Abstract
Objective
Chronic systemic inflammation contributes to the pathogenesis of many age-related diseases. Although not well understood, alterations in the gut microbiota, or dysbiosis, may be responsible for age-related inflammation.Methods
Using stroke as a disease model, we tested the hypothesis that a youthful microbiota, when established in aged mice, produces positive outcomes following ischemic stroke. Conversely, an aged microbiota, when established in young mice, produces negative outcomes after stroke. Young and aged male mice had either a young or an aged microbiota established by fecal transplant gavage (FTG). Mice were subjected to ischemic stroke (middle cerebral artery occlusion; MCAO) or sham surgery. During the subsequent weeks, mice underwent behavioral testing and fecal samples were collected for 16S ribosomal RNA analysis of bacterial content.Results
We found that the microbiota is altered after experimental stroke in young mice and resembles the biome of uninjured aged mice. In aged mice, the ratio of Firmicutes to Bacteroidetes (F:B), two main bacterial phyla in gut microbiota, increased ∼9-fold (p < 0.001) compared to young. This increased F:B ratio in aged mice is indicative of dysbiosis. Altering the microbiota in young by fecal gavage to resemble that of aged mice (∼6-fold increase in F:B ratio, p < 0.001) increased mortality following MCAO, decreased performance in behavioral testing, and increased cytokine levels. Conversely, altering the microbiota in aged to resemble that of young (∼9-fold decrease in F:B ratio, p < 0.001) increased survival and improved recovery following MCAO.Interpretation
Aged biome increased the levels of systemic proinflammatory cytokines. We conclude that the gut microbiota can be modified to positively impact outcomes from age-related diseases. Ann Neurol 2018;83:23-36.Free full text
Age‐related changes in the gut microbiota influence systemic inflammation and stroke outcome
Abstract
Objective
Chronic systemic inflammation contributes to the pathogenesis of many age‐related diseases. Although not well understood, alterations in the gut microbiota, or dysbiosis, may be responsible for age‐related inflammation.
Methods
Using stroke as a disease model, we tested the hypothesis that a youthful microbiota, when established in aged mice, produces positive outcomes following ischemic stroke. Conversely, an aged microbiota, when established in young mice, produces negative outcomes after stroke. Young and aged male mice had either a young or an aged microbiota established by fecal transplant gavage (FTG). Mice were subjected to ischemic stroke (middle cerebral artery occlusion; MCAO) or sham surgery. During the subsequent weeks, mice underwent behavioral testing and fecal samples were collected for 16S ribosomal RNA analysis of bacterial content.
Results
We found that the microbiota is altered after experimental stroke in young mice and resembles the biome of uninjured aged mice. In aged mice, the ratio of Firmicutes to Bacteroidetes (F:B), two main bacterial phyla in gut microbiota, increased ~9‐fold (p<
0.001) compared to young. This increased F:B ratio in aged mice is indicative of dysbiosis. Altering the microbiota in young by fecal gavage to resemble that of aged mice (~6‐fold increase in F:B ratio, p
<
0.001) increased mortality following MCAO, decreased performance in behavioral testing, and increased cytokine levels. Conversely, altering the microbiota in aged to resemble that of young (~9‐fold decrease in F:B ratio, p
<
0.001) increased survival and improved recovery following MCAO.
Interpretation
Aged biome increased the levels of systemic proinflammatory cytokines. We conclude that the gut microbiota can be modified to positively impact outcomes from age‐related diseases. Ann Neurol 2018;83:23–36
A growing body of literature demonstrates bidirectional communications between the gut microbiota and the brain, termed “gut‐brain axis” or “microbiota‐gut‐brain axis.”1, 2, 3 Interactions between the gastrointestinal (GI) tract and the central nervous system (CNS) through this axis are capable of shaping the onset, severity, and/or outcome of a number of neurological diseases.4, 5, 6, 7, 8, 9, 10, 11, 12
Little is known about how the gut‐brain axis changes with aging. This lack of knowledge is noteworthy given that aging alone alters the gut microbiota (dysbiosis) and likely contributes to age‐related inflammation,4, 3, 4, 5, 6, 7, 8, 9, 10, 11, 12, 13, 14, 15 a condition often referred to as “inflammaging.”16, 17, 18 Importantly, age‐related dysbiosis may be a major contributor to the increasing prevalence of many age‐related diseases and the poorer outcomes observed in the elderly after acute injury. Stroke is predominately a disease associated with aging, and outcomes after experimental stroke are poorer in aged mice compared to young.19, 20, 21, 22, 23, 24, 25 In this work, we first found that the microbiota shifted after stroke in young mice and resembled that observed in uninjured aged mice. We then determined whether aged mice would benefit from manipulation of the gut microbiota to create a more youthful bacterial population. In this study, we first tested the hypothesis that a youthful microbiota, when established in aged mice, produces more positive outcomes following stroke. Conversely, an aged microbiota, when established in young mice, produces more negative outcomes following stroke. We demonstrate that a “youthful” microbiota in aged mice is beneficial both at baseline and after an injury.
After determining that the gut microbiome influences stroke outcomes, we subsequently tested the hypothesis that a heightened inflammatory response, as occurs with “inflammaging,” accompanies the aged microbiome when transplanted into young mice. We further assessed short‐chain fatty acid (SCFA) changes, because these compounds are primarily produced by gut microbiota. Previous studies suggest that these SCFAs decline with age,26 and SCFAs have important homeostatic and anti‐inflammatory effects.14 In these studies, we show that aged microbiome leads to an exaggerated systemic inflammatory response and reduced levels of SCFAs in young mice.
Materials and Methods
Mice
This study was conducted in accord with the National Institutes of Health Guidelines for the Care and Use of Animals in research and under protocols approved by the Center for Lab Animal Care at the University of Connecticut Health Center and the University of Texas McGovern Medical School. Young male C57BL/6 male mice (8–12 weeks) and aged in‐house C57BL/6 male mice (18–20 months) were used in this set of studies. One additional cohort of 24‐week‐old male mice was used in study 5 to assess the systemic cytokine response (see below). Mice were housed 4 to 5 per cage in standard facilities with a 12‐hour light/dark schedule in a temperature‐ (21.7–22.7°C) and humidity‐controlled (40–60 RH) controlled vivarium, with ad libitum access to food and water. It is important to note that the vivarium ambient temperature is set to conform to Association for Assessment and Accreditation of Laboratory Animal Care (AALAC) guidelines, though the temperature inside cages may reach 26 to 28
°C attributed to thermal contribution of animals and that the animals huddle together. An initial cohort of mice were housed in cages with wire mesh bottoms to prevent coprophagy. A second cohort of mice was housed on corncob bedding, and differences between fecal microbiome between the two cohorts were analyzed in order to determine whether housing on mesh affected the gut microbiota. Mice in the facility are housed in individually ventilated cages on irradiated 1/8‐in corncob bedding and receive irradiated, nutritionally balanced, pelleted rodent diet (Picolab rodent diet 20 (#5053) LabDiet, St Louis, MO, USA) and filtered tap water. Cages are serviced under high‐efficiency particulate air filtered animal‐transfer stations. This is a barrier facility that requires investigators to wear personal protective equipment for entry—including disposable gown, shoe covers, hair cover, mask, and gloves.
Source of Mice
Mice were ordered from commercial vendors and verified to be free of exclusionary pathogens. For this study, both young and aged C57Bl6 mice were from Charles River Laboratories (Wilmington, MA), mice were aged in‐house, and were all fed a presterilized (irradiated) diet (Picolab rodent diet 20 (#5053) LabDiet, St Louis, MO, USA) for 2 or more months before fecal transplant gavage (FTG) studies. Additional studies were conducted to determine whether age‐related changes in the microbiome were similar in cohorts born and raised in our facility to those mice ordered from commercial vendors and maintained in our facility for a minimum of 2 months to control for dietary and other environmental variables.
Fecal Transplantation
DONOR MICE AND HARVESTING FECES
Feces from 3 aged mice residing in different cages were collected and pooled; similarly, feces from 3 young mice were collected and pooled. Each pooled sample was diluted in chilled phosphate‐buffered saline solution (PBS; 120mg feces/1ml buffer) and homogenized for 5 minutes until a paste‐like consistency was achieved. The suspension was vortexed for 1 minute and centrifuged 800g for 3 minutes. The supernatant was collected, aliquoted, for gavaging into recipient mice and a frozen sample was used for further analysis at a later date.
RECIPIENT MICE
Recipient mice were treated with 50‐ul antibiotic gavages consisting of 500mg of streptomycin HCl/ml of sterile water for 2 consecutive days. The premise of the antibiotic treatment was to decrease the bacterial load in the recipient mice to reduce competition for the repopulating microbiota from the donor mice. Twenty‐four hours after the second antibiotic gavage, donor supernatant was orally gavaged (50μl) to recipient mice daily for 5 days. Recipient mice were maintained for up to 2 months after oral gavage. Continued presence of donor biome was confirmed by 16S before ischemic stroke was induced.
Fecal Collection and Sample Sequencing
Microbiota in fecal samples, representative of that in the distal colon, were collected from mice and stored in sterile tubes at –80°C until analyzed. Bacteria taxa in each fecal sample were analyzed by amplifying the V4 to V5 hypervariable regions of the 16S ribosomal RNA (rRNA) gene using high‐throughput sequence analysis (Illumina MisSeq platform; Illumina, San Diego, CA).27 Quality filtered 16S rRNA sequences were clustered into operational taxonomic units (OTUs), with 97% similarity, by closed reference OTU‐picking using the UCLUST algorithm and GreenGenes reference database (v13.5) as implemented in Quantitative Insights Into Microbial Ecology (QIIME versions 1.6 and 1.7).28, 29, 30 Sequences were checked for chimeras using ChimeraSlayer with standard options as implemented in QIIME. Sequences not clustered were identified using the Ribosomal Database Project to the lowest possible taxonomic level.31 The data were randomly rarefied to 10,000 sequences per sample before any downstream analysis.
Experimental Stroke
Transient cerebral ischemia was induced by occluding the right middle cerebral artery (MCA) with silicon sutures, 0.21 and 0.23mm in diameter for young and aged mice, respectively, under isoflurane anesthesia.32, 33 After 60 minutes of MCA occlusion (MCAO), the suture was removed to allow for reperfusion. Rectal muscle temperature was monitored and maintained at 37°C by a Monotherm temperature control system. Sham mice underwent the same procedure; however, the suture was not advanced to the middle cerebral artery. Following ischemic stroke, all animals were given 0.5ml of saline subcutaneously daily for 1 week in addition to access to soft food. In studies where the microbiota was altered by antibiotic and fecal gavages, mice were allowed 1 month to establish a new microbiota before MCAO or sham MCAO was conducted.
Behavioral Testing
Behavioral testing was conducted at the same time of day for each testing time point. Before testing, all mice were acclimated for 1 hour in the testing room in their home cages. All equipment was cleaned with 70% ethanol between trials. Animals were pretested on some of these tests to obtain baseline scores. Mice that died during the experimental timeline were excluded from all the behavioral analysis, including the data for the previous days, to avoid any bias and from infarct‐size analysis. Animal mortality is shown for survival curves.
NEUROLOGICAL DEFICIT SCORING
The Neurological Deficit Score (NDS) was performed to assess MCAO outcomes as previously described.34 The NDS was scored at reperfusion, 1 and 3 days post‐MCAO using a 5‐point scale where: 0=
no deficit; 1
=
forelimb weakness and torso turning to the ipsilateral side when held by tail; 2
=
circling to affected side; 3
=
unable to bear weight on affected side; and 4
=
no spontaneous locomotor activity, or barrel rolling.
OPEN FIELD TEST
Mice were placed in the front right corner of a clear, acrylic box (16×
16in), and activity was assessed for 10 minutes. Activity was measured as the total number of laser beam breaks using a computer‐operated PAS open field system (San Diego Instruments, San Diego, CA). Tests were administered before MCAO and at 3 and 7 days post‐MCAO for 20‐minute intervals using Noldus/EthoVision behavior tracking software.
HANG WIRE TEST
Motor strength was measured using the hang wire test at 3 and 7 days post‐MCAO or post‐sham MCAO. Mice were placed in the center of a wire‐cage top (18×
9in) that was slowly inverted and placed at a height of 36in above a cage containing regular bedding. The time that elapsed between inversion to the moment the mouse fell from the wire‐cage top was recorded. Results from three trials for each mouse (45‐minute gap between each trial) was averaged to obtain a single observation for a given mouse.
Cresyl Violet Staining
Following reperfusion mice were deeply anesthetized using an Avertin overdose; mice were perfused transcardially using ice‐cold PBS solution followed by 4% paraformaldehyde solution to fix tissues. Brains were harvested and cryoprotected in 30% sucrose for 72 hours. Each brain was frozen and sectioned (30μm) in a coronal plane using a frozen slicing microtome. Sections were stained with cresyl violet and imaged for further analyzing of infarct volumes, expressed as a percentage of the contralateral hemisphere using SigmaScan Pro5 (Systat Software Inc., Chicago, IL).35, 36
Plasma Collection
Blood was collected by cardiac puncture before perfusion and centrifuged. Plasma was aliquoted and stored at –80°C. Changes in circulating inflammatory cytokines were examined utilizing Multiplex assay kits (purchased from Bio‐Rad Laboratories, Hercules, CA, USA) as per manufacturer's instructions.
SCFA Analysis
SCFAs were analyzed in fecal samples as previously described.37 In short, fecal samples were diluted 1:10 (w/v) in 50% aqueous acetonitrile and homogenized. SCFAs in the supernatant were derivatized using a mixture of 12C6‐3‐nitrophenlhydrazine (200mM) and (N‐ethyl‐N'‐(dimethylaminopropyl) carbodiimide hydrolyzed urea derivative (120mM). Samples was further spiked with derivatized 13C6‐3NPH‐HCl and 5‐ul aliquots were analyzed by liquid chromatography/mass spectrometry (electrospray ionization negative mode) using an Acquity UPLC HSS T3 1.8µm, 2.1
×
100mm high‐performance liquid chromatography column.
Experimental Design
In the initial study, we determined whether the fecal microbiota, representing bacteria primarily residing in the colon, differed between young (8–12 weeks) and aged (16–18 months) mice. In study 2, we determined whether transient MCAO (60 minutes) altered the gut microbiota. Fecal pellets were collected from young and aged mice before MCAO and 7 days following reperfusion in MCAO mice or an equivalent time for sham‐operated mice. In study 3, we determined whether fecal microbiota could be altered in young mice to resemble that of aged mice using supernatant gavages from fecal suspensions of the aged mice (see above). Conversely, we determined whether fecal microbiota could be altered in aged mice to resemble that in young mice using supernatant gavages from fecal suspensions of the young mice. In study 4, we determined the effect of fecal transplants between young and aged age mice on infarct size and recovery from MCAO. In study 5, adult mice (24 weeks) received either microbiota from aged (18–20 months) or young donors (8–12 weeks) to assess their impact on circulating cytokines following MCAO or sham MCAO. In study 6, young mice (8–12 weeks) and aged mice (16–18 months) received either microbiota from aged (18–20 months) or young donors (8–12 weeks) to assess the changes in fecal SCFAs concentrations following FTG, and recovery was evaluated.
Statistical Analysis
Values are presented as mean±
standard error of the mean with the exception of categorical data, which are expressed using a box and whisker plot. Statistical significance was set at p
≤
0.05. For the box and whisker plot, the box boundary closest to zero represents the 25th percentile; the box boundary furthest from zero represents the 75th percentile; the horizontal line within the box represents the median, and the error bars above and below the box represent the 90th and 10th percentile, respectively. When the statistical package could not calculate the 90th and 10th percentile the error bars were omitted. Individual outliers are depicted as circular black symbols.
Parametric analysis consisted of a Student's t test or two‐way analysis of variance (ANOVA) followed by Holm‐Sidak, Bonferroni, or Tukey post‐hoc analysis, when appropriate. Because the NDS consists of categorical data, the Kruskal‐Wallace ANOVA on ranks was used followed by a Dunn's post‐hoc analysis, when appropriate. The Mantel‐Cox and the Gehan‐Breslow‐Wilcoxon tests were used to analyze survival after MCAO.
Differences in Phyla in the gut microbiota of young and aged mice were analyzed using the unweighted UniFrac distance and plotted in a principal coordinates analysis (PCoA). The UniFrac distance is a measure that takes into account the branch length shared by the young and aged microbiota when placed on a common phylogenetic tree.38 Survival following MCAO was analyzed using the Mantel‐Cox and Gehan‐Breslow‐Wilcoxon tests.
Results
Differences Exist Between Aged and Young Gut Microbiota Populations
We determined whether gut microbiota, represented by the bacteria residing in feces, differed between young (8–12 weeks) and aged (18–20 months) male mice. Figure Figure1A1A shows the relative abundance of phyla in the young and aged microbiota. The vast majority of the gut microbiota are represented by bacteria from the phyla, Bacteroidetes and Firmicutes, with the remaining phyla combined representing less than 5% of the total bacteria. Note that aging increases the relative abundance of the Firmicutes while decreasing that of the Bacteroidetes. This shift in these phyla are graphically represented in Figure Figure1B1B as the ratio of Firmicutes/Bacteroidetes (F:B). The F:B ratio increased ~9‐fold with aging (from 0.26±
0.04 to 2.31
±
0.41; p
<
0.001; n
=
17 and 15 for young and aged, respectively). Furthermore, we confirmed similar F:B ratios in mice born and raised in our facility to that observed in animals purchased from an outside vendor and housed for 4 weeks in our facility. There were no significant differences in microbiome composition between mice born and raised in‐house compared to those purchased for either young mice or aged mice. There was a significant difference between young and aged mice (***p
<
0.001 and *p
<
0.05 compared to young and aged mice born at different locations). These data are consistent with our results in Figure Figure1.1. Thus, the increase in F:B ratio was a function of age and not the vendor or facilities where mice were born. Although there were no significant differences between young or aged mice that were purchased or born in‐house, there were significant differences in the gut microbiome between young and aged animals.
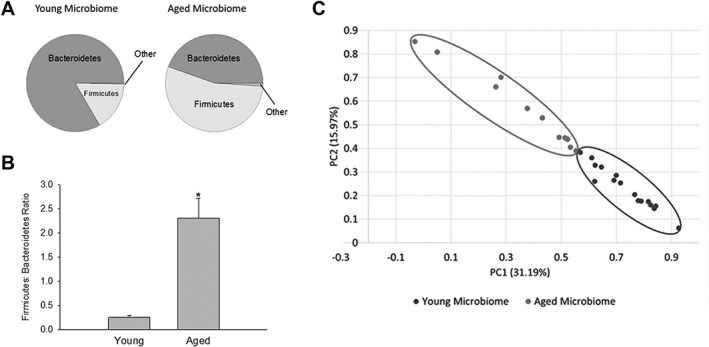
(A) Pie chart of phyla in the young and age microbiota obtained from 16S rRNA gene analysis in feces. (B) Firmicutes/Bacteroidetes (F:B) ratio in the young and aged microbiota. Firmicutes and Bacteroidetes comprise most of the bacteria in the gut. An increase in the F:B ratio is indicative of dysbiosis. *p<
0.001 (C) Principal coordinates analysis showing bacteria from the microbiota of young (N
=
16) and aged mice (N
=
11). p
=
0.009 between young and aged microbiota using analysis of similarity of UniFrac distance. PC
=
principal component; rRNA
=
ribosomal RNA.
In these studies, we will use the term “dysbiosis” to describe the increase in F:B ratio attributed to the fact that it represents a detrimental imbalance of the microbiota, and the definition of dysbiosis can differ in the literature.11 We demonstrate in subsequent studies that the aged microbiota fits our definition of dysbiosis. A number of indices have been developed to describe the degree of similarity or distance between microbiota communities. One of these indices, termed UniFrac distance, reduces the microbiota from the community in a single mouse to a coordinate, based on the phylogenetic distances between bacteria determined from the 16S rRNA sequencing.38 A PCoA plot (Fig (Fig1C)1C) demonstrates a clear separation in the UniFrac distances of the gut microbiota in young (circled in the lower right) and aged mice (circled in the upper left). Analysis of Similarity (ANOSIM) using the unweighted UniFrac distance matrix demonstrated that the aged microbiota was statistically significant from the young microbiota (p=
0.009). The PCoA analysis complements the F:B ratios (Fig (Fig1B)1B) in demonstrating that aging has a profound effect on the gut microbiota.
MCAO Causes Significant Shifts in the Microbiota
Figure Figure2A2A shows relative phyla abundance of the gut microbiota in young and aged mice before and 1 week after a 60‐minute MCAO. As observed with aging, MCAO decreased the relative abundance of the Bacteroidetes while increasing the relative abundance of the Firmicutes in young and aged mice. Note the appearance of other phyla following MCAO especially in the aged mice; the F:B ratios for data described in Figure Figure2A2A are shown in Figure Figure2B.2B. Note that stroke produced dysbiosis in the young mice and produced further dysbiosis in the aged mice as indicated by increased F:B ratios. Seven days poststroke, the F:B ratio increased more than 3‐fold in young mice (p<
0.001; n
=
16) compared to that in prestroke young mice. In aged mice, the poststroke F:B ratio increase more than 40% (p
=
0.014; n
=
14 and 15) compared to prestroke. Although the primary comparisons for these studies is between the F:B ratios pre‐ and poststroke in young mice and the F:B ratios pre‐ and post‐stroke in aged mice, we do note that the F:B ratio in young mice poststroke (0.72
±
0.13) was still less than the F:B ratio in aged mice preceding stroke (1.37
±
0.08).
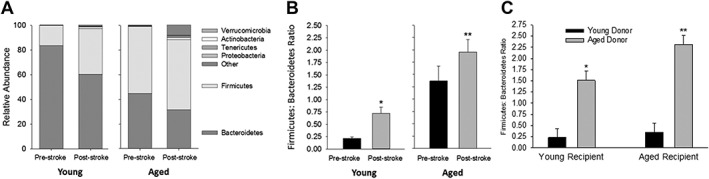
(A) Stacked bar chart of relative abundance of phyla in aged and young mice before and 1 week following MCAO. (B) Firmicutes/Bacteroidetes (F:B) ratio pre‐ and post‐MCAO in young and aged mice. MCAO significantly increased the F:B ratio in young (*p<
0.012) and aged mice (**p
=
0.014). (C) F:B ratio in young and aged recipient mice receiving contents from young and aged donor mice. Two‐way ANOVA revealed a significant recipient effect (p
=
0.03) and a significant donor effect (p
<
0.001). *p
<
0.001 compared to young mice with young microbiota (YY) and **p
≤
0.001 compared to all other groups using Holm‐Sidak post‐hoc analysis. ANOVA
=
analysis of variance; MCAO
=
middle cerebral artery occlusion.
Microbiota Manipulation by Fecal Transplants
We determined that after antibiotic treatment, the gut microbiota could be manipulated by gavaging supernatant from fecal suspensions of young mice into aged mice and, conversely, gavaging supernatant of aged mice into young mice. The study consisted of four groups of mice: (1) YY, young mice inoculated with the young microbiota (young control); (2) YA, young mice inoculated with aged microbiota; (3) AA, aged mice inoculated with the aged microbiota (aged control); and (4) AY, aged mice inoculated with young microbiota. One month after gavage from the donor mice, the F:B ratios of the recipient mice were similar to that of the donors (Fig (Fig2C).2C). Two‐way ANOVA revealed both significant recipient (p=
0.026) and donor (p
<
0.001) effects (n
=
14–16 per group). Mice transplanted with young microbiota showed the lowest F:B ratios regardless of the age of the recipient mice. Also, note that the F:B ratio in YY was similar to that in young mice without transplant (compare F:B ratios in Fig Fig2C2C to that in Fig Fig1B).1B). On the other hand, mice transplanted with aged microbiota showed an increased F:B ratio regardless of the age of the recipient (Fig (Fig2C).2C). Thus, we show that we cannot only successfully manipulate the microbiota to resemble that of the donor mice regardless of the age of the recipient mice, but also that these changes were sustained in the recipient.
Behavioral Outcomes Following MCAO Are Influenced by the Microbiota
In the next study, we determined the effect of young or aged microbiota on stroke outcome in cohorts of young and aged mice. The young cohort consisted of the sham‐operated and MCAO groups in that were inoculated with either young microbiota (YY) or aged microbiota (YA). Similarly, studies were conducted in aged mice that were inoculated with aged microbiota (AA) or young microbiota (AY). Mice were assessed using the NDS upon reperfusion, and 1 and 3 weeks following reperfusion (Fig (Fig3A;3A; n=
10–14 per group) or at equivalent time points for sham‐operated mice. After reperfusion, there were no significant differences in the NDS score of young mice with different gut microbiomes (Fig (Fig3A,3A, top panel) or in aged mice having different gut microbiomes (Fig (Fig3A,3A, bottom panel). NDS scores were increased at reperfusion (greater deficit) compared to subsequent trials at 1 and 3 weeks for both young (p
<
0.001) and aged mice (p
<
0.001, Kruskal‐Wallace one‐way ANOVA on ranks). At 1 week following reperfusion, AY mice showed an improved NDS score compared to AA mice (p
=
0.036), indicating an improved outcome with a young microbiome (Fig (Fig3A,3A, bottom panel). Although the NDS score was similarly improved in YY mice compared to YA mice, statistical significance was not achieved. At 3 weeks post‐MCAO, a similar pattern was observed in aged mice with the young microbiota trending toward an improvement. There were no significant differences in NDS among any of the sham groups (data not shown).
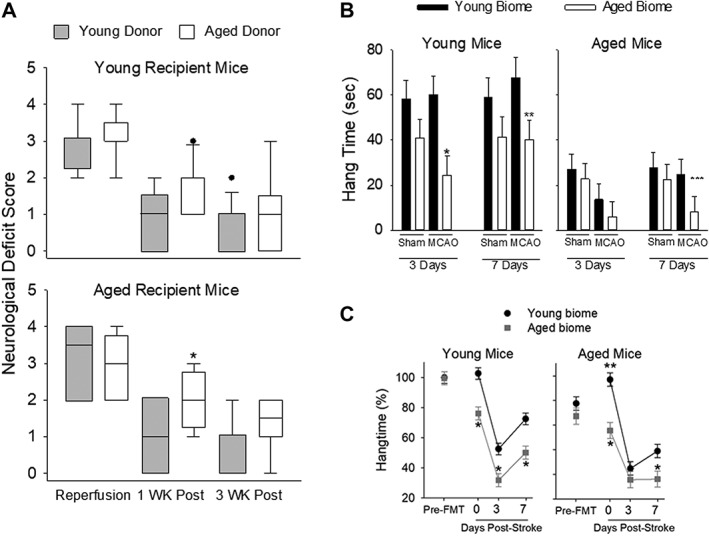
(A) Neurological Deficit Score (NDS) at the time of reperfusion and 1 and 3 weeks postreperfusion in young recipient (top panel) and aged recipient mice (bottom panel). Kruskal‐Wallis ANOVA on ranks showed statistical significance in both young (p<
0.001) and aged (p
<
0.001) mice (n
=
10–14 per group). *p
<
0.036 compared to AY. (B) Length of time mice could hang on a wire mesh once it was inverted. Studies were conducted 3 and 7 days post‐MCAO or post‐sham MCAO in young mice (left panel) or aged mice (right panel) having different microbiota (n
=
7 mice per group of young and aged recipients). In young recipient mice (left panel), there was a significant main effect of the microbiome at both 3 (p
=
0.004) and 7 days poststroke (p
=
0.018, two‐way ANOVA). *p
<
0.006 and **p
=
0.037 compared to corresponding group with young microbiome at the same time (Holm‐Sidak post‐hoc test). In aged mice (right panel), there was a significant main effect at 3 days (p
=
0.036). ***p
=
0.034 compared to AY at 7 days. (C) Percent time out of 5 minutes that mice spend hanging for hang‐wire behavior test. Note that mice with aged microbiome, regardless of chronological age, performed worse. There was a significant main effect of the microbiome in young mice (left) and aged mice (right; p
<
0.001 for both chronological age groups) and with time (p
<
0.001 for left and right panels). Pre‐FTG
=
prefecal transfer gavage. *p
≤
0.01 compared to corresponding time point; **p
=
0.036 compared to young biome pre‐FTG. ANOVA
=
analysis of variance; FMT
=
fecal microbiota transplantation; MCAO
=
middle cerebral artery occlusion.
Figure Figure3B3B shows the results of the hang‐wire test, a measure of motor strength, 3 and 7 days post‐MCAO or post sham‐MCAO in young (left panel) and aged (right, panel, n=
7 mice per group of young and aged) mice. In young mice (left panel), there was a main effect of the microbiome at both 3 (p
=
0.004) and 7 days poststroke (p
=
0.018, two‐way ANOVA, n
=
7 for all groups of young and aged mice). In addition to the main effect of microbiome at 3 and 7 days, there was also a significant effect of the microbiome at 3 days (p
=
0.037) and 7 days in in young MCAO (p
=
0.037, Holm‐Sidak post‐hoc test; Fig Fig3B,3B, left panel). Thus, mice with an aged microbiome had reduced motor strength overall (including sham and MCAO groups), but most pronounced at 3 and 7 days following MCAO compared to mice with a young microbiome.
Overall, aged mice (n=
7 per group) were unable to hang on to the wire mesh top as long as the young mice regardless of the microbiome or treatment group (compare left and right panels in Fig Fig3B).3B). At 3 days, there was a significant main effect of MCAO (p
=
0.036, two‐way ANOVA; Fig Fig3B,3B, right panel). Aged mice repopulated with young microbiota (AY) had an increased hang time after MCAO at 7 days (25
±
8 seconds) compared to the aged mice with aged microbiota (AA) after MCAO (8
±
8 seconds), which was significant (p
=
0.034, Mann‐Whitney rank‐sum test with Bonferroni correction). Some of the differences in hang time between young and aged mice could be attributed to the differences in body weight of young and aged mice. Regardless of a potential confounding effect of the greater weights observed in aged mice, the data are clear that young mice with an aged microbiota (YA) had decreased hang times following MCAO compared to young mice with a young microbiota (YY).
Open field activity was used to assess locomotor function and anxiety (n=
10 per group). In sham MCAO mice, there were no differences in open field activity at any time point regardless of the young or aged gut microbiota (Fig (Fig4A,4A, top two panels). However, open field activity in MCAO mice showed significant main effects for the microbiome and with time in young MCAO mice (p
<
0.001 and p
<
0.001, respectively, two‐way repeated‐measures ANOVA; Fig Fig4,4, left bottom panel) and in aged MCAO mice (p
<
0.001 and p
=
0.007, respectively, bottom right panel). Three days after MCAO, activities decreased in all groups compared to pre‐MCAO levels regardless of age or status of the microbiome. At 7 days post‐MCAO, activities rebounded to or toward that of the pre‐MCAO mice in all groups. However, YA was significantly less than YY at 7 days (P
<
0.001; Fig Fig4A,4A, bottom left panel) and AA was significantly less the AY at 7 days (P
<
0.001; Fig Fig4A,4A, bottom right panel). Additionally, activity levels in mice with aged microbiomes did not fully recover to the pre‐MCAO activity levels for young mice (p
<
0.001 for YA at 7 days compared to pre‐MCAO) or aged mice (p
<
0.001 for AA at 7 days compared to pre‐MCAO).
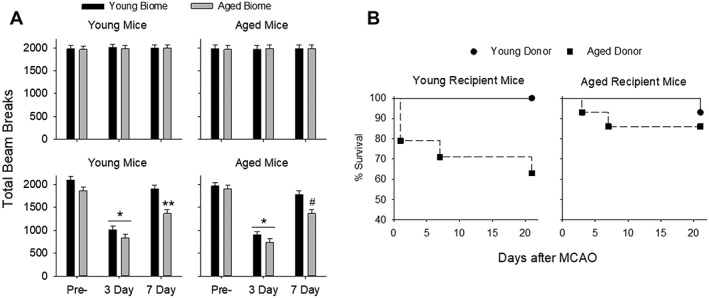
(A) Open field test to assess locomotor function and anxiety before MCAO (Pre‐) and 3 and 7 days postreperfusion in sham mice (top panels in A; n=
10/group). In sham MCAO mice, there were no differences in activity at any time point regardless of the young or aged gut microbiota (A, top two panels). Open field activity in MCAO mice showed significant main effects for the microbiome and for time in young MCAO mice (p
<
0.001 and p
<
0.001, respectively, two‐way repeated‐measures ANOVA, left bottom panel) and in aged MCAO mice (p
<
0.001 and p
=
0.007 right bottom panel). Activity significantly decreased from pre‐MCAO levels at 3 days regardless of microbiome (*p
<
0.001 compared to pre‐MCAO activity in the corresponding recipient age group). YA was significantly less than YY at 7 days (**p
<
0.001, bottom left panel) and AA was significantly less than AY at 7 days (#
p
<
0.001, bottom right panel). Additionally, activity levels in mice with aged microbiomes did not fully recover to the pre‐MCAO activity levels for young mice (p
<
0.001 for YA at 7 days compared to pre‐MCAO) or aged mice (p
<
0.001 for AA at 7 days compared to pre‐MCAO). (B) Survival in the various groups after MCAO. The increased mortality in the young mice having an aged microbiota was statistically significant by the Mantel‐Cox test (p
=
0.04) and the Gehan‐Breslow‐Wilcoxon test (p
=
0.03). ANOVA
=
analysis of variance; MCAO
=
middle cerebral artery occlusion.
Mice With an Aged Microbiota Have Increased Mortality Following MCAO
Mortality over 21 days in young and aged mice following MCAO is shown in Figure Figure4B.4B. Young mice with a young microbiota (YY) had no mortality 21 days after MCAO (n=
13). However, mortality in young mice reconstituted with aged microbiota (YA) increased to 36% (5 deaths in 14 mice) after MCAO. Aged mice with young microbiota (AY) had a 7% mortality rate (1 death in 14 mice) and aged mice with an aged microbiota (AA) had a 14% mortality rate (2 deaths in 14 mice) after MCAO. The increased mortality in the young recipients with aged microbiota was statistically significant by the Mantel‐Cox test (p
=
0.04) and the Gehan‐Breslow‐Wilcoxon test (p
=
0.03).
Effects of Altered Microbiota on Infarct Volume in Young and Aged Mice
To examine whether microbiota transplants influenced histological injury from stroke, cohorts of mice receiving fecal transplants were perfused and infarct size was analyzed using cresyl violet staining (Fig (Fig5A).5A). Regardless of the biome received, aged mice had significantly less cerebral atrophy 30 days after stroke compared to young mice (p<
0.001), consistent with previous studies.54 Significant differences were observed in percent of tissue loss with biome transfers in aged mice that received young microbiota (9.9
±
0.5%) compared to aged mice that received aged microbiota (13.1
±
1.0%; p
<
0.05), whereas no significant differences were found between young mice that received young microbiota (23.0
±
2.6%) compared to young mice that received aged microbiota (25.8
±
3.3%; p
<
0.05).
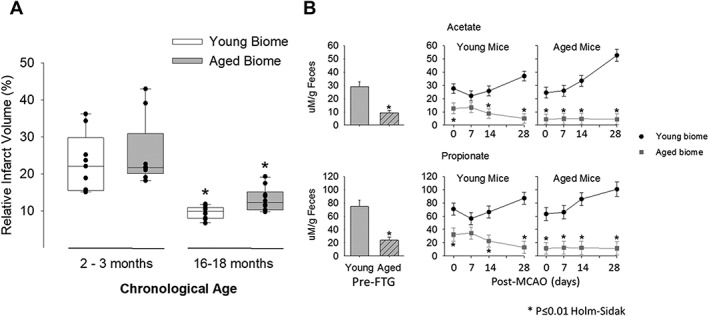
(A) Relative infarct volume 30 days after stroke in young and aged mice with either a young microbiota (biome) or aged biome. Regardless of the biome received, aged mice (N=
10) had significantly smaller levels of cerebral atrophy after stroke compared to young mice (N
=
10; p
=
0.004 Kruskal‐Wallis ANOVA on ranks). *p
<
0.05 compared to corresponding species in the young. (B, left side of panels) Fecal propionate and acetate compositions were significantly less in aged mice when compared to young at baseline p
<
0.001 for each SCFA, n
=
8/group, left side of the upper and lower panels). (B, right side of panels) Changes in acetate and propionate after fecal transplant gavage but immediately before MCAO (time 0) and several time points over 28 days post‐MCAO. As with the naïve mice, both acetate and propionate were decreased in mice with aged microbiomes. For acetate in both the young and aged mice (B, top panel), there was a significant main effect of microbiome (young or aged, p
<
0.001; N
=
8/group), a significant effect of time after MCAO (p
=
0.018), and a significant interaction between time after MCAO and microbiome (p
=
0.005). Similarly, propionate showed a significant main effect of the microbiome for both young and aged mice (p
<
0.001; N
=
8/group) and a significant interaction between microbiome and time (p
=
0.012). *p
≤
0.01 compared to corresponding group with an aged microbiome (Holm‐Sidak post‐hoc test). ANOVA
=
analysis of variance; MCAO
=
middle cerebral artery occlusion; SCFA
=
short‐chain fatty acid.
Reduced SCFAs Were Observed in Mice Harboring Aged Microbiota
Given that bacterial‐derived SCFAs have a prominent role in gut and brain health,39, 40, 41, 42, 43, 44 we measured SCFAs in fecal pellets. Figure Figure5B5B shows the levels of two important SCFAs in pellets from naïve young and aged mice (ie, no gavages or MCAO). Note that fecal levels for both acetate and propionate were decreased by ~68% for each SCFA in aged mice compared to that measured from young mice (p<
0.001 for each SCFA; n
=
8/group; left side of the upper and lower panels in Fig Fig5B).5B). Butyrate decreased by almost 80%, but did not reach statistical significance (Fig (Fig7).7). Fecal levels of butyrate and four other SCFAs in naïve young and aged mice are shown in Figure Figure77.
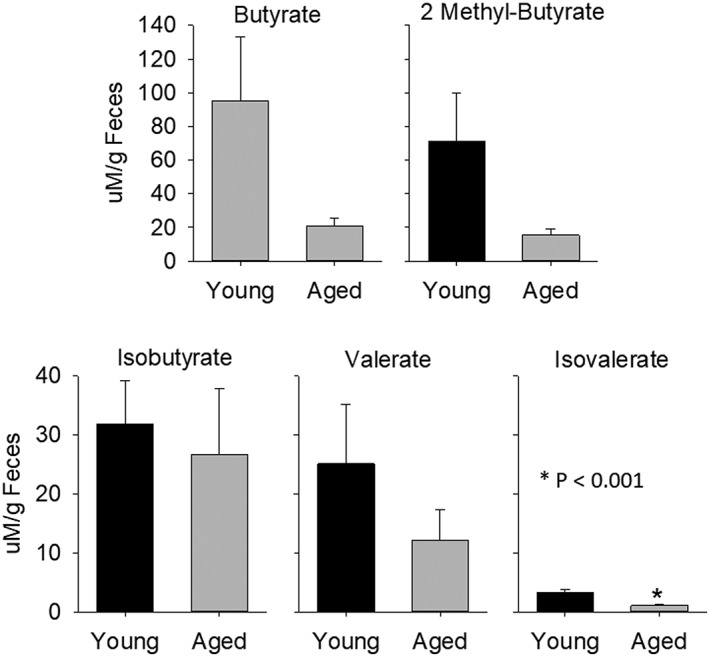
Short‐chain fatty acids, butyrate, isobutyrate, 2 methyl‐butyrate, valerate, and isovalerate in aged and young biomes, n=
8 per group. *p
<
0.001 compared to corresponding young.
Figure Figure5B5B (right side of the upper and lower panels) show the change in acetate and propionate after fecal transplant gavage, but immediately before MCAO (time 0) and several time points over 28 days post‐MCAO. Note that the level of acetate and propionate was determined by the microbiome (aged or young biome) and not by the age of the recipient mouse. As with the naïve mice, both acetate and propionate were decreased in mice with aged microbiomes.
For acetate in both the young and aged mice (5B, top panel), there was a significant main effect of microbiome (young or aged, p<
0.001; N
=
8/group), a significant effect of time after MCAO (p
=
0.018), and a significant interaction between time after MCAO and microbiome (p
=
0.005). Similarly, propionate showed a significant main effect of the microbiome for both young and aged mice (p
<
0.001; N
=
8 per group) and a significant interaction between microbiome and time (p
=
0.012).
Impact of Microbiota and MCAO on Circulating Immune Markers
Adult mice (24 weeks of age) were inoculated with microbiota from young or aged donors 1 month before MCAO or sham surgery. Cytokine levels measured from plasma 28 days after MCAO or sham MCAO demonstrated that the microbiota (young versus aged) had significant effects on the systemic cytokine response to stroke observed even a month after injury (Fig (Fig6).6). Protective cytokines had a more pronounced increase following MCAO in mice with a young microbiota compared to mice having aged microbiota as shown in Figure Figure6.6. Interleukin (IL)‐4 and granulocyte colony stimulating factor (G‐CSF) increased more in mice with young microbiota than mice having an aged microbiota. In Figure Figure6,6, bars having the same alphabet letter are significantly different from one another. Although IL‐10 did not reach statistical significance, it trended similar to IL‐4 and G‐CSF.
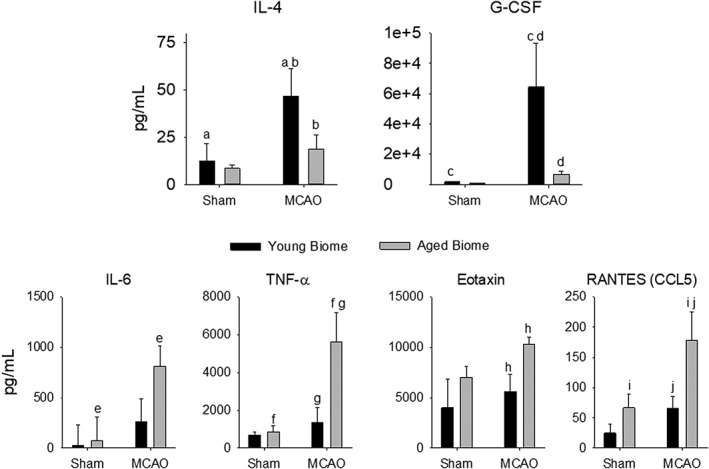
Plasma cytokines in adult MCAO or sham mice reconstituted with a young or aged microbiota. Plasma was sampled 7 days following the MCAO or sham MCAO. Two‐way ANOVA revealed significant effects of MCAO on IL‐4, G‐CSF (proinflammatory), IL‐6, TNFα, Eotaxin, and RANTES (anti‐inflammatory; p≤
0.04). Bars with the same letter are significantly different (p
<
0.05) using the Holm‐Sidak post‐hoc analysis. n
=
4 to 5 per group except Eotaxin and RANTES, which had 2 to 4 and 3 to 5 per group. ANOVA
=
analysis of variance; G‐CSF
=
granulocyte‐colony stimulating factor; IL
=
interleukin; MCAO
=
middle cerebral artery occlusion; RANTES
=
regulated on activation, normal T‐cell expressed and secreted; TNFα
=
tumor necrosis factor alpha.
Altering the microbiota had the opposite effects on several proinflammatory cytokines (bottom two rows in Fig Fig6).6). Young mice with aged microbiota had a greater increase in proinflammatory cytokines following stroke compared to adult mice with young microbiota. Taking the data as a whole, it is clear that the cytokine response is more proinflammatory in animals with aged microbiota compared to animals hosting young microbiota.
The Effect of Housing Conditions
One initial cohort of mice was housed in cages with suspended wire‐mesh bottoms, which allowed fecal pellets to drop through the mesh to prevent coprophagy. This allowed us to co‐house mice that had received young and aged biome in the same cage to avoid any cage bias. However, housing on mesh cage bottoms may have added stress, and although all animals were similarly exposed, this could have differentially affected young and old mice. To ensure that this did not affect our findings, we repeated the fecal transplant studies in a separate cohort of mice that were housed in ventilated cages on irradiated 1/8in corncob bedding. There was no difference in stroke outcomes or in the overall microbiome composition in mice housed on wire‐mesh or corncob bedding. Aged mice receiving young (AY) biome showed substantial improvement in recovery compared to AA mice. Young mice receiving an aged biome (YA) had slower recovery after stroke than YY mice. Figure Figure3C3C shows hang times for the second cohort (remained on bedding throughout the study) similar to studies described on mice housed on wire mesh in Figure Figure3B.3B. Qualitatively, the results in Figure Figure3B3B are similar to those shown in Figure Figure3C.3C. Our results suggest that the detrimental effects of aged microbiota and the beneficial effects of young microbiota on stroke recovery are independent of housing on wire mesh, and that the benefit of transfer of young biome into aged hosts on stroke outcome is consistent and reproducible.
Discussion
The present study demonstrates that the microbiota of young and aged mice have differential effects at baseline and following experimental stroke. Youthful microbiota, when established in aged mice, improved outcomes; conversely, aged microbiota, when established in young mice, led to more negative outcomes following stroke. Furthermore, aged microbiota enhanced systemic inflammation in young adult hosts in the absence of injury as observed in young sham mice with aged biota. Conversely, young biota enhanced behavioral and strength performance in aged mice even in the absence of injury. Our results provide hope for altering the course of age‐related diseases, given that the gut microbiota in humans is potentially modifiable. In addition to these novel findings, our studies also extend and amplify the ideas that gut dysbiosis occurs with aging, bidirectional communication between the gut and the brain exists in aged animals, and stroke produces dysbiosis in young mice and produces further dysbiosis in aged mice. In regard to external factors, it is important to note that the biome can change with ambient temperature and dietary factors. To avoid this, all of our animals were maintained at the same temperature and fed identical irradiated chow.66
Aging Produces Dysbiosis in Mice
Although it is generally accepted that the microbiota is altered in the elderly, the changes observed in humans is complicated by social and environmental factors, including the place of residence (home, nursing home, or hospital), habitual diet, lifestyle, and medications taken.14, 45, 46 Whereas better control of the diet and environmental conditions can be maintained in rodents, there are still some inconsistencies in the literature as to the change and degree of change that occurs with aging.13, 47, 48 Nevertheless, the general consensus is that the microbiota is altered with aging regardless of the species.
Our results support the idea that aging alters the gut microbiota in mice. When comparing fecal samples from aged (18–20 months) and young (8–12 weeks) male mice, we report a 9‐fold increase in the F:B ratio with aging (Fig (Fig1A,B)1A,B) and a clear separation in the UniFrac distances of the gut microbiota (Fig (Fig1C).1C). We propose that the changes in the gut microbiota provide potential mechanisms for the development or acceleration of pathological states associated with aging. These dysbiotic changes in the gut microbiota are likely a substantial component of “inflammaging,” the condition of increased inflammatory mediators occurring with aging.15, 47
Young Microbiota Improves Outcomes After MCAO in Aged Mice, While Aged Microbiota Impairs MCAO Outcomes in Young Mice
Our studies demonstrate that young gut microbiota had beneficial effects following 60‐minute MCAO in aged mice. Furthermore, aged microbiota, regardless of the age of host, negatively impacted outcomes compared to mice harboring young microbiota. For example, there were enhanced neurological deficits (neurological deficit scoring) at 7 days post MCAO (Fig (Fig3A),3A), reduced hang times (Fig (Fig3B,C),3B,C), decreased activity at 7 days post‐MCAO (Fig (Fig4A),4A), and decreased survival (Fig (Fig4B)4B) in mice with aged microbiota. When taking the data as a whole, mice with aged microbiota, regardless of age of the recipient mice, had worse outcomes compared to mice with a young microbiota. One particularly important finding is the mortality rate following MCAO. Note that young mice with young microbiota (YY) had no mortality at day 21 following MCAO. However, the mortality in young mice with aged microbiota (YA) dramatically and significantly increased to 36% (Fig (Fig4B;4B; p≤
0.04), suggesting a detrimental factor produced by the aged biota, or loss of a beneficial factor produced by young microbiota. Identification of these factors is the next step toward the development of practical therapeutics. Although we conclude that the “age” of the microbiota affects the outcome after MCAO, we do not yet know whether the young microbiota has a rejuvenating effect, an aged microbiota is toxic, or a combination of both.
A Bidirectional Communication of the Microbiota‐Gut‐Brain Axis Exists in the Context of Stroke
Our studies demonstrate that both directions of the “microbiota‐gut‐brain axis” are functional in the context of stroke in aged mice.1, 2, 3 First, we show that stroke produced gut dysbiosis in young mice and produced further dysbiosis in aged mice (“top‐down signaling”; Fig Fig2A,B)2A,B) and further increased the F:B ratio beyond that already occurring with age. The F:B ratio in aged mice, which was already increased compared to young mice, significantly increased by an additional 44% after stroke (Figs (Figs1B1B and and2B).2B). Furthermore, the age of the gut microbiota affected behavioral outcomes and recovery following stroke (“bottom‐up signaling”). Given that stroke and aging combine from the standpoint of “top‐down” and “bottom‐up” signaling to negatively affect outcome,12 it is interesting to consider that a vicious cycle is at play following stroke in the aged mice that slows mechanisms involved in recovery and enhance those mechanisms that worsen outcome, including an enhancement of inflammation.
Although the microbiota‐gut‐brain axis does exist, the details of how this communication occurs have not been fully clarified. For the “top‐down” signaling, stroke can directly or indirectly affect areas of the brain that control the autonomic nervous system (including sympathetic and parasympathetic modulation of enteric nervous system) and the hypothalamus‐pituitary‐adrenal axis. These systems have prominent roles in modulating gut function to include motility, acid secretion, bicarbonate and mucus regulation, fluid regulation, gut permeability, mucosal immune response, secretion of antimicrobial peptides, release of neurotransmitters into the gut, and altering the access of luminal bacteria and antigens to immune cell in the gut.1, 12, 50, 51 Perturbations in autonomic nervous system or the hypothalamus‐pituitary‐adrenal axis resulting from stroke could alter the gut environment with a consequential change in the microbiota serving to select for those species best adapted for the change.
For “bottom‐up” signaling, multiple mechanisms exist whereby the gut microbiota can influence the brain.1, 50, 51, 52, 53 Microbiota communicate with one another by releasing quorum sensing molecules, which include SCFAs, gamma amino‐butyric acid, tryptophan, serotonin, catecholamines, and metabolites of bile acids. These neurotransmitters and signaling molecules can interact with receptors in the gut wall and enteric nervous system to communicate to the brain through vagal afferents.53, 54 Bacterial metabolites can diffuse into the circulation and act in an endocrine role to signal distant targets such as the brain.1, 50, 55 Additionally, stroke in young and aged animals increases the permeability of the gut wall allowing for the translocation of bacteria and bacterial components (toxins and bacterial proteins) to produce, initiate, or enhance regional and systemic inflammation.1, 11, 19, 50, 51, 52, 56, 57
SCFAs, important signaling molecules in the body, are almost exclusively derived from bacterial metabolism in the gut.41, 42 These SCFAs, primarily butyrate, propionate, and acetate, can be highly beneficial and protective to the host by G‐protein‐coupled receptor activation or inhibition of histone deacetylases.41, 42, 43, 44 Working through either or both of these pathways, SCFAs stabilize the gut epithelial barrier, modulate cytokine secretion, alter T‐lymphocyte populations, increase the protective mucus layer, modulate antibody secretion, and inhibit nuclear factor kappa B.44 Additionally, SCFAs mediate the maturation and function of brain microglia and influence components of the blood–brain barrier.39, 40 We found that SCFAs in feces were reduced in animals with an aged microbiome (Fig (Fig7),7), regardless of the age of the recipient/host, and this reduction was associated with increased inflammation and worse outcomes following MCAO. Figure Figure77 shows SCFAs: butyrate, isobutyrate, 2 methyl‐butyrate, valerate, and isovalerate in aged and young biomes (*p<
0.001) compared to corresponding young. Propionate and acetate were decreased by ~68% in naïve aged mice compared to naïve young mice and, similarly, decreased in YA compared to YY mice (Fig (Fig5B).5B). The association of SCFAs and MCAO outcome suggests a protective role for the SCFAs and highlights the need for further studies in this area.
Thus, there are a number of potential signaling pathways by which gut microbiota can influence the brain following stroke. Regardless of the signaling mechanisms, “bottom‐up” interactions between the (GI) tract and CNS have been implicated in axonal myelination and demyelination, anxiety, depression, autism spectrum disorder, chronic pain, blood–brain barrier integrity, microglia maturation and function, the response to stress, pain modulation, and neuroinflammation.10, 11, 39, 40, 53, 58, 59 The metabolic and functional balance of the brain and CNS is disrupted by the process of aging, and the levels of SCFAs could play a major role in this process.
The Negative Effects of an Aged Microbiota Likely Involves an Enhanced Inflammatory Response Following Stroke
Although a number of mechanisms are likely involved with the bidirectional communications of the microbiota‐gut‐brain axis following stroke, one important consideration is the initiation of the inflammatory response by the gut microbiota.11, 12 Consistent with the idea of inflammaging, the immune system is likely more reactive in aged mice following insults such as stroke. Whereas recent studies have found that transplanting microbes from mice treated with antibiotics before stroke increased anti‐inflammatory T‐regulatory cells and reduced CNS leukocyte infiltration after stroke,11 a more recent study suggests that selective ablation of bacterial genes can lead to significant life‐span extension in Caenorhabditis elegans.65 Our results not only embrace this idea, but also they go beyond it by demonstrating that the microbiota in aged mice has a major contributory role in the heightened activity of the immune system with aging. Young mice hosting aged microbiota showed an attenuation in circulating anti‐inflammatory cytokines and an enhancement in the proinflammatory cytokines following stroke compared to young mice harboring young microbiota (Fig (Fig66).
This study demonstrates that age and the gut microbiota affect outcomes following stroke. If proven to be translational, then clinicians will have a novel approach to potentially influence stroke recovery, especially in the elderly. This could include proactive and preventive strategies to alter the gut microbiota through diet, probiotics (beneficial bacteria), prebiotics (substrate for beneficial bacteria), fecal transplant, or fecal substitute transplant—a process of transplanting purified intestinal bacteria derived from a healthy donor.15, 44, 60, 61, 62, 63, 64 Beneficial effects of probiotics include enhancing epithelial function, stabilizing the gut epithelial barrier during stress, modulating cytokine secretion, altering T‐lymphocyte populations, and modulating antibody secretion.51, 63 Therefore, using elements of precision and customized medicine, daily probiotics and/or supplements could be personalized depending on an individual's precise microbiota composition to act as protective measures in the event of stroke or other insults.
Author Contributions
M.S.S., V.R.V., M.J., S.J.D., R.M.B., and L.D.M. were involved in study concept and design. M.S.S., V.R.V., M.J., S.J.D., D.J.D., B.P.G., N.J.A., N.P., J.G., R.M.B., and L.D.M. were involved in data acquisition and analysis. M.S.S., V.R.V., M.J., S.J.D., B.P.J., D.J.D., N.J.A., N.P., J.G., R.M.B., and L.D.M. were responsible for drafting the manuscript and figures.
Acknowledgment
This project was funded by NINDS RO1 NSO94543 (L.D.M.), R01NS080531 and R21 NS094806 (R.M.B.); RF1AG058463, R01NS103592 (L.D.M. and R.M.B.), NIH Public Health Service Grant DK‐56338 (V.R.V., D.J.D.), and American Heart Association grants 16SDG29970000 (D.J.D.) and 15SDG23250025 (V.R.V.).
References

Full text links
Read article at publisher's site: https://doi.org/10.1002/ana.25250
Read article for free, from open access legal sources, via Unpaywall:
https://onlinelibrary.wiley.com/doi/pdfdirect/10.1002/ana.25250
Citations & impact
Impact metrics
Citations of article over time
Alternative metrics
Smart citations by scite.ai
Explore citation contexts and check if this article has been
supported or disputed.
https://scite.ai/reports/10.1002/ana.25250
Article citations
A potential role of gut microbiota in stroke: mechanisms, therapeutic strategies and future prospective.
Psychopharmacology (Berl), 241(12):2409-2430, 27 Oct 2024
Cited by: 0 articles | PMID: 39463207
Review
Changes in Type 1 Diabetes-Associated Gut Microbiota Aggravate Brain Ischemia Injury by Affecting Microglial Polarization Via the Butyrate-MyD88 Pathway in Mice.
Mol Neurobiol, 26 Sep 2024
Cited by: 0 articles | PMID: 39322832
An Oral Botanical Supplement Improves Small Intestinal Bacterial Overgrowth (SIBO) and Facial Redness: Results of an Open-Label Clinical Study.
Nutrients, 16(18):3149, 18 Sep 2024
Cited by: 0 articles | PMID: 39339748 | PMCID: PMC11435404
Gut microbiota-derived acetate promotes long-term recovery through angiogenesis guided by lymphatic ingrowth in older adults with stroke.
Front Neurosci, 18:1398913, 20 Sep 2024
Cited by: 0 articles | PMID: 39371609 | PMCID: PMC11450648
The Aging Immune System: A Critical Attack on Ischemic Stroke.
Mol Neurobiol, 14 Sep 2024
Cited by: 0 articles | PMID: 39271626
Review
Go to all (213) article citations
Similar Articles
To arrive at the top five similar articles we use a word-weighted algorithm to compare words from the Title and Abstract of each citation.
Different gender-derived gut microbiota influence stroke outcomes by mitigating inflammation.
J Neuroinflammation, 19(1):245, 04 Oct 2022
Cited by: 17 articles | PMID: 36195899 | PMCID: PMC9531521
Gut Microbiota-Derived Short-Chain Fatty Acids Promote Poststroke Recovery in Aged Mice.
Circ Res, 127(4):453-465, 01 May 2020
Cited by: 219 articles | PMID: 32354259 | PMCID: PMC7415518
Neuroprotective effects of fecal microbiota transplantation on MPTP-induced Parkinson's disease mice: Gut microbiota, glial reaction and TLR4/TNF-α signaling pathway.
Brain Behav Immun, 70:48-60, 20 Feb 2018
Cited by: 297 articles | PMID: 29471030
Intestinal microbiome as a novel therapeutic target for local and systemic inflammation.
Pharmacol Ther, 199:164-172, 12 Mar 2019
Cited by: 33 articles | PMID: 30877020
Review
Funding
Funders who supported this work.
American Heart Association (2)
Grant ID: 16SDG29970000
Grant ID: 15SDG23250025
NIA NIH HHS (1)
Grant ID: RF1 AG058463
NIDDK NIH HHS (1)
Grant ID: P30 DK056338
NIH (1)
Grant ID: DK‐56338
NINDS (5)
Grant ID: R01NS080531
Grant ID: R01NS103592
Grant ID: RF1AG058463
Grant ID: RO1 NSO94543
Grant ID: R21 NS094806
NINDS NIH HHS (4)
Grant ID: R01 NS103592
Grant ID: R01 NS080531
Grant ID: R21 NS094806
Grant ID: R01 NS094543