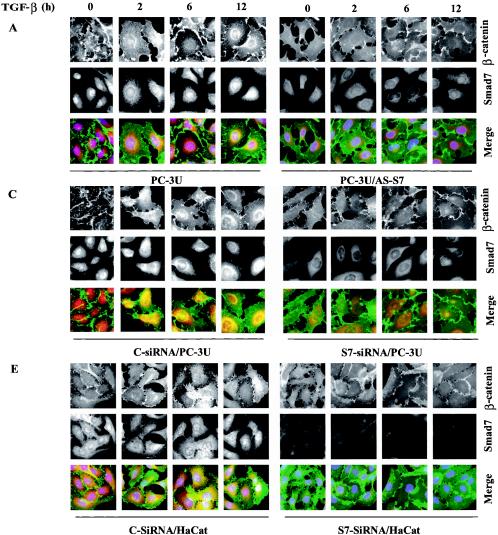
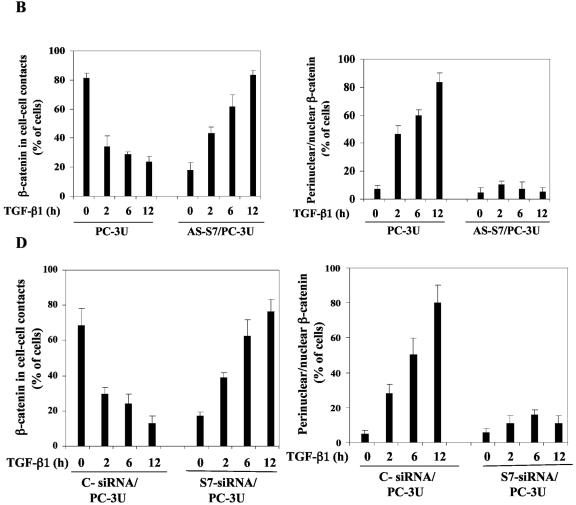
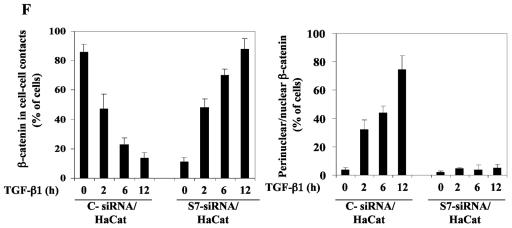
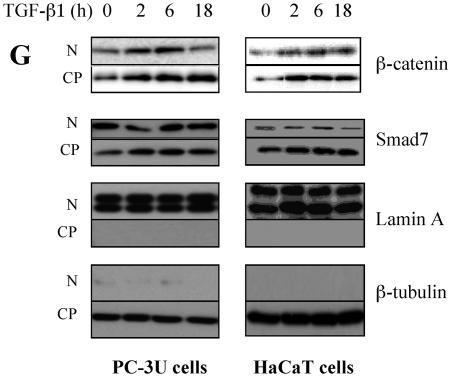
Smad7 is required for the TGF-β-induced redistribution of β-catenin to the perinuclear-nuclear compartment. (A) Subcellular localization of endogenous Smad7 and β-catenin in PC-3U or PC-3U/AS-S7 cells after treatment with TGF-β for 2 h, 6 h, or 12 h as investigated by immunofluorescence with antibodies against Smad7 and β-catenin, together with additional staining of the nuclei by DAPI. Note that β-catenin is localized in the cytoplasm and in cell-cell contacts in untreated cells. After 2 h of TGF-β treatment, a portion of Smad7 is perinuclear or nuclear as β-catenin. At longer time points (12 h) after TGF-β treatment, both proteins predominantly localize in the perinuclear and nuclear compartment (left panel). In contrast, in PC-3U/AS-S7 cells, where the levels of endogenous Smad7 is reduced, no significant change of the subcellular localization of β-catenin was observed (right panel). (B) Quantification of the effect of TGF-β treatment on the subcellular localization of endogenous β-catenin in PC-3U and PC-3U/AS-S7 cells is shown in Fig. Fig.2A.2A. (C) PC-3U cells were transiently transfected with control (C) or specific Smad7 (S7) siRNAs and treated or not with TGF-β for the indicated times. The subcellular localizations of β-catenin and Smad7 were analyzed as described above. The values for the quantification of the subcellular localization of β-catenin in the transfected cells are shown in panel D. (F) HaCaT cells were transiently transfected with control (C) or specific Smad7 (S7) siRNAs and treated or not for the indicated time periods. The distribution of endogenous β-catenin and Smad7 was investigated by immunofluorescence and shown in panel E. The quantification of the differences in distribution of β-catenin is shown in panel F. Approximately 300 cells from each condition were counted under the microscope at 40× magnification. (G) Immunoblots from subcellular fractions of PC-3U and HaCat; nucleus (N) and cytoplasm (CP), derived from cells treated or not with TGF-β for the indicated time periods. Lamin A and β-tubulin were used as markers for the nucleus and cytoplasmic fractions, respectively. Total cell lysate from cells ectopically expressing Flag-Smad7 (PC-3U/pMEP4-Flag-S7) were used as the positive control for Smad7.
The TGF-β-induced alterations of the subcellular localization for β-catenin was associated with the amount of Smad7 present in the cells as this event was not observed in PC-3U/AS-S7 cells, where the endogenous levels of Smad7 is repressed (Fig. (Fig.3A).3A). A majority of PC-3U cells showed a redistribution of β-catenin from cell-cell contacts to the perinuclear-nuclear area after 12 h of treatment (Fig. (Fig.3B).3B). In contrast, TGF-β treatment of PC-3U/AS-S7 cells caused an accumulation of β-catenin in cell-cell contacts over time (Fig. 3A and B). We have investigated by immunofluorescence stainings the localization of LEF1/TCF in PC-3U cells and found a specific granular staining predominantly in the nucleus which was not significantly affected by TGF-β treatment (our unpublished data).
To further validate our observation that Smad7 is important for TGF-β-induced redistribution from cell-cell contacts to the perinuclear/nuclear area, PC-3U and HaCaT cells were transiently transfected with control and specific siRNA for Smad7 and were treated or not with TGF-β for different time-periods. We observed in both cell lines a TGF-β-induced redistribution of β-catenin in cells transfected with control siRNA but not in cells transfected with specific Smad7 siRNA (Fig. 3C and E). The TGF-β-induced redistribution of β-catenin was counted in the microscope and the number of cells with β-catenin either in cell-cell contacts or in perinuclear/nuclear areas are shown in Fig. 3D and F. In order to further validate our observation of a TGF-β- and Smad7-dependent accumulation and redistribution of β-catenin, we performed subcellular fractionation analysis of TGF-β-treated PC-3U and HaCaT cells. We observed that the accumulation of β-catenin occurred both in the cytoplasm and in the nucleus after TGF-β treatment of PC-3U and HaCaT cells. Smad7 was found both in the cytoplasmic and nuclear fraction (Fig. (Fig.3G).3G). Thus, our data show that TGF-β treatment leads to an alteration in the localization of β-catenin in two different cell lines and that Smad7 is required for this event.
As the Smad3/Smad4 complex previously has been reported to synergize with β-catenin and LEF1/TCF in the activation of the Xtwn promoter (27), we investigated by coimmunofluorescence the possible colocalization of endogenous β-catenin and phospho-Smad3 in PC-3U and PC-3U/AS-S7 cells at different times after TGF-β treatment. We observed nuclear accumulation of phospho-Smad3 in both cell lines, while the TGF-β-induced redistribution of β-catenin from cell-cell contacts to the perinuclear/nuclear compartment occurred only in PC-3U cells. Interestingly, we observed a colocalization of phosho-Smad3 and β-catenin in the nucleus of PC-3U but not PC-3U/AS-S7 cells as shown in see Fig. S4a in the supplemental material.
Smad7 has been shown to exert a negative feed-back inhibition on TGF-β signaling (24, 43). However, we did not observe any significant differences for the TGF-β-induced phosphorylation of Smad3, in PC-3U, PC-3U/AS-S7 and PC-3U cells transiently transfected with control or specific siRNA for Smad7, after treatment with TGF-β as shown in Supplementary Fig. S4b.
Furthermore, we found that, in addition to TGF-β stimulation, Smad7 overexpression in PC-3U/pMEP4-S7 cells, induced by CdCl2 treatment, caused a nuclear accumulation of β-catenin. In CdCl2-treated PC-3U/pMEP4-S7 cells, Flag-Smad7 colocalized with endogenous β-catenin in the nucleus (see Fig. S5 in the supplemental material).
We also observed a decrease of the staining for E-cadherin in PC-3U cells stimulated with TGF-β (data not shown) and in PC-3U/pMEP4-S7 cells upon overexpression of Smad7; in contrast, an accumulation of E-cadherin at cell-cell contacts in PC-3U/AS-S7 cells with reduced levels of Smad7 occurred in response to TGF-β stimulation (our unpublished data). Thus, Smad7 appears to cause a redistribution of β-catenin from the plasma membrane to the nucleus. In two other cell lines stably transfected with pMEP-4 Flag-Smad7, i.e., porcine aortic endothelial cells and MvLu1 cells, we also observed a nuclear accumulation of β-catenin, which colocalized with Smad7 (our unpublished data). Our data propose that Smad7 plays an important role for the TGF-β-induced redistribution of β-catenin from cell-cell contacts to the perinuclear/nuclear compartment in immortalized epithelial cells and prostate cancer cells.
TGF-β- and Smad7-induced accumulation of β-catenin is p38 MAP kinase dependent.
The TGF-β pathway, via TGF-β-associated kinase 1 (TAK-1), is known to converge with the Wnt signaling pathway on specific promoters like X-Twin in Xenopus and siamos in Drosophila (6). Recently, we found that Smad7 is required for activation of the TGF-β-TAK1-MKK3-p38 MAP kinase pathway in PC-3U cells, resulting in apoptosis. Smad7 can interact with TAK1, MKK3, as well as with p38, suggesting that it acts as a scaffolding protein (18). Therefore, we explored if activation of p38 is connected to the observed Smad7-dependent accumulation of β-catenin and subsequent apoptosis.
To confirm our previous observation that Smad7 is required for TGF-β-induced phosphorylation of p38, total cell lysates isolated from TGF-β-treated PC-3U and PC-3U/AS-S7 cells were subjected to immunoblotting. TGF-β-induced phosphorylation of p38 was observed in PC-3U cells but not in PC-3U/AS-S7 cells (data not shown), thus in line with our previous report (18). In order to further validate this finding, PC-3U cells were transiently transfected with control or specific siRNA for Smad7 and treated or not with TGF-β for 30 min up to 12 h. Notably, specific siRNA for Smad7 was found to repress TGF-β-induced phosphorylation of p38 (Fig. (Fig.4A).4A). Interestingly, the selective p38 inhibitor SB203580 prevented TGF-β- and Smad7-induced accumulation of β-catenin in the perinuclear area and in the nucleus of PC-3U cells (Fig. (Fig.4B),4B), suggesting that the p38 MAP kinase pathway is important for accumulation of β-catenin in the nucleus. In PC-3U cells transiently transfected with a dominant negative (DN) Flag-p38, we observed that TGF-β did not cause nuclear translocation of β-catenin to the nucleus (see Fig. S7 in the supplemental material).
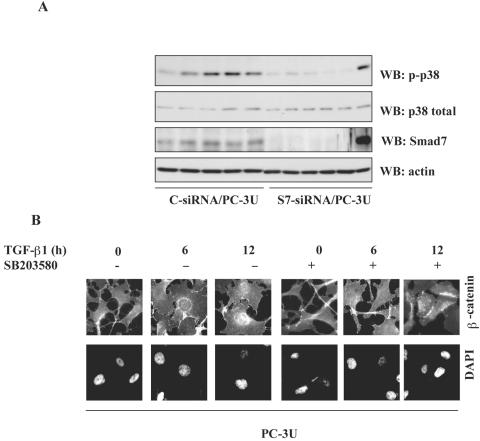
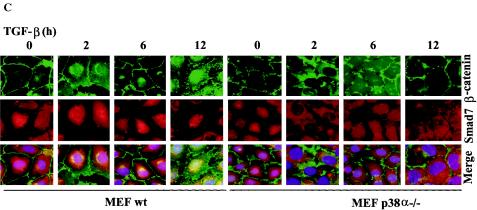
Smad7 is required for TGF-β-induced phosphorylation of p38 and the TGF-β-induced nuclear accumulation of β-catenin is associated with p38. (A) Lysates from PC-3U cells transiently transfected with control siRNA (C-siRNA) or specific Smad7 siRNA (S7-siRNA) were treated or not with TGF-β for the indicated time periods and the total cell lysates were investigated for the amount of endogenous p-p38 and Smad7. The filters were then stripped and reprobed with antibodies against total p38 to show the specificity of the band for p38 and again stripped and reprobed with actin antibodies to confirm equal loading of proteins. Total cell lysates from cells ectopically expressing Flag-Smad7 (PC-3U/pMEP4S-7) were used as the positive control for Smad7 (Ctrl). (B) The subcellular localization of β-catenin in PC-3U cells treated with TGF-β in the absence or presence of 10 μM SB203580, the p38 inhibitor, for the indicated time periods were analyzed by immunofluorescence staining. Note the absence of TGF-β-induced redistribution in cells simultaneously treated with SB203580. SB203580 was added to cells 1 h before the initiation of the experiment. Nuclei were counterstained with DAPI. (C) Mouse embryonic fibroblasts derived from wild-type (wt) or p38α−/− knockout mice were treated with TGF-β for the indicated time periods and subjected to immunofluorescence staining for endogenous β-catenin and Smad7. Nuclei were counterstained with DAPI. Note the lack of TGF-β-induced effects on β-catenin and Smad7 colocalization in p38α−/− mouse embryonic fibroblasts compared to wild-type mouse embryonic fibroblasts.
In order to verify the importance of p38 for the TGF-β-induced translocation of β-catenin, we investigated the effects of TGF-β treatment in mouse embryonic fibroblasts (MEFs) derived from p38α knockout mice (48). Interestingly, no TGF-β-induced Smad7 and β-catenin colocalization was observed in p38α−/− MEFs when compared to wild-type MEFs (Fig. (Fig.4C).4C). Thus, our data shows that TGF-β-induced redistribution of β-catenin is facilitated by p38α.
TGF-β treatment leads to accumulation of β-catenin and phosphorylation of GSK-3β in a Smad7-, p38 MAP kinase-, and phosphatidylinositol 3-kinase-dependent manner.
The activity of GSK-3β is regulated by several growth factors and when GSK-3β is phosphorylated on Ser9 the kinase is not able to phosphorylate its downstream substrates such as β-catenin (16a). Interestingly, p38 MAP kinase has previously been reported to phosphorylate Akt on Thr308 and Ser473 in human neutrophils (49). Since GSK-3β is a known substrate for Akt (9), we examined whether TGF-β could induce phosphorylation of GSK-3β on Ser9 which then would lead to its inactivation. As shown in Fig. Fig.5A,5A, TGF-β treatment of PC-3U cells led to an increased phosphorylation of GSK-3β, which was seen after 30 min of TGF-β stimulation and remained detectable up to 48 h. The amount of β-catenin detected in total cell lysates corresponded to the observed inactivation of GSK-3β. Notably, the level of Smad7 corresponded well with the increased levels of β-catenin.
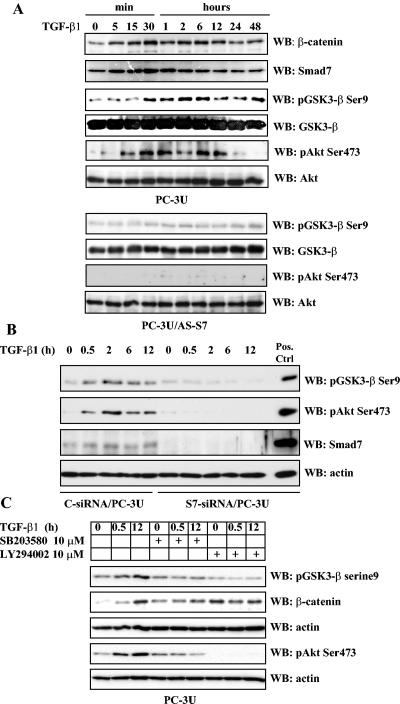
TGF-β regulates the levels of β-catenin in PC-3U cells via phosphorylation of GSK-3β on Ser9 and Akt on Ser473 in a Smad7-, p38 MAP kinase-, and phosphatidylinositol 3-kinase-dependent manner. Total cell lysates were prepared from PC-3U and PC-3U/AS-S7 cells, treated or not with TGF-β, and used for immunoblotting. (A) Levels of β-catenin and Smad7 and of GSK-3β and Akt phosphorylated at Ser9 and Ser473, respectively, in PC-3U cells treated with TGF-β for different time periods. In the upper and lower panels, the phosphorylated and nonphosphorylated forms, respectively, of GSK-3β and Akt, are shown. A similar analysis with PC-3U/AS-S7 cells treated with TGF-β is also shown. Equal amounts of proteins were loaded for each cell line. The filters were subjected to immunoblotting simultaneously under equal conditions. (B) Lysates from PC-3U cells transiently transfected with control (C-siRNA) or specific Smad7 siRNAs (S7-siRNA) were treated or not with TGF-β for the indicated time periods, and the total cell lysates were investigated for the amount of endogenous pGSK-3β Ser9, phospho-Akt Ser473, and Smad7. The filters were then stripped and reprobed with actin antibodies to confirm equal loading of proteins. Total cell lysate from cells ectopically expressing Flag-Smad7 (PC-3U/pMEP4S-7) were used as the positive control for Smad7 (Pos. Ctrl). (C) Effects of the p38 inhibitor SB203580 and the phosphatidylinositol 3-kinase inhibitor LY294002 on TGF-β-induced phosphorylation of GSK-3β on Ser9. Total cell lysates were prepared from PC-3U cells treated or not with TGF-β, in the presence or absence of SB203580 and LY294002, andsubjected to immunoblotting. The phosphorylation of GSK-3β on Ser9 and amount of β-catenin are shown in the upper two panels, and the phosphorylation of Akt on Ser473 is shown in the lower panel. Both filters were stripped, blocked, and reprobed with actin antibodies, which served as the control for equal loading of proteins.
Next, we examined the importance of Smad7 for TGF-β-induced phosphorylation of Akt by immunoblotting of total cell lysates obtained from TGF-β-treated PC-3U or PC-3U/AS-S7 cells. As shown in Fig. Fig.5A,5A, TGF-β-induced phosphorylation of Akt on Ser473 was seen in PC-3U cells but not in PC-3U/AS-S7 cells, suggesting that the phosphorylation requires Smad7. To confirm the importance of Smad7 as a regulator of phosphorylation of Akt on Ser473 and GSK3-β on Ser9, total cell lysates from PC-3U cells transiently transfected with control or specific siRNA for Smad7 was investigated by immunoblotting. Notably, reduction of Smad7 protein by siRNA reduced phosphorylation of Ser473 of Akt and Ser9 of GSK-3β, showing that Smad7 is crucial for the TGF-β-induced effects on Akt and GSK-3β (Fig. (Fig.5B5B).
The roles of p38 MAP kinase and phosphatidylinositol 3-kinase in the phosphorylation on Ser9 of GSK-3β, were further investigated using specific inhibitors. Cell lysates from PC-3U cells treated with TGF-β in the absence or presence of the selective inhibitors for p38 and PI3 kinase (SB203580 and LY294002, respectively) were immunoblotted with phospho-GSK-3β (Ser9) antibodies. TGF-β treatment led to phosphorylation of GSK-3β on Ser9, which was partially prevented by SB203580, while LY294002 almost totally prevented its phosphorylation (Fig. (Fig.5C).5C). The same filter was stripped and reprobed with β-catenin antibodies and again the TGF-β-induced accumulation of β-catenin was observed. From these results we conclude that TGF-β treatment leads to phosphorylation of GSK-3β on Ser9, in a Smad7-, p38 MAP kinase-, and phosphatidylinositol 3-kinase-dependent manner, which correlates to the accumulation of β-catenin.
Suppression of β-catenin by siRNA decreases TGF-β-induced apoptosis.
We have previously shown that TGF-β induces apoptosis of epithelial cells in a Smad7- and p38-dependent manner (18, 39). We also observed that overexpression of Smad7 in PC-3U cells induced nuclear accumulation of β-catenin (see Fig. S6 in the supplemental material). TGF-β stimulated PC-3U cells as well as PC-3U/pMEP4-S7 cells with high amounts of nuclear β-catenin showed morphological signs of apoptosis observed as condensation of nucleus and shrinkage of the cytoplasm. In contrast, the PC-3U/AS-S7 cells were protected from TGF-β-induced apoptosis, as we have earlier reported (17, 39). As previously reported we observed a reduction of the number of cell undergoing apoptosis in the presence of the p38 inhibitor SB203580 (see Fig. S6 in the supplemental material and data not shown). Since we observed that TGF-β-induced accumulation in the cytoplasm and nucleus of β-catenin correlated with apoptosis of PC-3U cells, we investigated whether a reduction of the amount of β-catenin by siRNA affected the apoptotic response induced by TGF-β.
PC-3U cells transfected with specific siRNA for β-catenin showed a decreased amount of β-catenin (Fig. (Fig.6A),6A), and were protected against TGF-β-induced apoptosis, as demonstrated by staining with the M30 antibody, which specifically recognizes caspase-cleaved cytokeratin 18, and of the nucleus by 4′,6′-diamidino-2-phenylindole (DAPI) (only 4.6% apoptotic cell [Fig. [Fig.6A;6A; Table Table1]).1]). In contrast, control cells and cells transfected with unspecific siRNA had normal levels of β-catenin and underwent TGF-β-induced apoptosis (14.5 and 16% apoptotic nuclei, respectively [Fig. [Fig.6A]).6A]). In order to investigate whether β-catenin is involved in TGF-β-induced apoptosis in another epithelial cell line, the siRNA technique was also utilized in HaCaT human keratinocyte cells. Again, we observed that nontransfected HaCaT or HaCaT cells transfected with control siRNA underwent TGF-β-induced apoptosis (13.7 and 9.2% apoptotic cells, respectively [Table [Table1]),1]), while cells transfected with specific siRNA were protected against TGF-β-induced apoptosis (1% apoptotic cells [Table [Table1]).1]). The amount of β-catenin protein in PC-3U and HaCat cells transiently transfected with specific siRNA for β-catenin was decreased compared to cells transfected with control siRNA as determined by immunoblotting (Fig. (Fig.6B6B).
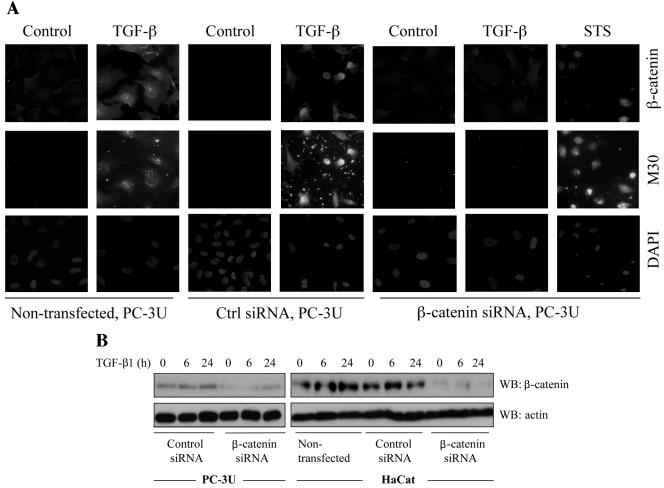
Transfection of siRNA for β-catenin reduces TGF-β induced apoptosis. (A) PC-3U and HaCaT cells were transfected or not with specific or unspecific (ctrl) siRNA for β-catenin and treated with TGF-β for 24 h. The localization and amount of β-catenin and number of apoptotic cells, shown by M30 staining, were examined by immunofluorescence. Nuclei were counterstained with DAPI. PC-3U cells transfected with specific siRNA for β-catenin and treated with 1 μM staurosporine (STS) for 8 h served as the control. The number of apoptotic cells was determined in triplicate samples in three different experiments, and at least 1,000 nuclei/sample were counted. The mean values are given in Table Table1.1. Note the correlation between M30 staining and high levels of β-catenin. (B) Lysates from PC-3U and HaCaT cells transiently transfected with control (Ctrl) siRNA or specific β-catenin siRNA were treated or not with TGF-β for the indicated time periods, and the total cell lysates were investigated for the amount of endogenous β-catenin. The filters were then stripped and reprobed with actin antibodies to confirm equal loading of proteins.
TABLE 1.
Effects of β-catenin siRNA on TGF-β-induced apoptosisa
Cell line | % Apoptotic cells
| |||
---|---|---|---|---|
Control | +TGF-β1 | +TGF-β1 + control siRNA | +TGF-β1 + β-catenin siRNA | |
PC-3U | 1.0 | 14.5 | 16.0 | 4.6 |
HaCaT | 1.0 | 13.7 | 9.2 | 1.0 |
Our data suggest that β-catenin is necessary for TGF-β-induced apoptosis in epithelial cells.
DISCUSSION
TGF-β and Wnt proteins cooperate in tissue specification during development. Smad proteins, β-catenin and LEF1/TCF can act as transcriptional regulators in the TGF-β or Wnt signaling cascade, regulating specific gene expression depending on the cellular context. Here we report on the physical association and functional cooperation between Smad7 and β-catenin in the regulation of apoptosis in prostate cancer cells. We show that TGF-β treatment of PC-3U and HaCaT cells lead to an increase of the levels of β-catenin and LEF1 in a Smad7-dependent manner and to a TGF-β-dependent association of Smad7 with β-catenin and LEF1. Our data also show that Smad7 is required for the TGF-β induced phosphorylation of p38, GSK-3β on Ser9, and Akt on Ser 473, which correlates with the observed TGF-β-induced accumulation of β-catenin. Smad7, β-catenin, and TCF-4 had synergistic effects on the synthetic TCF-4 promoter TOPFLASH, and β-catenin and LEF1/TCF enhanced transcription by a Gal4-Smad7 fusion protein. These observations suggest that Smad7 can affect gene regulation. Interestingly, PC-3U and HaCaT cells transiently transfected with β-catenin siRNA were protected against apoptosis induced by TGF-β, demonstrating a functional cooperation of Smad7 and β-catenin in this pathway. Our current data are summarized schematically in Fig. Fig.77.
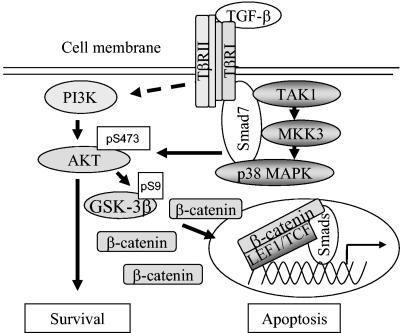
Schematic illustration of the molecular mechanisms involved in the TGF-β-Smad7 signaling pathway resulting in activation of Akt and p38, followed by phosphorylation and inactivation of GSK-3β. The inactivated GSK-3β thus cannot phosphorylate and cause degradation of β-catenin, which leads to its accumulation. The figure summarizes the result of the current study and of a previously published study (18). For further explanation and details, see the text.
Smad3 and Smad4 have previously been found to be directly associated with LEF1, and TGF-β has been shown to enhance the interaction between Smad3 and LEF1 in HEK 293T cells and to promote their activity on a Xtwn promoter. Furthermore, putative Smad4 binding sites were identified in the Xtwn promoter (27, 44). The association between Smad3, Smad4, and β-catenin was found to be indirect, while colocalization of endogenous β-catenin and Smad4 was demonstrated in the nucleus of Xenopus animal cap cells, injected with Xwnt mRNA (44). We report here that TGF-β treatment leads to an interaction between endogenous β-catenin, LEF1 and endogenous Smad7 in prostate cancer cells. Our results suggest that Smad7 is required for the TGF-β-induced interaction between β-catenin and LEF1, as we observed in two cell lines that repression of Smad7 by siRNA prevents the TGF-β-induced assembly of the β-catenin-LEF1 complex.
Interestingly, the interaction between Smad7 and β-catenin occurred primarily via the N-terminal part of Smad7. This finding is notable since the N-terminal part of Smad7 is not conserved in the R-Smads and Smad4. In contrast, the C-terminal MH2 domain of Smad7 was found to interact with LEF1 and TCF-4. Moreover, the interactions were stronger with the MH2 domain only than with wild-type Smad7, suggesting that wild-type Smad7 can occur in a latent conformation. We examined whether the colocalization of phospho-Smad3 and β-catenin in the nucleus was affected by Smad7 and we observed colocalization of the proteins in the nucleus of TGF-β-treated PC-3U cells but not in PC-3U/AS-S7 cells with a small amount of Smad7 (see Fig. S4 in the supplemental material). This finding suggests that Smad7 is required for the TGF-β-induced accumulation of β-catenin and its cooperation with the Smad3-Smad4 complex. Our findings thus suggest that the specific effects of β-catenin on different promoters are moderated by alterations in the nuclear amounts of the β-catenin-LEF1/TCF complex as well of R-Smads, Co-Smads, and I-Smads and their transcriptional coregulators or corepressors.
We have earlier reported that expression of Smad7 is required for TGF-β-induced activation of the TAK1-MKK3-p38 MAP kinase pathway and subsequent apoptosis of prostate cancer cells (18). In the current study, our data indicate that both Smad7 and p38 MAP kinase positively contributed to the accumulation of β-catenin and that high levels of Smad7 cause an increase of c-myc expression. Our preliminary data suggest that Smad7 has positive effects on a full-length human c-Myc promoter (see Fig. S1 in the supplemental material). However, future studies are required to more precisely investigate the effects of Smad7 alone or in combination with β-catenin and LEF1 on the c-myc promoter. In addition, the identification of a specific responsive site in the promoter is needed for a proper evaluation of the putative effects of Smad7 on c-Myc.
c-Myc has been shown to potently induce apoptosis, especially under conditions of stress, genotoxic damage or depletion of survival factors (19); therefore it is possible that β-catenin-mediated induction of c-myc contributes to Smad7-induced apoptosis. TGF-β is known to repress c-myc in a Smad3-dependent manner (11, 52, 57). Smad3 is present in a cytoplasmic complex consisting of the transcription factors E2F4/5 and DP1 and the corepressor p107. In response to TGF-β, this complex associates with Smad4, recognizing a composite Smad-E2F site on c-myc for repression (11, 53). Our data propose that Smad7 has a positive effect on the expression of c-Myc, which could be explained by cooperation with β-catenin and LEF1 on the c-myc promoter. This possibility requires future studies. Alternatively, Smad7-induced activation of the p38 MAP kinase pathway could cause activation of the c-myc promoter.
Another possible explanation for the increase in c-Myc expression might be that Smad7, in a TGF-β- and p38 MAP kinase-dependent manner, regulates the localization and levels of β-catenin, through their effect on GSK-3β, as discussed below. Our finding that Smad7, together with β-catenin and LEF1/TCF, induces an increase of c-myc expression suggests that Smad7 acts as a molecular switch downstream of the activated TGF-β-receptor complex to coordinate responses from both Wnt and TGF-β signals, or alternatively, that TGF-β via Smad7 regulates the localization and activity of β-catenin independently from the canonical Wnt signaling pathway. Oncoproteins like Myc and E2F1 regulate G1 progression and are also potent inducers of apoptosis by their promotion of release of cytochrome c from mitochondria. The release of cytochrome c is prevented by survival signals (34, 46a). In this respect, it is interesting that both Smad7 and β-catenin have been shown to inhibit NF-κB activity (3, 15). Future investigations will more precisely describe the mechanism for the observed positive regulation of Smad7 and p38 MAP kinase on c-Myc expression, and if Smad7 is required for nuclear accumulation of β-catenin in the canonical Wnt signaling pathway.
GSK-3 is a multifunctional serine/threonine kinase, acting as a key regulator in cellular responses evoked by Wnt, receptor tyrosine kinases and G-protein-coupled receptors (16a). GSK-3 has been reported to have important regulatory roles in apoptosis in a tissue specific manner. Thus, overexpression of GSK-3 in mammalian neuronal cells promotes apoptosis, while massive apoptosis is observed in the liver of GSK-3β−/− embryos, which causes their death around embryonic day 16. GSK-3β was found to be required for the NF-κB-mediated survival responses evoked by tumor necrosis factor alpha (27).
We report here that Smad7 is required for TGF-β-induced phosphorylation of Ser9 in GSK-3β. Several growth factors like insulin and epidermal growth factor have previously been shown to induce phosphorylation of GSK-3β on Ser9 and thereby inactivate this kinase. The inhibition of GSK-3β by insulin has been demonstrated to occur via a phosphatidylinositol 3-kinase-dependent pathway via activation of Akt (12a, 13, 16a). The exact mechanism whereby the canonical Wnt signal regulates GSK-3β is not well known, however, it has been reported that GSK-3β is not phosphorylated at Ser9 in response to Wnt signaling (16, 50). The observed accumulation of β-catenin induced by TGF-β in our study led us to examine the possible role of Akt and GSK-3β in this pathway, since p38 MAP kinase has been demonstrated to be involved in the phosphorylation of Akt on Thr308 and Ser473 (49). Activated Akt can in turn lead to the phosphorylation on Ser9 in GSK-3β and its inactivation (9).
We observed that TGF-β causes phosphorylation of GSK-3β 30 min after stimulation of cells and that phosphorylation of Akt on Ser473 occurs just 15 min after stimulation. Furthermore, TGF-β-induced phosphorylation of Akt required Smad7. These findings indicate that TGF-β-induced activation of p38 MAP kinase preceded the phosphorylation of Akt in PC-3U cells. However, after longer periods of TGF-β stimulation, i.e., 24 and 48 h, when PC-3U cells undergo apoptosis, the amounts of Akt phosphorylated on Ser473 declined, which then could promote an apoptotic response. The requirement of Smad7 for TGF-β-induced activation of p38 MAP kinase is consistent with our previous report that Smad7 facilitates the interaction between MKK3 and p38 MAP kinase in PC-3U cells (18) and is also in line with the report (58) showing that the TGF-β-induced activation of p38 MAP kinase is required for TGF-β-induced apoptosis of mouse mammary epithelial cells. As we found that Smad7 is required for the TGF-β-induced activation of p38 and phosphorylation of Akt and GSK-3β, we are currently investigating the possibility that Smad7 could regulate the activity of GSK-3β by acting as a scaffold between p38, Akt, and GSK-3β.
Acknowledgments
We are greatful to S. Itoh, R. Heuchel, A. Moustakas, S. Souchelnytskyi, H. Clevers, K. W. Kinzler, A. R. Nebreda, and B. Vogelstein for kindly providing expression vectors, cells, and reagents. We appreciate the critical comments on the manuscript given by A. Moustakas and P. ten Dijke.
This work was supported in part by grants from the Swedish Medical Research Council, M. Bergvalls Foundation, and the Swedish Cancer Society.
REFERENCES
Articles from Molecular and Cellular Biology are provided here courtesy of Taylor & Francis
Full text links
Read article at publisher's site: https://doi.org/10.1128/mcb.25.4.1475-1488.2005
Read article for free, from open access legal sources, via Unpaywall:
https://mcb.asm.org/content/25/4/1475.full.pdf
Citations & impact
Impact metrics
Citations of article over time
Alternative metrics
Article citations
Chimeric TβRII-SE/Fc overexpression by a lentiviral vector exerts strong antitumoral activity on colorectal cancer-derived cell lines in vitro and on xenografts.
Cancer Gene Ther, 31(1):174-185, 22 Nov 2023
Cited by: 0 articles | PMID: 37993543
Identification and characterization of colorectal-cancer-associated SNPs on the SMAD7 locus.
J Cancer Res Clin Oncol, 149(18):16659-16668, 18 Sep 2023
Cited by: 1 article | PMID: 37721570
Smad7 Antisense Oligonucleotide in Crohn's Disease: A Re-Evaluation and Explanation for the Discordant Results of Clinical Trials.
Pharmaceutics, 15(1):95, 28 Dec 2022
Cited by: 0 articles | PMID: 36678723 | PMCID: PMC9864707
Smad7 effects on TGF-β and ErbB2 restrain myofibroblast activation and protect from postinfarction heart failure.
J Clin Invest, 132(3):e146926, 01 Feb 2022
Cited by: 44 articles | PMID: 34905511 | PMCID: PMC8803336
CXCR4 induces podocyte injury and proteinuria by activating β-catenin signaling.
Theranostics, 12(2):767-781, 01 Jan 2022
Cited by: 12 articles | PMID: 34976212 | PMCID: PMC8692909
Go to all (83) article citations
Other citations
Data
Data behind the article
This data has been text mined from the article, or deposited into data resources.
BioStudies: supplemental material and supporting data
Similar Articles
To arrive at the top five similar articles we use a word-weighted algorithm to compare words from the Title and Abstract of each citation.
Transforming growth factor-beta1 (TGF-beta)-induced apoptosis of prostate cancer cells involves Smad7-dependent activation of p38 by TGF-beta-activated kinase 1 and mitogen-activated protein kinase kinase 3.
Mol Biol Cell, 14(2):529-544, 01 Feb 2003
Cited by: 153 articles | PMID: 12589052 | PMCID: PMC149990
Activation of the {beta}-catenin/T-cell-specific transcription factor/lymphoid enhancer factor-1 pathway by plasminogen activators in ECV304 carcinoma cells.
Cancer Res, 65(2):526-532, 01 Jan 2005
Cited by: 14 articles | PMID: 15695395
2-Methoxyestradiol-induced apoptosis in prostate cancer cells requires Smad7.
J Biol Chem, 280(15):14773-14779, 11 Feb 2005
Cited by: 23 articles | PMID: 15708859
Regulation of the versican promoter by the beta-catenin-T-cell factor complex in vascular smooth muscle cells.
J Biol Chem, 280(13):13019-13028, 24 Jan 2005
Cited by: 62 articles | PMID: 15668231