Abstract
Free full text

Metabolic Pathways and Potencies of New Fentanyl Analogs
Abstract
Up to now, little is known about the metabolic pathways of new fentanyl analogs that have recently emerged on the drug markets worldwide with high potential for producing addiction and severe adverse effects including coma and death. For some of the compounds, limited information on the metabolism has been published, however, for others so far no information is available. Considering the well characterized metabolism of the pharmaceutically used opioid fentanyl and the so far available data, the metabolism of the new fentanyl analogs can be anticipated to generally involve reactions like hydrolysis, hydroxylation (and further oxidation steps), N- and O-dealkylation and O-methylation. Furthermore, phase II metabolic reactions can be expected comprising glucuronide or sulfate conjugate formation. When analyzing blood and urine samples of acute intoxication cases or fatalities, the presence of metabolites can be crucial for confirmation of the uptake of such compounds and further interpretation. Here we present a review on the metabolic profiles of new fentanyl analogs responsible for a growing number of severe and fatal intoxications in the United States, Europe, Canada, Australia, and Japan in the last years, as assessed by a systematic search of the scientific literature and official reports.
Introduction
Opiates have been used for thousands of years to treat a broad variety of conditions. The first semi-synthetic opioids (such as heroin) were derived from the opium alkaloid morphine. In association with the discovery and deeper investigation of the opioid receptors numerous synthetic, structurally diverse opioids were developed by research chemists and pharmaceutical companies. Fentanyl has first been synthesized by Paul Janssen in 1959 (Janssen, 1965) and was derived from the synthetic opioid meperidine. Its pharmacological action is 50–100 times more potent than morphine and 25–40 times more than heroin, and it is commonly used in anesthesia and pain treatment (US Drug Enforcement Administration [US DEA], 2015; National Institute on Drug Abuse [NIDA], 2016). Fentanyl and its clinically used analogs are regarded as highly potent μ-opioid receptor agonists.
Besides the medicinal usage and progress in the therapeutic application of opioids, misuse of opioids has always been an issue. However, non-medical use of opioids often leads to health problems due to the high addictive potential of opioids and their severe acute side effects like respiratory depression. Repeated opiate and opioid use leads to tolerance, a contributing factor to opioid dependence. Development of tolerance is a controversially discussed topic and not fully understood yet. However, there is a consensus that different mechanism are involved, among them pharmacodynamic tolerance (adaptive changes in networks or pathways in organs and tissues affected by drug interaction), behavioral tolerance, pharmacokinetic (metabolic) tolerance and tachyphylaxis (Bespalov et al., 2016). In both clinical use and misuse tolerance may lead to dose escalation and finally severe adverse effects.
Over the last few years a wave of highly potent synthetic opioids emerged on the market of new psychoactive substances (NPS). These ‘new synthetic opioids’ (NSO) are often derived from fentanyl (also known as ‘designer fentanyls,’ ‘fentanyl derivatives,’ or ‘fentalogs’) and available at a cheaper cost compared to heroin (Marchei et al., 2018; Rothberg and Stith, 2018). Fentanyl analogs have recently been encountered as cutting agents in seized heroin samples, in ready-to-use preparations like nasal sprays or as ‘research chemicals’ marketed via internet shops. These drugs have caused an increasing number of acute intoxications and fatalities in North America, as well as in Europe, Japan, Canada, and Australia (Pichini et al., 2017, 2018). Plenty of pharmacokinetic studies have been published evaluating and characterizing receptor binding and potency of fentanyl (Costa et al., 1992; France et al., 1995) and its clinically relevant analogs (Henriksen et al., 2005; Volpe et al., 2011). When comparing binding constants, it has to be kept in mind that variables like type of assay, choice of competitive ligand etc. significantly impact the experimental outcome and may lead to varying values for identical compounds. In contrast, information on pharmacological data – and in particular metabolism – of non-medically used fentanyl analogs is scant, with evident difficulties in identifying the molecules in biological fluids of the consumers in order to assess consumption (Armenian et al., 2018). In addition, ratios of parent compound and metabolite concentrations can help to examine the plausibility of specific scenarios in forensic toxicology (e.g., acute vs. slow accumulative poisoning). An early study assessing the opioid-like activity of several fentanyl metabolites in a guinea pig ileum assay found that norfentanyl, 4-ANPP (4-anilino-N-phenethylpiperidine) and 4-anilinopiperidine (metabolites of fentanyl) were less potent than either fentanyl or morphine by several orders of magnitude (Schneider and Brune, 1986). The only metabolite showing significant activity in this study was a phenolic derivative hydroxylated at the 4-position of the phenylethyl moiety of fentanyl, the activity of which was found to lie between morphine and pethidine.
Nevertheless, some of the fentanyl analog metabolites might retain opioid activity with clinical relevance. What has been documented for fentanyl metabolism typically translates to the new designer fentanyls, which also show an extensive metabolism, however, to varying degrees. This review article summarizes the current knowledge on pharmacological data with a focus on the metabolism of novel fentanyl analogs.
Methods
Procedures for Assessment of Metabolic Profiles
To investigate the metabolism of a distinct compound, in vivo or in vitro approaches can be used: in vivo studies are performed in animals or humans, whereas in vitro approaches include the use of human liver microsome preparations, human hepatocytes or fungi as models for metabolism. In general, in vivo studies in humans would be the best choice due to limited transferability of animal data, but require ethical approval and are often not feasible. Human self-administration studies or the investigation of body fluids of death cases can serve as an alternative if available. However, such studies may show biased metabolic profiles due to health conditions or enzymatic phenotypes of study subjects. In vitro approaches generally do not reflect the full human metabolism, but are much easier to implement. Human hepatocytes are a commonly used model simulating human hepatic metabolism. However, due to varying factors like cell line and culture environment, the metabolic profile resulting from hepatocyte incubation may vary and does often not reflect the metabolic profile obtained in vivo sufficiently. Human liver microsomes or fungi like Cunninghamella elegans are further tools to produce in vitro metabolites. They are relatively easy to handle and cost-efficient, but may lack the ability to produce the whole human metabolic spectrum.
Analytical identification of metabolites is usually performed by mass spectrometric techniques like liquid chromatography-high resolution mass spectrometry (LC-HRMS) and use of different scan modes of tandem mass spectrometry. Differentiation of isomers often affords isolation of specific metabolites and nuclear magnetic resonance (NMR) spectroscopy analysis.
Literature Search
MEDLINE for biomedical literature and EMBASE for pharmacological literature as well as multidisciplinary databases such as Scopus and Web of Science were searched using the following combined terms: fentanyl analogs or analogs or derivatives or designer fentanyls or fentalogs, fentanyl, remifentanil, sufentanil, alfentanil, acetylfentanyl, acryloylfentanyl (or acrylfentanyl), α-methylfentanyl, butyr(-yl)fentanyl, carfentanil, cyclopropylfentanyl, cyclobutyl-fentanyl, cyclopentylfentany, cyclohexylfentanyl, 2,2,3,3-tetramethylcyclopropylfentanyl, crotonylfentanyl, 4-fluoro-isobutyr(-yl)fentanyl, isofentanyl, furanylfentanyl, methoxya-cetylfentanyl, ocfentanil, ortho-fluorofentanyl, tetrahydro-furanylfentanyl, metabolism, metabolic networks, metabolic pathways, μ-opioid receptor, opioid receptor binding. Further studies were retrieved by hand search through the reference lists of the selected articles. Moreover, a search for reports was conducted on Institutional websites, to identify documentation published by international agencies or institutions including the United States Drug Enforcement Administration (US DEA), United States National Institute on Drug Abuse (NIDA), World Health Organization (WHO) and the European Monitoring Centre for Drugs and Drug Addiction (EMCDDA).
General Remarks
Opioid Receptors
Opioid receptors are membrane bound G-protein coupled receptors predominantly located at the synaptic complex in the central nervous system but are also found in peripheral tissues. In the 1960s and 1970s first binding studies were performed by Van Praag and Simon (1966), Ingoglia and Dole (1970), Simon et al. (1973), Terenius (1973) and Pert and Snyder (1973) locating the opioid receptors in different brain areas using radio-labeled ligand assays. First proof for the existence of multiple opioid receptors was published by Martin (1967) proposing three different types of the opioid receptor (μ, κ, and δ). The μ-opioid receptor (MOR) named by its agonist morphine is mainly located in brain tissue and the gastrointestinal (GI) tract. This receptor mediates many of the typical opiate effects like analgesia, euphoria, miosis, physical dependence, reduced GI-mobility and respiratory depression. Three subtypes of the μ-opioid receptor (μ1, μ2, and μ3) have been identified, while μ1 is characterized best (Pan et al., 2005). The κ- and δ-opioid receptors are both found primarily in the brain tissue. For the κ-receptor three subtypes and for the δ-receptors two subtypes have been identified (Rothman et al., 1989; Portoghese and Lunzer, 2003). In principal, the same central nervous effects are produced by activation of the κ receptors as for the μ receptors, but additionally κ receptor agonists can cause hallucination and dissociation. δ-Opioid receptors are believed to contribute to analgesia as well, but also modulate immune response of myenteric neurons (Poonyachoti et al., 2001). Fentanyl and its analogs have been specifically designed for the activation of the μ-opioid receptors and usually show high selectivity for this receptor type. This is one of the factors complicating a direct comparison of morphine and fentanyl potency. Considering potency regarding central nervous effects, transmission through the blood-brain barrier has to be taken into consideration, too.
Fentanyl and Fentanyl Analogs
Fentanyl is a 2-phenylethyl-substituted 4-anilinopiperidine derivative carrying a propionylamide moiety linked to the aniline-nitrogen. In principle, there are four structural features which can potentially be modified, resulting in a huge variety of fentanyl analogs: (a) the piperidine ring, (b) the anilinophenyl ring, (c) the 2-phenylethyl substituent and (d) a carboxamide moiety linked to the anilino-nitrogen (Figure 1).
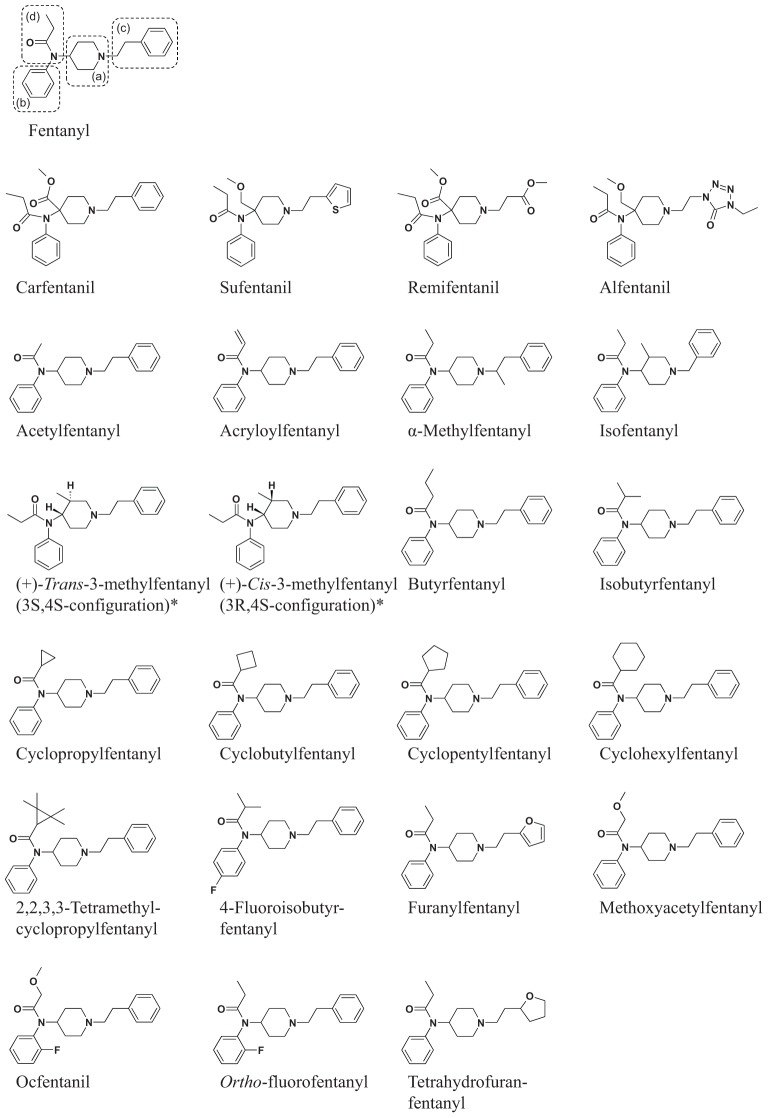
Chemical structures of fentanyl and reviewed fentanyl analogs with data on metabolism and/or potency available in the scientific literature. The structures marked with ‘’ show only one of the two enantiomers.
In the 1970s, Janssen Pharmaceutica patented a series of highly potent fentanyl derivatives, the N-4-substituted 1-(2-arylethyl)-4-piperidinyl-N-phenylpropanamides, such as the medically used Sufentanil and Carfentanil (Janssen, 1979). Carfentanil, which has been approved for veterinary use (Wildnil®) due to its extremely high potency, recently emerged as a designer drug on the recreational drug market, posing a huge health risk not only for users but also for first responders and law enforcement staff. Since the 1970s, a multitude of further analogs has been investigated (Brine et al., 1995; Wang et al., 1995; Vuckovic et al., 2009). One of the first fentanyl analogs on the designer drug market was the highly potent 3-methylfentanyl, methylated at the piperidine ring (a) (Figure 1) resulting in a pair of diastereomers (Van Bever et al., 1974). Several different substituents like halogen atoms, methyl or methoxy groups of the anilinophenyl ring (b) (Figure 1) have been published and some of these emerged on the designer drug market in recent years (United Nations Office on Drugs and Crime [UNODC], 2017). The 2-phenylethyl moiety (c) (Figure 1) substituted at the tertiary piperidinyl-nitrogen seems to improve receptor binding over non-substituted or methyl substituted compounds, presumably by fitting better into a hydrophobic cavity of the μ-opioid-receptor in close proximity to the active binding site (Jiang et al., 2000; Subramanian et al., 2000). Fentanyl analogs modified at this moiety like α-methylfentanyl have been reported to be involved in some fatal intoxication cases in the 1980s (Gillespie et al., 1982). The β-hydroxylated analog of 3-methylfentanyl, ohmefentanyl, has been well researched in the 1980s, showing extremely high potencies for some of the diastereomers (Subramanian et al., 2000). Modification of the propanamide moiety (d) (Figure 1) of fentanyl led to a huge variety of newly emerged fentanyl analogs in recent years (such as butyrfentanyl, furanylfentanyl, benzodioxole fentanyl, cyclopropylfentanyl, methoxyacetylfentanyl and many more). These derivatives are presently in the focus of research, since there is none or very little data available so far.
Fentanyl
Fentanyl is a medically used 4-Anilinopiperidine derivative like alfentanil, sufentanil, and remifentanil. These drugs are used in surgery as adjuncts to anesthesia, for sedation and for the treatment of acute and chronic pain (Van Bever et al., 1976; Van Daele et al., 1976; Kukanich and Papich, 2009).
First metabolism studies on fentanyl were conducted by Van Wijngaarden and Soudijn (1968) monitoring the parent compound and metabolites of radio-labeled fentanyl in urine and feces of rats after intravenous administration. In the late 1980s, Banks and Ferguson (1988) described fentanyl metabolism, indicating that several factors have to be taken into consideration in order to determine drug metabolism: administration routes (intravenous, subcutaneous, transdermal, transmucosal, and spinal), tissue chosen for analysis, isolation procedure and inter- and intra-individual variation that can influence metabolite formation and distribution (Streisand et al., 1991; Solassol et al., 2005).
Fentanyl (N-phenyl-N-[1-(2-phenethyl)-4-piperidinyl]pro-panamide) has several sites for metabolic transformation. It is a heterocyclic tertiary aliphatic amine containing two different phenyl rings and an aromatic amide function. Tertiary aliphatic amines are biotransformed through a reversible reaction into tertiary amine oxides. The tertiary amines also undergo N-dealkylation through the carbinolamine. When this process happens on the phenylethyl side chain, in addition to the secondary amine a phenylacetaldehyde is produced, which immediately oxidizes into phenylacetic acid. Oxidation at the 2-position of the piperidine ring generates a carbinolamine, which transforms into a more stable aminoaldehyde, resulting in ring cleavage. Aromatic rings undergo oxidation producing the equivalent phenolic derivatives. Furthermore, benzylic positions are more prone to oxidation. Amide functions usually undergo hydrolysis, and oxidation of the carbon chain is also frequent (Goromaru et al., 1984; Banks and Ferguson, 1988; Vardanyan and Hruby, 2014).
In humans, fentanyl is mainly metabolized in the liver by CYP3A4 into norfentanyl through oxidative N-dealkylation at the piperidine ring by hepatic CYP3A4 and 3A5 isoenzymes, which is the principal pathway of metabolism (Feierman and Lasker, 1996; Guitton et al., 1997; Labroo et al., 1997; Gudin, 2012; Bista et al., 2014; Armenian et al., 2018). The inactive metabolites and less than 10% of the intact molecule are mainly excreted in urine and feces (Mercadante, 2015; Kuip et al., 2017; Armenian et al., 2018) and less than 1% is metabolized by alkyl hydroxylation, combined N-dealkylation and hydroxylation or amide hydrolysis to the inactive compounds hydroxyfentanyl, hydroxy norfentanyl, and despropionylfentanyl (Kuip et al., 2017; Wu et al., 2017). The schematic human metabolic profile of fentanyl is depicted in Figure 2.
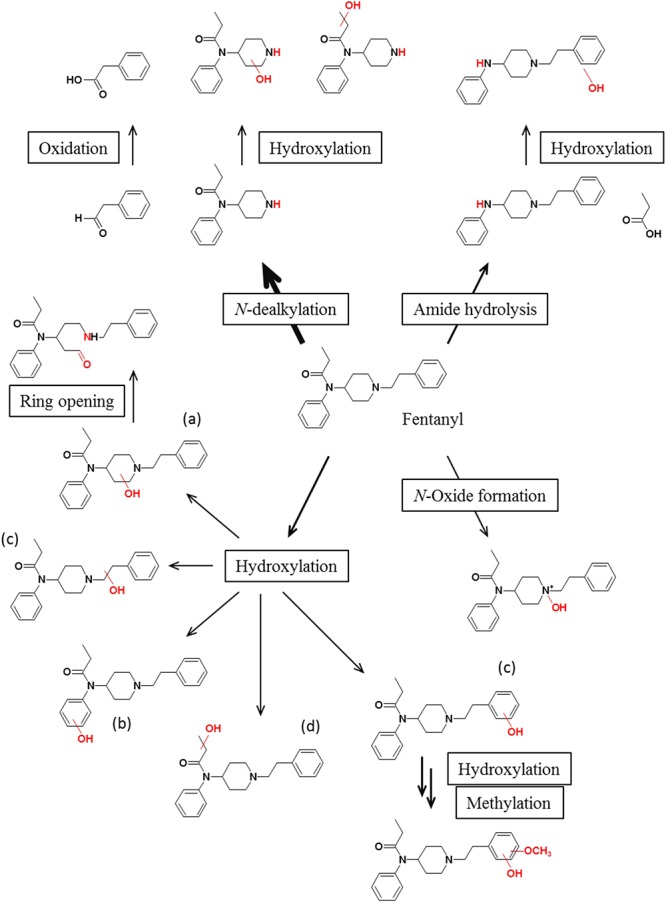
Schematic metabolic profile of fentanyl in humans, depicting the main biotransformations described in the literature. Main metabolic pathways are marked by bold arrows.
Fentanyl is also metabolized to norfentanyl in human duodenal microsomes; the mean rate is approximately half of the hepatic metabolism. Consequently, both intestinal and liver microsomes catalyze fentanyl metabolism and N-dealkylation by CYP3A4 is the principal active enzyme in both organs (Labroo et al., 1997). Hydroxylation occurs on the 2 or 3 position of the piperidine ring (a) (Figure 1), at the phenyl ring of the anilino moiety (b) (Figure 1), at the ethyl linker or the phenyl ring of the phenethyl moiety (c) (Figure 1), or along the amide alkyl chain (d) (Figure 1). The 4′-hydroxyfentanyl and other hydroxylated metabolites might be bioactive (Schneider and Brune, 1986), although the majority is believed to be inactive. The metabolite 4′-hydroxyfentanyl can undergo biotransformation via a second hydroxylation to allow a catechol that is then O-monomethylated to generate another metabolite. This reaction is probably catalyzed by the enzyme catechol-O-methyltransferase and presumably occurs at the 3′ position. This is technically a phase II metabolic product and can be detected in both hydrolyzed and non-hydrolyzed urine specimens due to its stability.
Minor metabolites such as hydroxypropionyl-fentanyl and hydroxypropionyl-norfentanyl are also created through different pathways without any relevant pharmacological activity. These metabolites have been detected in urine, stool and plasma (Bista et al., 2014).
Referring to despropionyl-fentanyl, another minor human metabolite, also known as 4-ANPP (Mahlke et al., 2014), results from carboxamide hydrolysis (Armenian et al., 2018).
Fentanyl is considered to be safer than morphine, in patients with liver and renal damage, because of a lack of metabolite accumulation (DePriest et al., 2015). Fentanyl activity can increase or decrease depending on genetic variations in the GI tract and in the liver, or through drugs which inhibit or induce CYP3A4. Fentanyl metabolism may be inhibited by macrolides, antifungal agents, and cimetidine (Bernard and Bruera, 2000). Serum fentanyl concentrations can vary significantly depending on liver function and the use of CYP3A4 inducers, therefore a model formula including these parameters has been provided, as a means to determine a transdermal fentanyl dose for the alleviation of cancer pain (Kokubun et al., 2012; Mercadante, 2015).
Bista and colleagues conducted a study to detect fentanyl and norfentanyl in plasma and saliva, showing that plasma and saliva had mean fentanyl concentrations of 0.785 and 3.335 μg/L, respectively. Similarly, in plasma and saliva the mean norfentanyl concentration was 0.53 and 0.517 μg/L, respectively. These data show that the concentration of fentanyl in saliva exceeds the concentration in plasma, suggesting an active transport into saliva. These data may in part be explained by the variable sample collection times with reference to time of dose, as distribution mechanisms will likely alter the saliva/plasma concentration ratio (Bista et al., 2014).
Alfentanil, Sufentanil, Remifentanil
In humans, the other fentanyl analogs frequently used in anesthesia – alfentanil, sufentanil, and remifentanil – are extensively metabolized and just a little percentage of the dose is excreted in urine without metabolic transformation. Metabolites such as norsufentanil and noralfentanil seem to be pharmacologically inactive (Valaer et al., 1997; Skulska et al., 2004).
Alfentanil (N-{1-[2-(4-ethyl-5-oxo-4,5-dihydro-1H-tetrazol-1-yl)ethyl]-4-(methoxymethyl)-piperidin-4-yl}-N-phenylpropanamide) and sufentanil (N-{4-(methoxymethyl)-1-[2-(thiophen-2-yl)ethyl]piperidin-4-yl}-N-phenylpropanamide) are also principally metabolized in the liver via the CYP3A4 hepatic pathway, which generates the same N-dealkylated inactive metabolite, making a forensic distinction impossible when only this metabolite is detected (Armenian et al., 2018).
Compared to fentanyl, alfentanil has a smaller volume of distribution, greater binding to plasma proteins, less binding to red blood cells, a shorter elimination half-life, a slower total body clearance, and is less lipid soluble – characteristics which suggest that alfentanil would be an appropriate drug to give by continuous i.v. infusion (Fragen et al., 1983).
Sufentanil is metabolized by the liver and enterocytes of the small intestines, catalyzed by the cytochrome P450 enzyme system (Donk et al., 2018). Sufentanil metabolites are excreted in the urine. N-Dealkylation of sufentanil leads to mostly inactive metabolites such as the metabolites formed by oxidative N-dealkylation at the piperidine ring (norsufentanil) or the phenylpropanamide nitrogen (leading to N-phenylpropanamide) and by aromatic hydroxylation (Lavrijsen et al., 1990; Tateishi et al., 1996; Koyyalagunta, 2007). Norsufentanil retains some activity, whereas the oxidative O-demethylation product (demethylsufentanil) is active retaining about 10% of the activity of sufentanil. However, it is produced in small quantities only and therefore not clinically relevant. The extensive metabolism of sufentanil in the GI tract is responsible for the low bioavailability following oral administration, so if a patient accidentally swallows a sublingual tablet this will result in under-dosing. Although the absence of clinically relevant metabolites makes sufentanil an option in mild-to-moderate renal impairment, there is insufficient data in patients with severe renal impairment, and hence careful patient monitoring is advised (Donk et al., 2018).
Remifentanil {methyl 1-(3-methoxy-3-oxopropyl)-4-[phenyl(propanoyl) amino]piperidine-4-carboxylate} is metabolized directly in the plasma by non-specific esterases, a hugely active group of enzymes found in blood and tissues throughout the body, resulting in an ultra-short duration of action (Rosow, 1999; Panzer et al., 2009). It is the only fentanyl analog that is 95% metabolized in the blood and tissues by non-CYP enzymes, because of an easily accessible ester group allowing for rapid hydrolysis by circulating blood esterases (Armenian et al., 2018). Its primary metabolite is remifentanil acid (a carboxylic acid derivative, GR90291), which has negligible pharmacological activity. Therefore, although remifentanil acid is excreted by the kidneys, remifentanil’s action is not prolonged to a significant extent by renal injury or prolonged infusion in patients in intensive care (Panzer et al., 2009; Cascone et al., 2018). Experimental in vivo evaluations of the metabolic kinetics are presently not available (Cascone et al., 2018).
Acetylfentanyl
Acetylfentanyl (N-Phenyl-N-[1-(2-phenylethyl)-4-piperidinyl] acetamide) is the acetyl amide analog of fentanyl. Relative potencies of several fentanyl analogs compared to fentanyl were evaluated by Higashikawa and Suzuki (2008a) in an animal study using the Litchfield–Wilcoxon test after peroral administration of diluted solutions of the fentanyl analogs to mice. ED50 and LD50 values obtained for acetylfentanyl were 0.021 and 9.3 mg/kg, respectively, suggesting about 30% of the analgesic potency of fentanyl.
In general, acetylfentanyl is metabolized in a similar way to fentanyl. Acetylfentanyl has a major primarily inactive metabolite, acetyl norfentanyl, produced by N-dealkylation via CYP450 enzymes (Patton et al., 2014; Watanabe et al., 2017). Melent’ev et al. (2015) investigated metabolism of acetylfentanyl in urine samples collected from fatal intoxication cases with this fentanyl analog. In this study, besides the proposed main metabolite acetyl norfentanyl and the deacetylated acetylfentanyl metabolite (4-ANPP), primarily hydroxylated acetylfentanyl metabolites and their phase II conjugates were detected. Hydroxylated metabolites of acetylfentanyl were also identified after incubation with hepatocytes (Kanamori et al., 2018b), including a 4′-hydroxy-3′-methoxy-metabolite which has also been found as a metabolite of fentanyl and was also detected by Melent’ev et al. (2015) in the death cases involving acetylfentanyl. In an additional work Kanamori et al. (2018a) determined the involvement of different CYP isoenzymes in the formation of the metabolites of acetylfentanyl described above. Moreover, Watanabe et al. (2017) identified 31 metabolites of acetylfentanyl in human hepatocytes and authentic human urine samples, including the β-hydroxy and 4′-hydroxy-3′-methoxy metabolite, and several other phase I and phase II metabolites formed via various pathways such as glucuronidation, sulfation, dihydroxylation, monohydroxylation, carbonylation, and dihydrodiol formation.
Acryloylfentanyl
The fentanyl analog acryloylfentanyl (acrylfentanyl, N-Phenyl-N-[1-(2-phenylethyl)-4-piperidinyl]-acrylamide) differs from fentanyl only in dehydration in the 2,3-position of the propionylamide moiety. The competitive binding affinity of acryloylfentanyl was determined by Maryanoff et al. (1982) in rat brain using tritium-labeled naloxone. The IC50 value obtained was 1.4 nM and therefore similar to fentanyl (IC50 1.6 nM). The analgesic properties of acryloylfentanyl were investigated by Essawi (1999) and it was found to be less potent than fentanyl (approximately 75% of fentanyl potency), but the analgesic effects persisted considerably longer. Though, the acrylamide moiety may lead to irreversible receptor binding and higher toxicity. However, LD50 values for acryloylfentanyl and fentanyl were 0.082 and 0.062 mg/kg, respectively, suggesting similar acute toxicity.
Similarly to fentanyl, acryloylfentanyl is lipophilic and expected to easily cross the blood-brain barrier. Distribution into fat and other tissues seems likely due to the presumably high volume of distribution (Ujváry et al., 2017). The metabolic pathway of acryloylfentanyl shows similarity with the pathways of fentanyl and acetylfentanyl. The main metabolites generated by human hepatocytes in vitro and of those detected in the urine in a few fatalities, caused by acryloylfentanyl, and their chemical structures were recently described by Watanabe et al. (2017). Overall, 14 biotransformation products, including major metabolites of acryloylfentanyl detected in human urine after hydrolysis of glucuronidated and/or sulfated phase II conjugates were identified in this work. The biotransformations involve an oxidative N-dealkylation, presumably catalyzed by cytochrome P450 (CYP450) enzymes, leading to the desphenethyl metabolite acryloylnorfentanyl which is biologically inactive. Furthermore, monohydroxylations were observed either at the alkyl linker of the phenylethyl moiety or at the piperidine ring. Dihydroxylation of the phenyl ring of the phenylethyl moiety resulting in a catechol structure followed by O-monomethylation were additional oxidative metabolic processes leading to similar metabolites as described for acetylfentanyl in the same work. Similar to fentanyl metabolism, amide hydrolysis (deacylation) results in a minor metabolite 4-ANPP, which is a common metabolite of fentanyl, acryloylfentanyl and several other fentanyl analogs. Acryloylfentanyl was also present in the urine of the deceased individuals.
With respect to acryloylfentanyl, the major human urinary metabolites identified in vivo (fatal cases) were acryloylnorfentanyl, as well as mono- and dihydroxylated derivatives and their conjugates (Watanabe et al., 2017).
α-Methylfentanyl and (cis/trans)-3-Methylfentanyl
As one of the mono-methylated fentanyl derivatives, α-methylfentanyl (N-Phenyl-N-[1-(1-phenyl-2-propanyl)-4-piperidinyl]propanamide) carries the additional methyl group at the 1-position of the ethyl bridge of the phenethyl moiety. The diastereomeric pairs of enantiomers cis-3-methylfentanyl and trans-3-methylfentanyl carry the additional methyl group at the 3-position of the piperidine ring. The analgesic activity of these derivatives proved to be similar to fentanyl or higher. In a study of Higashikawa and Suzuki (2008a) α-methylfentanyl showed a very similar ED50 value as fentanyl (0.0058 and 0.0061 mg/kg, respectively). However, toxic effects occurred at significantly lower doses of α-methylfentanyl when compared to fentanyl (LD50 values 8.6 and 62 mg/kg, respectively). Van Bever et al. (1974) synthesized the different isomers of α-methylfentanyl and 3-methylfentanyl and investigated their relative analgesic potencies. ED50 values for α-methylfentanyl (0.0085 mg/kg) obtained in this work were in agreement with the reported values of Higashikawa and Suzuki (2008a), although fentanyl showed a higher value here (0.011 mg/kg). The (±)-trans-3-methylfentanyl enantiomers (ED50 0.0094 mg/kg) showed about the same effective dose as α-methylfentanyl, but the (±)-cis-enantiomers turned out to be even more potent and exhibited a significant difference between the (+)- and (-)-enantiomer. The most potent isomer was (+)-cis-3-methylfentanyl (ED50 0.00058 mg/kg) being about 20 times more potent than fentanyl, whereas the (-)-cis-isomer showed only 20% of the potency of fentanyl.
The first reports about detection of a metabolite of α-methylfentanyl were published by Gillespie (Gillespie et al., 1982), who found the hydrolysis product despropionyl-α-methylfentanyl in several biological samples of fatal intoxication cases. Higashikawa and Suzuki (2008b) investigated the metabolism of α-methylfentanyl in urine after administration to rats. The main metabolite formed by N-dealkylation in this study was norfentanyl, a metabolite shared with fentanyl. Furthermore, two metabolites in common with fentanyl were formed by further hydroxylation of the propionylamide moiety of norfentanyl [ω-hydroxypropionyl-norfentanyl and (ω-1)-hydroxypropionyl-norfentanyl]. However, four additional metabolites were identified enabling differentiation of α-methylfentanyl and fentanyl consumption resulting from mono- and dihydroxylation of α-methylfentanyl [ω-hydroxypropionyl-α-methylfentanyl, (ω-1)-hydroxypropionyl-α-methylfentanyl, para-hydroxy-phenyl-α-methylfentanyl and para-hydroxyphenyl-ω-hydroxypropionyl-α-methylfentanyl].
Investigations in rat performed by Sato et al. (2010) confirmed the findings of Higasikawa and Suzuki regarding the metabolic spectrum and demonstrated the time-course of metabolite excretion as well as the proportions of metabolites excreted in rat urine over a 96 h time period. Non-specific metabolites of α-methylfentanyl were detectable up to 72 h after administration, whereas the specific metabolites were completely eliminated after 48 h and accounted for only 2–3% of the total amount of metabolites excreted in urine.
First detection of single metabolites of the methylated fentanyl analog 3-methylfentanyl was reported by Hammargren and Henderson (1988) who detected the dealkylated metabolite nor-3-methylfentanyl in urine of suspected drug users. A systematic investigation of the metabolism of 3-methylfentanyl was done by Meyer et al. (2012) proposing a metabolic pathway for this fentanyl analog and reporting 9 phase I and 5 corresponding phase II metabolites in rat urine after drug administration. In accordance to Hammargren and Henderson, the main metabolite detected was nor-3-methylfentanyl formed by oxidative N-dealkylation. Further oxidation of this metabolite led to formation of hydroxypropionyl-nor-3-methylfentanyl and hydroxyphenyl-nor-3-methylfentanyl. In addition, mono- and dihydroxylations were observed primarily at the phenylethyl and the propionylamide moiety followed by either further oxidative reactions leading to a carboxy-propionyl metabolite or methylation of the 3,4-dihydroxyphenyl metabolite leading to a 3-methoxy-4-hydroxy metabolite in analogy to previously reported fentanyl and fentanyl analog metabolites. Furthermore, Meyer et al. (2012) also reported phase II glucuronic acid conjugates detected for hydroxylated metabolites.
Isofentanyl
Isofentanyl (N-(1-benzyl-3-methylpiperidin-4-yl)-N-phenylpro-panamide) was clandestinely synthesized to circumvent 3-methylfentanyl regulation.
Meyer and collaborators identified isofentanyl together with 3-methylfentanyl phase I and phase II metabolites in rat urine (Meyer et al., 2012). Isofentanyl is an isomer of fentanyl and shares some main fragment ions in MS analysis. Metabolites such as the nor-metabolite can help to unequivocally prove uptake of this compound. For isofentanyl 11 phase I and 4 phase II metabolites were identified. The following metabolic steps could be postulated: N-dealkylation resulting in a common metabolite with 3-methylfentanyl (nor-3-methylfentanyl = nor-isofentanyl) followed by hydroxylations of the alkyl and/or aryl moiety, hydroxylation of the propionylamide side chain followed by oxidation to the corresponding carboxylic acid, and hydroxylations of the benzyl moiety followed by methylation resulting in the corresponding 3-methoxy-4-hydroxy metabolite. In addition, N-oxidation of isofentanyl was also observed. Some hydroxylated metabolites were partly excreted as glucuronides. Using recombinant human isoenzymes, CYP2C19, CYP2D6, CYP3A4, and CYP3A5 were found to be involved in the initial metabolic steps. The parent drugs could not be detected in urine. Their common nor-metabolite was suggested as a common target for urine screening for 3-methylfentanyl and isofentanyl. Targeting less abundant specific metabolites may enable differentiation of an uptake of either of the drugs (Meyer et al., 2012).
Butyrfentanyl and Isobutyrfentanyl
Butyrfentanyl (N-Phenyl-N-[1-(2-phenylethyl)-4-piperidinyl] butanamide) belongs to the mono-methylated fentanyl derivatives carrying the additional methyl group at the ω-carbon of the propionyl amide resulting in a butyryl amide analog of fentanyl. Isobutyrfentanyl is the isomer carrying the additional methyl group at the α-carbon of the propionylamide moiety. Both compounds were included in the activity studies of Higashikawa and Suzuki (2008a). In a study from Alburges et al. (1992) the binding affinity of butyrfentanyl to the μ-opioid receptor was reported (Ki = 32 ± 4.1 nM), which is about 32-fold lower than the binding affinity of fentanyl (Ki = 1.03 ± 0.15 nM).
Metabolites of butyrfentanyl were detected and identified in a fatal poisoning described by Staeheli et al. (2016) with focus on the post mortem tissue distribution and redistribution, a phenomenon often observed when analyzing post mortem samples. The identified metabolites were norbutyrfentanyl, carboxybutyrfentanyl, hydroxybutyrfentanyl, and desbutyrfentanyl. In pursuit of elucidation of the metabolism of butyrfentanyl, blood and urine samples of the same fatal intoxication case in conjunction with in vitro studies producing phase I and phase II metabolites of butyrfentanyl were investigated by Steuer et al. (2017). Human liver microsomes and recombinant cytochrome P450 enzymes (CYP) were used for in vitro assays. Butyrfentanyl was shown to undergo extensive metabolism. In total, 36 metabolites were identified in this study. The postulated primary metabolic pathways were hydroxylations at the butanamide side chain (in two positions), the phenylethyl moiety and the piperidine ring, oxidative N-dealkylation, formation of N-oxides and hydrolysis of the acyl moiety. Besides that, combinations of these biotransformations and additional reactions were observed leading to, e.g., carboxylated metabolites by further oxidation of the ω-hydroxy-butanamide moiety or methylation of the 3,4-catechol moiety of dihydroxylated metabolites forming the respective 3-methoxy-4-hydroxy metabolites. Furthermore phase II conjugates were detected in the human post mortem samples for nine metabolites (eight glucuronic acid conjugates and one sulfate). The main metabolites detected in the in vitro studies, nor-butyrfentanyl, butyrohydroxy-butyrfentanyl and phenylethyl-hydroxy-butyrfentanyl, were not in agreement with the main metabolites detected in authentic biological samples. The main metabolites detected in vivo were carboxy-butyrfentanyl in blood and carboxy-butyrfentanyl, butyrohydroxy-butyrfentanyl and carboxy-phenylethyl-hydroxy-butyrfentanyl in urine. Initial screening experiments with the most relevant CYPs indicated that mainly CYP2D6 and 3A4 were involved in the primary metabolic steps. Therefore, variability of phenotypes regarding these enzymes may have an influence on the metabolic profile in vivo. As a strategy to reach maximum detectability it seems advisable to include metabolites formed by different pathways as targets into analytical methods.
Carfentanil
Carfentanil [methyl 1-(2-phenylethyl)-4-[phenyl(propanoyl) amino]piperidine-4-carboxylate] is a member of the N-4 substituted fentanyl analogs carrying an additional methyl-carboxylate moiety at the 4-position of the piperidine ring. This group of fentanyl analogs turned out to be significantly more potent than their non-substituted analogs. Carfentanil is about 10,000 times more potent than morphine and shows 30–100 times the potency of fentanyl (Van Bever et al., 1976), thereby representing the most potent approved opioid drug. Receptor binding affinity and analgesic activity of this compound has been investigated extensively by many research groups, reporting ED50 values from 0.00032 nM up to 0.0017 (<0.01) nM and Ki values for the μ-opioid receptor of 0.024 nM up to 0.15 nM (Thompson et al., 1987; Costa et al., 1992; Maguire et al., 1992; Villemagne et al., 1994; Bi-Yi et al., 1999; Jewett and Kilbourn, 2004; Henriksen et al., 2005). Carfentanil is used in veterinary medicine as general anesthetic, for pain management, and to immobilize large animals (Kukanich and Papich, 2009).
Due to its extremely high potency studies assessing the metabolism of carfentanil in humans have not been performed yet and it seems unlikely that they would be approved by an Ethics Committee. Though, metabolites of carfentanil have only been detected in fatal intoxication cases so far, the most well-known case being the Moscow Theater hostage-taking (Riches et al., 2012). Riches et al. (2012) detected the N-dealkylated metabolite norcarfentanil in a donated urine sample. Norcarfentanil is a common (minor) metabolite of the fentanyl analog remifentanil. First and only studies assessing the metabolic pathways of carfentanil were performed by Feasel et al. (2016) using metabolism predictions software (Molecular Discovery’s MetaSite software and Simulations Plus’s ADMET Predictor) for first in silico prediction and human liver microsomes and hepatocytes as in vitro models.
In total, 12 metabolites were identified for carfentanil, 11 phase I metabolites and 1 phase II conjugate as glucuronide. The following metabolic reactions or combinations of these were observed: oxidative N-dealkylation, ester hydrolysis, hydroxylation and N-oxide formation. The most abundant metabolites reported were formed by N-dealkylation partly followed by ester hydrolysis or hydroxylation. Hydroxylations occurred at the propionylamide side chain, at the phenylethyl moiety or at the piperidine ring resulting in formation of eight hydroxylated metabolites, and five of them showed an additional biotransformation (ester hydrolysis, N-oxide formation or glucuronidation) or were further oxidized to ketones. Three N-oxide metabolites were reported with minor abundances, formed by oxidation of either the piperidine nitrogen or the anilino-nitrogen linked in the amide moiety. In contrast to studies concerning the metabolism of other fentanyl analogs so far, no amide hydrolysis metabolites or hydroxy-methoxy metabolites have been reported in this study.
Alicyclic Fentanyl Analogs: Cyclopropylfentanyl, Cyclobutylfentanyl Cyclopentylfentanyl, Cyclohexylfentanyl and 2,2,3,3-Tetramethylcyclopropyl-Fentanyl
This subgroup of newly emerging fentanyl analogs structurally differs in the aliphatic amide linked moiety, which is substituted by an aliphatic cyclic moiety in these compounds. Concerning the receptor binding affinities and potencies only cyclopropylfentanyl has been evaluated so far. In vitro studies using chinese hamster ovary (CHO) and rat cell preparations expressing the three types of opioid receptors were used for determination of binding affinities. Cyclopropylfentanyl binds selectively to the μ-opioid receptor (vs. [3H]-DAMGO) with Ki values of 0.088 ± 0.027 nM for the μ-opioid receptor as well as 59.4 ± 3.0 nM and 36 ± 10 nM for the δ- and κ-opioid receptors, respectively. EC50 values were determined employing a [35S]GTPγS binding assay, resulting in 10.8 ± 2.7 nM for cyclopropylfentanyl at the μ-opioid receptor compared to 32 ± 11 nM for fentanyl, showing a more or less similar (about threefold higher) potency to fentanyl (Drug Enforcement Administration–Veterans Affairs (DEA-VA) Interagency Agreement, 2017; European Monitoring Centre for Drugs Drug Addiction [EMCDDA], 2018b). For the other alicyclic analogs no literature on receptor binding affinities and potencies is available yet. Theoretically, these analogs may imitate the steric requirements for receptor binding of fentanyl. They probably show similar or lower potency than fentanyl, in analogy to butyr- and valerylfentanyl which have been reported to be less potent.
A study investigating metabolism of this group of compounds has been published very recently by Åstrand et al. (2018) using human hepatocytes. Seven metabolites were identified for cyclopropylfentanyl, and the most abundant metabolite was norcyclopropylfentanyl formed by oxidative N-dealkylation. Other metabolic reactions observed were monohydroxylation, dihydroxylation followed by subsequent methylation, dihydrodiol and N-oxide formation. The glucuronic acid conjugate of the most intense hydroxy metabolite (hydroxylated at the piperidine ring) was detected as well. Hydroxylation of the cyclopropyl moiety or amide hydrolysis has not been detected in this study. The main metabolite norcyclopropylfentanyl has also been detected by Maher et al. (2018) in urine samples of two death cases and Palaty et al. (2018) in several urine samples of patients with a substance use disorder from two canadian provinces.
The major metabolites detected for cyclobutylfentanyl by Åstrand et al. (2018) were also N-dealkylation and, in contrast to cyclopropylfentanyl, hydroxylation of the cyclobutyl moiety and amide hydrolysis leading to a metabolite found in common with fentanyl, 4-ANPP. Further mono- and dihydroxylated metabolites were identified, mainly hydroxylated at the cyclobutyl moiety, the piperidine ring or the phenylethyl moiety.
In agreement with findings of cyclobutylfentanyl, the most abundant metabolites found were hydroxylations of the cyclopentyl moiety and nor-cyclopentylfentanyl. Moreover, another monohydroxlated metabolite (at the piperidine ring) and two monohydroxylated normetabolites (both at the cyclopentyl ring), a dihydroxylated metabolite (at the piperidine ring and the cyclopentyl ring), the amide hydrolysis product 4-ANPP and two further oxidation products (N-oxide and carbonyl metabolite) were formed to a minor extent in this assay.
Incubation of cyclohexylfentanyl with hepatocytes mainly led to the amide hydrolysis product 4-ANPP, norcyclohexylfentanyl and two monohydroxlated metabolites (both modified at the cyclohexyl moiety). Again, further hydroxylated metabolites were detected, comprising monohydroxlation, dihydroxylation, and hydroxylations in a second metabolic step primarily at the cyclohexyl and the piperidine ring.
Substitution of the amide linked alkyl chain with a 2,2,3,3-tetramethylcyclopropyl moiety seemed to steer metabolic reactions to this part of the molecule. Except for the nor-2,2,3,3-tetramathylcyclopropylfentanyl metabolite, which was formed to a minor extent, all metabolites showed at least one biotransformation of the 2,2,3,3-tetramethylcyclopropyl moiety. Monohydroxylations and dihydroxylations and subsequent further oxidation steps resulting in the formation of, e.g., carboxylic acids have been reported by Åstrand et al. (2018).
4-Fluoroisobutyrfentanyl (4F-iBF, Para-Fluoroisobutyrfentanyl)
4-fluoroisobutyrfentanyl (4-fluoro-isobutylfentanyl (N-(4-fluorophenyl)-2-methyl-N-[1-(2-phenylethyl)-4-piperidinyl]-propanamide) is one of the fluorinated fentanyl analogs that emerged on the NPS drug market recently. For this analog no data on binding affinity and selectivity to the μ-opioid receptor is available. The potency of 4F-iBF might be similar or lower than the potency of butyrfentanyl/isobutyrfentanyl, following the evaluation of fluorinated derivatives by Higashikawa and Suzuki (2008a). Metabolism of 4F-iBF was investigated by Watanabe et al. (2017) using hepatocyte incubates and analyzing authentic urine samples. In total, 17 metabolites were found and the following biotransformations were observed: N-dealkylation, monohydroxylations, dihydroxylations and subsequent methylation and glucuronidation, dihydrodiol formation, amide hydrolysis, carbonylation and carboxylation. Nine metabolites were identified in the hepatocyte assay. The most abundant ones were nor-4F-iBF, and two hydroxylated metabolites (at the piperidine ring or the phenylethyl moiety). Analysis of the urine samples after conjugate cleavage revealed 11 metabolites, resulting in a similar metabolic profile as obtained from the hepatocyte incubation assay, although the hydroxy-methoxy metabolite was more dominant in the authentic urine sample. Two additional glucuronic acid conjugates were detected when analyzing the urine without hydrolysis prior to extraction.
Furanylfentanyl
Binding affinity studies on furanylfentanyl have been performed in vitro using CHO and rat cell preparations expressing the three types of opioid receptors. Furanylfentanyl binds selectively to the μ-opioid receptor (vs. [3H]-DAMGO) with Ki values of 0.028 ± 0.008 nM for the μ-opioid receptor as well as 54 ± 15 nM and 59.2 ± 6.4 nM for the δ- and κ-opioid receptors, respectively. In vitro EC50 values were determined employing a [35S]GTPγS binding assay, resulting in 2.52 ± 0.46 nM for furanylfentanyl compared to 17.9 ± 4.3 nM for fentanyl at the μ-opioid receptor, suggesting a sevenfold higher potency for furanylfentanyl over fentanyl (Drug Enforcement Administration–Veterans Affairs (DEA-VA) Interagency Agreement, 2016; European Monitoring Centre for Drugs Drug Addiction [EMCDDA], 2017). In the patent literature of furanylfentanyl an in vivo ED50 value (0.02 mg/kg) was reported after i.v. administration to mice, but comparative data of fentanyl or morphine was not reported (Huang et al., 1986).
Goggin et al. (2017) identified four metabolites of furanylfentanyl after analyzing 500 urine samples of opioid intoxication cases. The most pronounced metabolites detected in 42 out of 51 cases positive for furanylfentanyl was the hydrolysis product 4-ANPP and its sulfate conjugate. Moreover, a very unique metabolite formed by dihydrodiol formation of the heterocyclic furanyl moiety was detected in 86% of the cases. The N-dealkylated metabolite norfuranylfentanyl was detected in only four of the furanylfentanyl positive cases indicating that substitution of the amide linked moiety of the fentanyl analogs to a furanyl-carboxamide shifts the metabolic profile of this compound toward a hydrolytic reaction and biotransformation of the furanyl moiety. In accordance with these findings, Mohr et al. (2016) detected 4-ANPP in five out of eight fatal intoxications with furanylfentanyl and Martucci et al. (2018) reported detection and distribution of the hydrolysis metabolite 4-ANPP in various tissues of a fatal furanylfentanyl intoxication case. In total, 17 and 14 phases I and II metabolites of furanylfentanyl were identified in a more detailed in vitro approach by Richeval et al. (2017) and Watanabe et al. (2017) using human liver microsomes and hepatocytes. In contrast to the findings of Goggins and Martucci, the spectrum of metabolic reactions in these in vitro studies comprised several hydroxylations, N-oxide formation and glucuronidation besides the already mentioned amide hydrolysis (plus sulfate conjugation), dihydrodiol formation and N-dealkylation. The most abundant in vitro metabolites reported by both authors were the hydrolysis product 4-ANPP, a dihydrodiol metabolite and norfuranylfentanyl. Additionally, a metabolite formed by oxidative ring-opening of the furanyl ring (and further oxidation to a carboxylic acid) was reported by both groups. Since metabolism of furanylfentanyl has been studied by a couple of research groups it can be said, that N-dealkylation which often leads to main metabolites of fentanyl and fentanyl analogs in vitro and in vivo, plays a minor role in the metabolism of furanylfentanyl, whereas amide hydrolysis and oxidative transformations of the furanyl moiety (such as dihydrodiol formation) are major biotransformation steps seen both in vitro and in vivo for this compound.
Methoxyacetylfentanyl
Methoxyacetylfentanyl is one of the numerous newly emerged fentanyl analogs differing from fentanyl by the modification of the N-acyl moiety. Structure activity relationships of methoxyacetylfentanyl and several other alkyloxy derivatives were investigated by Bagley et al. (1991) reporting an ED50 value of 0.053 mg/kg for methoxyacetylfentanyl. Compared to the ED50 of 0.018 mg/kg for fentanyl, about 30% of the potency of fentanyl can be assumed for this compound.
Metabolism of methoxyacetylfentanyl (2-methoxy-N-(1-phenethylpiperidin-4-yl)-N-phenylacetamide) was first examined in vitro using a human liver microsomal preparation (European Monitoring Centre for Drugs Drug Addiction [EMCDDA], 2018a). A main metabolic step for methoxyacetylfentanyl appears to be O-demethylation leading to 2-hydroxyacetamide metabolite. Further metabolic reactions were N-dealkylation, hydroxylations of the piperidine ring and the phenylethyl side chain, N-oxidation, as well as amide hydrolysis to 4-ANPP.
Mardal et al. (2018) investigated the in vitro and in vivo metabolic profiles of methoxyacetylfentanyl in the context of three case reports on deaths related to methoxyacetylfentanyl and by applying an additional in vitro study using human hepatocytes. A total of 10 methoxyacetylfentanyl metabolites were identified in hepatocyte incubates and biological samples. The metabolic pathways comprised mono- and dihydroxylations (at the phenylethyl ring or the anilinophenyl ring), N-dealkylation, O-demethylation, amide hydrolysis and combinations thereof as well as O-glucuronidation of the O-demethylated metabolite. The main metabolites both detected in vitro and in vivo were the O-demethylated metabolite and the hydrolysis product 4-ANPP. The findings of this study were consistent with unpublished data provided to the EMCDDA for risk assessment of methoxyacetylfentanyl (European Monitoring Centre for Drugs Drug Addiction [EMCDDA], 2018a).
Ocfentanil
This fentanyl analog has been developed and patented by Huang et al. (1986) and was evaluated for clinical application. Ocfentanil (N-(2-fluorophenyl)-2-methoxy-N-[1-(2-phenylethyl)-4-piperidinyl]acetamide) is the ortho-fluorinated analog of methoxyacetylfentanyl and has also been subject to the studies of Bagley et al. (1991). They determined an ED50 value of 0.0077 mg/kg for ocfentanil using the mouse hot plate test for evaluation of analgesic effects. Compared to fentanyl (0.018 mg/kg) the potency can be estimated to be about 2.5 times higher than for fentanyl. At the same time, ocfentanil showed less respiratory depression in animal studies. Fletcher et al. (1991) investigated dose-dependent pharmacologic effects in humans but were not able to draw conclusions from the study regarding a benefit of ocfentanil over fentanyl.
Allibe et al. (2018) performed metabolism studies on ocfentanil using human liver microsomes in addition to metabolism profiling in post mortem samples of a fatal intoxication case. Ocfentanil was found in all biological samples except nasal swab and concentrations were similar in peripheral blood and cardiac blood (Allibe et al., 2018). This observation is in contrast to results in two previously reported fatalities which observed significant deviations of the cardiac/peripheral blood concentration ratio (Coopman et al., 2016; Dussy et al., 2016).
Four metabolites were detected in vitro by Allibe et al. (2018) formed by hydroxylation (at the phenylethyl moiety), O-demethylation and combination of both reactions as well as the conjugation product of the O-demethyl metabolite with glucuronic acid. The main metabolite in vitro and in vivo clearly was O-demethyl ocfentanil, presumably even exceeding quantities of the parent compound (when comparing peak areas). In contrast, commonly seen biotransformations such as N-dealkylation and amide hydrolysis have not been detected in this work, suggesting that these metabolic reactions only play a minor role in metabolism of ocfentanil.
Ortho-Fluorofentanyl
Ortho-fluorofentanyl (N-(2-fluorophenyl)-N-[1-(2-phenylethyl)-4-piperidinyl]-propanamide) is a fluorinated fentanyl derivative. No data on receptor binding is available for this compound so far. However, the para-substituted analog was included in the studies performed by Higashikawa and Suzuki (2008a) and showed about 30% the potency of fentanyl determined by ED50 values. The LD50 values of 9.3 mg/kg for p-fluorofentanyl compared to 63 mg/kg for fentanyl indicate a higher toxicity of the fluorinated compound. The only study reporting metabolite identification of ortho-fluorofentanyl so far was a case report from Denmark by Andreasen et al. (2017). They detected the N-dealkylation product ortho-fluoro-norfentanyl in blood by HRMS techniques. Other potential metabolites like hydroxy-ortho-fluorofentanyl, hydroxy-ortho-fluoro-norfentanyl or the hydrolysis product ortho-fluoro-despropionylfentanyl were not detected in the authentic case sample. However, a urine sample was not part of the investigation, which could be the reason for the limited number of detected metabolites. The amide hydrolysis product ortho-fluoro-despropionylfentanyl has been reported to the EMCDDA as a fentanyl analog marketed independently, but further data on this compound is not available so far. A case report from Helland et al. (2017) focuses on the identification of ortho-fluorofentanyl and problems with the distinction of stereo-isomers. In this work the authors emphasize the necessity of integrating fluorinated analogs into general analytical screening procedures.
Tetrahydrofuranylfentanyl
The binding affinity of tetrahydrofuranylfentanyl (THFF, N-phenyl-N-[1-(2-phenylethyl)piperidin-4-yl]oxolane-2-carbo-xamide) was determined by the United States Drug Enforcement Administration (DEA) using CHO and rat cell preparations for opioid receptor expression. Ki values for THFF were 0.95 ± 0.32 nM (μ-OR vs. [3H]-DAMGO), compared to 741 ± 44 nM (vs. [3H]-U-69593) and 1,730 ± 260 nM (vs. [3H]-DPDPE) for the δ- and κ-opioid receptors, respectively, showing high selectively for the μ-opioid receptor. EC50 values were determined in vitro employing an [35S]GTPγS binding assay and resulted in 89 ± 16 nM for THFF at the μ-opioid receptor. The authors report a lower potency compared to fentanyl for this compound (European Monitoring Centre for Drugs Drug Addiction [EMCDDA], 2018c).
Data provided to the EMCDDA for risk assessment of THFF propose N-dealkylation to be the predominant metabolic step for THFF in human liver microsomal preparations, as in the case of fentanyl. Hydroxylation of the piperidine ring and the phenylethyl side chain, N-oxidation and amide hydrolysis to 4-ANPP were also observed (European Monitoring Centre for Drugs Drug Addiction [EMCDDA], 2018c).
Metabolic profiling of THFF was performed by Krotulski et al. (2018) to assist analytical identification of THFF in a fatality. Overall, seven metabolites were identified in vitro for THFF using pooled human liver microsomes. The hydroxylated metabolite species produced multiple, indistinguishable signals for hydroxylations at the tetrahydrofuranyl ring or the phenylethyl moiety. One of the major metabolites in vitro was nortetrahydrofuranylfentanyl formed by N-dealkylation, which proofed to be an applicable biomarker for THFF ingestion in biological samples. The hydroxylated species were also prominently detected in post mortem blood and urine samples. The hydrolysis product 4-ANPP was not unequivocally identified as a metabolite in this study (for analytical reasons), but may be considered as a possible minor metabolite since another hydroxylated metabolite (hydroxyl-4-ANPP) was also identified. Additionally, a biotransformation product presumably formed by oxidation of the tetrahydrofuranyl moiety and subsequent ‘internal hydrolysis’ under ring-opening was identified (Krotulski et al., 2018).
A short summary of the reviewed fentanyl analogs and their main metabolites (and metabolic pathways) described in the literature and estimated relative potencies (compared to fentanyl) are listed in Table 1.
Table 1
Summary of the reviewed fentanyl analogs and their metabolites and metabolic pathways.
Compounds | Detected metabolites (metabolic pathways) | Estimated relative potencies to fentanyl |
---|---|---|
Alfentanil | Noralfentanil (N-dealkylation) | Approximately 0.3 |
Sufentanil | Norsufentanil and N-phenylpropanamide (N-dealkylation), demethylsufentanil (O-demethylation), hydroxy metabolites | Approximately 10 |
Remifentanil | Remifentanil acid (ester hydrolysis) | Approximately 1 |
Acetylfentanyl | Acetyl norfentanyl (N-dealkylation), 4-ANPP (amide hydrolysis), β-hydroxyacetylfentanyl and further hydroxy metabolites, 4’-hydroxy-3’-methoxy-acetylfentanyl (dihydroxylation + methylation) and phase II conjugates | 0.3 |
Acryloylfentanyl | Acryloylnorfentanyl (N-dealkylation), 4-ANPP (amide hydrolysis), β-hydroxyacryloylfentanyl and further hydroxy metabolites, 4’-hydroxy-3’-methoxy-acryloylfentanyl (dihydroxylation + methylation) and phase II conjugates | Approximately 0.75 |
α-Methylfentanyl | Norfentanyl (N-dealkylation), Despropionyl-α-methylfentanyl (amide hydrolysis), alkyl/aryl hydroxy metabolites | Approximately 1 |
Cis-3-methylfentanyl Trans-3-methylfentanyl | Nor-3-methylfentanyl (N-dealkylation), alkyl/aryl hydroxy metabolites, carboxypropionyl-3-methylfentanyl (hydroxylation + oxidations), 4′-hydroxy-3′-methoxy-3-methylfentanyl (dihydroxylation + methylation) and phase II conjugates | 20 (+) isomer 0.2 (-) isomer Approximately 1 |
Isofentanyl | Nor-3-methylfentanyl (N-dealkylation), alkyl/aryl hydroxy metabolites, carboxypropionyl-isofentanyl (hydroxylation + oxidations), 4′-hydroxy-3′-methoxy-isofentanyl (dihydroxylation + methylation), N-oxide formation and phase II conjugates | n.a. |
Butyrfentanyl | Norbutyrfentanyl (N-dealkylation), carboxybutyrfentanyl (hydroxylation + oxidations), 4-ANPP (amide hydrolysis), alkyl/aryl hydroxy metabolites, 4′-hydroxy-3′-methoxy-butyrfentanyl (dihydroxylation + methylation), N-oxide formation and phase II conjugates | 0.03–0.13 |
Isobutyrfentanyl | n.a. | 0.13 |
Carfentanil | Norcarfentanil (N-dealkylation), alkyl/aryl hydroxy metabolites, carfentanil acid (ester hydrolysis), keto-carfentanil (hydroxylation + oxidation), N-oxide formation and phase II conjugates | 30–100 |
Cyclopropylfentanyl | Norcyclopropylfentanyl (N-dealkylation), hydroxylations, dihydrodiol and N-oxide formation | 3 |
Cyclobutylfentanyl | Norcyclobutylfentanyl (N-dealkylation), mainly alkyl hydroxy metabolites, 4-ANPP (amide hydrolysis), N-oxide and ketone formation | n.a. |
Cyclopentylfentanyl | Norcyclopentylfentanyl (N-dealkylation), mainly alkyl hydroxy metabolites, 4-ANPP (amide hydrolysis), N-oxide and ketone formation | n.a. |
Cyclohexylfentanyl | Norcyclohexylfentanyl (N-dealkylation), mainly alkyl hydroxy metabolites, 4-ANPP (amide hydrolysis), N-oxide and ketone formation | n.a. |
2,2,3,3-Tetramethyl-cyclopropylfentanyl | Mainly alkyl hydroxy metabolites, Nor-2,2,3,3-tetramethylcyclopropylfentanyl (N-dealkylation), carboxy-2,2,3,3-tetramethylcyclopropylfentanyl (hydroxylation + oxidations) | n.a. |
4-Fluoroisobutyrfentanyl | Nor-4-fluoroisobutyrfentanyl (N-dealkylation), alkyl/aryl hydroxy metabolites, 4-ANPP (amide hydrolysis), 4′-hydroxy-3′-methoxy-4-fluoroisobutyrfentanyl (dihydroxylation + methylation), dihydrodiol and ketone formation, carboxy-4-fluoroisobutyrfentanyl (hydroxylation + oxidations) and phase II conjugates | n.a. |
Furanylfentanyl | Furano-dihydrodiol formation, 4-ANPP (amide hydrolysis), norfuranylfentanyl (N-dealkylation), alkyl/aryl hydroxy metabolites, ring opening of the furanyl ring and phase II conjugates | 7 |
Methoxyacetylfentanyl | Demethylmethoxyacetylfentanyl (O-demethylation), 4-ANPP (amide hydrolysis), normethoxyacetylfentanyl (N-dealkylation), alkyl/aryl hydroxy metabolites and phase II conjugates | 0.3 |
Ocfentanil | Demethylocfentanil (O-demethylation), alkyl/aryl hydroxy metabolites and phase II conjugates | 2.5 |
Ortho-Fluorofentanyl | Nor-ortho-fluorofentanyl (N-dealkylation) | n.a. |
Tetrahydrofuranylfentanyl | Nortetrahydrofuranylfentanyl (N-dealkylation), alkyl/aryl hydroxy metabolites, ring opening of the tetrahydrofuranyl ring and 4-ANPP (amide hydrolysis) | Approximately 0.2 |
A number of further fentanyl analogs have been reported to the EMCDDA (mainly referring to seizures by police or customs authorities or intoxication cases) and were included into the literature search. However, no data on potency, receptor binding or metabolism was available yet. For the sake of completeness these compounds will be listed here in alphabetical order:
2-fluoroisobutyrfentanyl, 2-methyl-acetylfentanyl, 3-methyl-crotonylfentanyl (senecionyl fentanyl), 3-fluorofentanyl, 3-phenylpropanoylfentanyl, 4-chloroisobutyrfentanyl, 4-fluoro-butyrfentanyl, 4-fluoro-cyclopropylbenzylfentanyl, 4-fluoro-fentanyl, 4-fluoroisobutyrfentanyl N-benzyl analog, 4-methox-ybutyrfentanyl, α-methylfentanyl butanamide analog (2-methyl-N-phenyl-N-[1-(1-phenyl-propan-2-yl)piperidine-4-yl]propa-namide), acetyl benzylfentanyl, benzodioxolefentanyl, benzoyl-benzylfentanyl, benzoylfentanyl, benzylfentanyl, crotonyl-fentanyl, furanylbenzylfentanyl, furanylethylfentanyl, furanyl-fentanyl-3carboxamide isomer, thiophenefentanyl and valerylfentanyl.
Author Contributions
RS, SP, RP, AT, FB, VA, and MW searched for bibliographic material, drafted different chapter of the manuscript, and contributed substantially to manuscript intellectual content and revision.
Conflict of Interest Statement
The authors declare that the research was conducted in the absence of any commercial or financial relationships that could be construed as a potential conflict of interest.
Acknowledgments
The authors thank Mrs. Simonetta di Carlo, Mrs. Antonella Bacosi, Mrs. Laura Martucci, and Mr. Michele Sciotti. Oronzo Persano is acknowledged for technical assistance.
Footnotes
Funding. This work was supported by the European Union’s Programme – Drugs Policy Initiatives (grant agreement number: 806996-JUSTSO; call JUST-2017-AG-DRUG) and by the Department for Antidrug Policies, Presidency of the Council of Ministers, Italy.
References
- Alburges M. E., Hanson G. R., Gibb J. W., Sakashita C. O., Rollins D. E. (1992). Fentanyl receptor assay II. Utilization of a radioreceptor assay for the analysis of fentanyl analogs in urine. J. Anal. Toxicol. 16 36–41. 10.1093/jat/16.1.36 [Abstract] [CrossRef] [Google Scholar]
- Allibe N., Richeval C., Phanithavong M., Faure A., Allorge D., Paysant F., et al. (2018). Fatality involving ocfentanil documented by identification of metabolites. Drug Test. Anal. 10 995–1000. 10.1002/dta.2326 [Abstract] [CrossRef] [Google Scholar]
- Andreasen M. F., Hardlei T. F., Rosendal I., Thomsen A. H., Johannsen M., Saedder E. (2017). “A fatal poisoning involving 2-fluorofentanyl,” in Proceedings of the 55th SOFT-TIAFT 2017 Annual Meeting, Boca Raton, FL. [Google Scholar]
- Armenian P., Vo K. T., Barr-Walker J., Lynch K. L. (2018). Fentanyl, fentanyl analogs and novel synthetic opioids: a comprehensive review. Neuropharmacology 134 121–132. 10.1016/j.neuropharm.2017.10.016 [Abstract] [CrossRef] [Google Scholar]
- Åstrand A., Töreskog A., Watanabe S., Kronstrand R., Gréen H., Vikingsson S. (2018). Correlations between metabolism and structural elements of the alicyclic fentanyl analogs cyclopropyl fentanyl, cyclobutyl fentanyl, cyclopentyl fentanyl, cyclohexyl fentanyl and 2,2,3,3-tetramethylcyclopropyl fentanyl studied by human hepatocytes and LC-QTOF-MS. Arch. Toxicol. 93 95–106. 10.1007/s00204-018-2330-9 [Europe PMC free article] [Abstract] [CrossRef] [Google Scholar]
- Bagley J. R., Kudzma L. V., Lalinde N. L., Colapret J. A., Huang B.-S., Lin B.-S., et al. (1991). Evolution of the 4-anilidopiperidine class of opioid analgesics. Med. Res. Rev. 11 403–436. 10.1002/med.2610110404 [Abstract] [CrossRef] [Google Scholar]
- Banks H. D., Ferguson C. P. (1988). The Metabolites of Fentanyl and its Derivatives. Aberdeen, MD: Chemical Research Development & Engeneering Center. [Google Scholar]
- Bernard S. A., Bruera E. (2000). Drug interactions in palliative care. J. Clin. Oncol. 18 1780–1799. 10.1200/JCO.2000.18.8.1780 [Abstract] [CrossRef] [Google Scholar]
- Bespalov A., Müller R., Relo A.-L., Hudzik T. (2016). Drug tolerance: a known unknown in translational neuroscience. Trends Pharmacol. Sci. 37 364–378. 10.1016/j.tips.2016.01.008 [Abstract] [CrossRef] [Google Scholar]
- Bista S. R., Lobb M., Haywood A., Hardy J., Tapuni A., Norris R. (2014). Development, validation and application of an HPLC–MS/MS method for the determination of fentanyl and nor-fentanyl in human plasma and saliva. J. Chromatogr. B 960 27–33. 10.1016/j.jchromb.2014.04.019 [Abstract] [CrossRef] [Google Scholar]
- Bi-Yi C., Wen-Qiao J., Jie C., Xin-Jian C., You-Cheng Z., Zhi-Qiang C. (1999). Analgesic activity and selectivity of isothiocyanate derivatives of fentanyl analogs for opioid receptors. Life Sci. 65 1589–1595. 10.1016/S0024-3205(99)00404-X [Abstract] [CrossRef] [Google Scholar]
- Brine G. A., Stark P. A., Liu Y., Carroll F. I., Singh P., Xu H., et al. (1995). Enantiomers of diastereomeric cis-N-[1-(2-Hydroxy-2-phenylethyl)-3-methyl-4-piperidyl]-N-phenylpropanamides: synthesis, X-ray analysis, and biological activities. J. Med. Chem. 38 1547–1557. 10.1021/jm00009a015 [Abstract] [CrossRef] [Google Scholar]
- Cascone S., Lamberti G., Piazza O., Abbiati R. A., Manca D. (2018). A physiologically-based model to predict individual pharmacokinetics and pharmacodynamics of remifentanil. Eur. J. Pharm. Sci. 111 20–28. 10.1016/j.ejps.2017.09.028 [Abstract] [CrossRef] [Google Scholar]
- Coopman V., Cordonnier J., De Leeuw M., Cirimele V. (2016). Ocfentanil overdose fatality in the recreational drug scene. Forensic Sci. Int. 266(Suppl. C), 469–473. 10.1016/j.forsciint.2016.07.005 [Abstract] [CrossRef] [Google Scholar]
- Costa E. M., Hoffman B. B., Loew G. H. (1992). Opioid agonists binding and responses in SH-SY5Y cells. Life Sci. 50 73–81. 10.1016/0024-3205(92)90199-Y [Abstract] [CrossRef] [Google Scholar]
- DePriest A. Z., Puet B. L., Holt A. C., Roberts A., Cone E. J. (2015). Metabolism and disposition of prescription opioids: a review. Forensic Sci. Rev. 27 115–145. [Abstract] [Google Scholar]
- Donk T., Ward S., Langford R., Dahan A. (2018). Pharmacokinetics and pharmacodynamics of sublingual sufentanil for postoperative pain management. Anaesthesia 73 231–237. 10.1111/anae.14132 [Abstract] [CrossRef] [Google Scholar]
- Drug Enforcement Administration–Veterans Affairs (DEA-VA) Interagency Agreement (2017). N-(1-Phenethylpiperidin-4-yl)-N-phenylcyclopropanecarboxamide, HCl Binding and Functional Activity at Delta, Kappa and Mu Opioid Receptors. In Vitro Receptor and Transporter Assays for Abuse Liability Testing for the DEA by the VA. Springfield, VA: Drug Enforcement Administration. [Google Scholar]
- Drug Enforcement Administration–Veterans Affairs (DEA-VA) Interagency Agreement (2016). N-phenyl-N-[1-(2-phenylethyl)-4-piperidinyl]-2-furancar-boxamide, mononhydrochloride Binding and Functional Activity at Delta, Kappa and Mu Opioid Receptors. In Vitro Receptor and Transporter Assays for Abuse Liability Testing for the DEA by the VA. Springfield, VA: Drug Enforcement Administration. [Google Scholar]
- Dussy F. E., Hangartner S., Hamberg C., Berchtold C., Scherer U., Schlotterbeck G., et al. (2016). An acute ocfentanil fatality: a case report with postmortem concentrations. J. Anal. Toxicol. 40 761–766. 10.1093/jat/bkw096 [Abstract] [CrossRef] [Google Scholar]
- Essawi M. Y. (1999). Fentanyl analogues with a modified propanamido group as potential affinity labels: synthesis and in vivo activity. Die Pharmazie 54 307–308. [Abstract] [Google Scholar]
- European Monitoring Centre for Drugs Drug Addiction [EMCDDA] (2017). Report on the Risk Assessment of N-phenyl-N-[1-(2-phenylethyl)piperidin-4-yl]-furan-2-Carboxamide (furanylfentanyl) in the Framework of the Council Decision on New Psychoactive Substances, Risk Assessments. Luxembourg: Publications Office of the European Union. [Google Scholar]
- European Monitoring Centre for Drugs Drug Addiction [EMCDDA] (2018a). EMCDDA–Europol Joint Report on a New Psychoactive Substance: 2-Methoxy-N-Phenyl-N-[1-(2-phenylethyl) Piperidin-4-yl]Acetamide (Methoxyacetylfentanyl), Joint Reports. Luxembourg: Publications Office of the European Union. [Google Scholar]
- European Monitoring Centre for Drugs Drug Addiction [EMCDDA] (2018b). Report on the Risk Assessment of N-phenyl-N-[1-(2-phenylethyl)piperidin-4-yl]Cyclopropanecarboxamide (cyclopropylfentanyl) in the Framework of the Council Decision on New Psychoactive Substances, Risk Assessments. Luxembourg: Publications Office of the European Union. [Google Scholar]
- European Monitoring Centre for Drugs Drug Addiction [EMCDDA] (2018c). Report on the Risk Assessment of N-phenyl-N-[1-(2-phenylethyl)piperidin-4-yl]oxolane-2-carboxamide (tetrahydrofuranylfentanyl; THF-F) in the framework of the Council Decision on new Psychoactive substances, Risk Assessments. Luxembourg: Publications Office of the European Union. [Google Scholar]
- Feasel M. G., Wohlfarth A., Nilles J. M., Pang S., Kristovich R. L., Huestis M. A. (2016). Metabolism of carfentanil, an ultra-potent opioid, in human liver microsomes and human hepatocytes by high-resolution mass spectrometry. AAPS J. 18 1489–1499. 10.1208/s12248-016-9963-5 [Abstract] [CrossRef] [Google Scholar]
- Feierman D. E., Lasker J. M. (1996). Metabolism of fentanyl, a synthetic opioid analgesic, by human liver microsomes. Role of CYP3A4. Drug Metab. Dispos. 24 932–939. [Abstract] [Google Scholar]
- Fletcher J. E., Sebel P. S., Murphy M. R., Mick S. A., Fein S. (1991). Comparison of ocfentanil and fentanyl as supplements to general anesthesia. Anesth. Analg. 73 622–626. 10.1213/00000539-199111000-00019 [Abstract] [CrossRef] [Google Scholar]
- Fragen R. J., Booij L. H. D. J., Braak G. J. J., Vree T. B., Heykants J., Crul J. F. (1983). Pharmacokinetics of the infusion of alfentanil in man. Br. J. Anaesth. 55 1077–1081. 10.1093/bja/55.11.1077 [Abstract] [CrossRef] [Google Scholar]
- France C. P., Gerak L. R., Flynn D., Winger G. D., Medzihradsky F., Bagley J. R., et al. (1995). Behavioral effects and receptor binding affinities of fentanyl derivatives in rhesus monkeys. J. Pharmacol. Exp. Ther. 274 17–28. [Abstract] [Google Scholar]
- Gillespie T. J., Gandolfi A. J., Davis T. P., Morano R. A. (1982). Identification and quantification of alpha-methylfentanyl in post mortem specimens. J. Anal. Toxicol. 6 139–142. 10.1093/jat/6.3.139 [Abstract] [CrossRef] [Google Scholar]
- Goggin M. M., Nguyen A., Janis G. C. (2017). Identification of unique metabolites of the designer opioid furanyl fentanyl. J. Anal. Toxicol. 41 367–375. 10.1093/jat/bkx022 [Abstract] [CrossRef] [Google Scholar]
- Goromaru P. D. T., Matsuura P. D. H., Yoshimura M. D. N., Miyawaki M. D. T., Sameshima B. A. T., Miyao B. A. J., et al. (1984). Identification and quantitative determination of fentanyl metabolites in patients by gas chromatography-mass spectrometry anesthesiology. Anesthesiology 61 73–77. 10.1097/00000542-198407000-00013 [Abstract] [CrossRef] [Google Scholar]
- Gudin J. (2012). Opioid therapies and cytochrome P450 interactions. J. Pain Symptom Manage 44 S4–S14. 10.1016/j.jpainsymman.2012.08.013 [Abstract] [CrossRef] [Google Scholar]
- Guitton J., Buronfosse T., Désage M., Lepape A., Brazier J.-L., Beaune P. (1997). Possible involvement of multiple cytochrome P450S in fentanyl and sufentanil metabolism as opposed to alfentanil. Biochem. Pharmacol. 53 1613–1619. 10.1016/S0006-2952(96)00893-3 [Abstract] [CrossRef] [Google Scholar]
- Hammargren W. R., Henderson G. L. (1988). Analyzing normetabolites of the fentanyls by gas chromatography/electron capture detection. J. Anal. Toxicol. 12 183–191. 10.1093/jat/12.4.183 [Abstract] [CrossRef] [Google Scholar]
- Helland A., Brede W. R., Michelsen L. S., Gundersen P. O. M., Aarset H., Skjølås J. E., et al. (2017). Two hospitalizations and one death after exposure to ortho-fluorofentanyl. J. Anal. Toxicol. 41 708–709. 10.1093/jat/bkx050 [Abstract] [CrossRef] [Google Scholar]
- Henriksen G., Platzer S., Marton J., Hauser A., Berthele A., Schwaiger M., et al. (2005). Syntheses, biological evaluation, and molecular modeling of 18f-labeled 4-anilidopiperidines as μ-opioid receptor imaging agents. J. Med. Chem. 48 7720–7732. 10.1021/jm0507274 [Abstract] [CrossRef] [Google Scholar]
- Higashikawa Y., Suzuki S. (2008a). Studies on 1-(2-Phenethyl)-4-(N-propionylanilino)piperidine (fentanyl) and its related compounds: novel metabolites in rat urine following injection of alpha-methylfentanyl, one of the most abused typical designer drugs. J. Health Sci. 54 629–637. 10.1248/jhs.54.629 [CrossRef] [Google Scholar]
- Higashikawa Y., Suzuki S. (2008b). Studies on 1-(2-phenethyl)-4-(N-propionylanilino)piperidine (fentanyl) and its related compounds. VI. Structure-analgesic activity relationship for fentanyl, methyl-substituted fentanyls and other analogues. Forensic Toxicol. 26 1–5. 10.1007/s11419-007-0039-1 [CrossRef] [Google Scholar]
- Huang B.-S., Terrell R. C., Deutsche K. H., Kudzma L. V., Lalinde N. L. (1986). N-aryl-N-(4-piperidinyl)amides and pharmaceutical compositions and method employing such compounds. U.S. Patent No 4584303A. New Providence, NJ: Anaquest Inc. [Google Scholar]
- Ingoglia N. A., Dole V. P. (1970). Localization of d and l methadone after interventricular injection into rat brains. J. Pharmacol. Exp. Ther. 175 84–87. [Abstract] [Google Scholar]
- Janssen P. A. (1965). 1-aralkyl-4-(n-aryl-carbonyl amino)-piperidines and related compounds. U.S. Patent No 5100903A. Madison, WI: Datex Ohmeda Inc. [Google Scholar]
- Janssen P. A. (1979). N-(4-piperidinyl)-N-phenylamides. U.S. Patent No 4179569A. Beerse: Janssen Pharmaceutica NV. [Google Scholar]
- Jewett D. M., Kilbourn M. R. (2004). In vivo evaluation of new carfentanil-based radioligands for the mu opiate receptor. Nuclear Med. Biol. 31 321–325. 10.1016/j.nucmedbio.2003.10.009 [Abstract] [CrossRef] [Google Scholar]
- Jiang H. L., Huang X. Q., Rong S. B., Luo X. M., Chen J. Z., Tang Y., et al. (2000). Theoretical studies on opioid receptors and ligands. I. Molecular modeling and QSAR studies on the interaction mechanism of fentanyl analogs binding to μ-opioid receptor. Int. J. Quant. Chem. 78 285–293. 10.1002/(SICI)1097-461X(2000)78:4<285::AID-QUA11>3.0.CO;2-I [CrossRef] [Google Scholar]
- Kanamori T., Togawa Iwata Y., Segawa H., Yamamuro T., Kuwayama K., Tsujikawa K., et al. (2018a). Metabolism of fentanyl and acetylfentanyl in human-induced pluripotent stem cell-derived hepatocytes. Biol. Pharm. Bull. 41 106–114. 10.1248/bpb.b17-00709 [Abstract] [CrossRef] [Google Scholar]
- Kanamori T., Togawa-Iwata Y., Segawa H., Yamamuro T., Kuwayama K., Tsujikawa K., et al. (2018b). Use of hepatocytes isolated from a liver-humanized mouse for studies on the metabolism of drugs: application to the metabolism of fentanyl and acetylfentanyl. Forensic Toxicol. 36 467–475. 10.1007/s11419-018-0425-x [Europe PMC free article] [Abstract] [CrossRef] [Google Scholar]
- Kokubun H., Ebinuma K., Matoba M., Takayanagi R., Yamada Y., Yago K. (2012). Population pharmacokinetics of transdermal fentanyl in patients with cancer-related pain. J. Pain Palliat. Care Pharmacother. 26 98–104. 10.3109/15360288.2012.679725 [Abstract] [CrossRef] [Google Scholar]
- Koyyalagunta D. (2007). “Opioid analgesics,” in Pain Management, ed. Waldmann S.D. Saunders: Philadelphia, PA, 939–964. 10.1016/B978-0-7216-0334-6.50117-5 [CrossRef] [Google Scholar]
- Krotulski A. J., Papsun D. M., Friscia M., Swartz J. L., Holsey B. D., Logan B. K. (2018). Fatality following ingestion of tetrahydrofuranylfentanyl, U-49900 and methoxy-phencyclidine. J. Anal. Toxicol. 42 e27–e32. 10.1093/jat/bkx092 [Abstract] [CrossRef] [Google Scholar]
- Kuip E. J. M., Zandvliet M. L., Koolen S. L. W., Mathijssen R. H. J., Rijt C. C. D. (2017). A review of factors explaining variability in fentanyl pharmacokinetics; focus on implications for cancer patients. Br. J. Clin. Pharmacol. 83 294–313. 10.1111/bcp.13129 [Europe PMC free article] [Abstract] [CrossRef] [Google Scholar]
- Kukanich B., Papich M. G. (2009). “Opioid analgesic drugs,” in Veterinary Pharmacology and Therapeutics, 9th Edn, eds Riviere J. E., Papich M. G., Adams H. R. (Hoboken, NJ: Wiley-Blackwell; ), 301–337. [Google Scholar]
- Labroo R. B., Paine M. F., Thummel K. E., Kharasch E. D. (1997). Fentanyl metabolism by human hepatic and intestinal cytochrome P450 3A4: implications for interindividual variability in disposition, efficacy, and drug interactions. Drug Metab. Dispos. 25 1072–1080. [Abstract] [Google Scholar]
- Lavrijsen K., Van Houdt J., Van Dyck D., Hendrickx J., Lauwers W., Hurkmans R., et al. (1990). Biotransformation of sufentanil in liver microsomes of rats, dogs, and humans. Drug Metab. Dispos. 18 704–710. [Abstract] [Google Scholar]
- Maguire P., Tsai N., Kamal J., Cometta-Morini C., Upton C., Loew G. (1992). Pharmacological profiles of fentanyl analogs at μ, δ and κ opiate receptors. Eur. J. Pharmacol. 213 219–225. 10.1016/0014-2999(92)90685-W [Abstract] [CrossRef] [Google Scholar]
- Maher S., Elliott S. P., George S. (2018). The analytical challenges of cyclopropylfentanyl and crotonylfentanyl: an approach for toxicological analysis. Drug Test. Anal. 10 1483–1487. 10.1002/dta.2417 [Abstract] [CrossRef] [Google Scholar]
- Mahlke N. S., Ziesenitz V., Mikus G., Skopp G. (2014). Quantitative low-volume assay for simultaneous determination of fentanyl, norfentanyl, and minor metabolites in human plasma and urine by liquid chromatography—tandem mass spectrometry (LC-MS/MS). Int. J. Legal Med. 128 771–778. 10.1007/s00414-014-1040-y [Abstract] [CrossRef] [Google Scholar]
- Marchei E., Pacifici R., Mannocchi G., Marinelli E., Busardò F. P., Pichini S. (2018). New synthetic opioids in biological and non-biological matrices: A review of current analytical methods. Trends Analyt. Chem. 102 1–15. 10.1016/j.trac.2018.01.007 [CrossRef] [Google Scholar]
- Mardal M., Johansen S. S., Davidsen A. B., Telving R., Jornil J. R., Dalsgaard P. W., et al. (2018). Postmortem analysis of three methoxyacetylfentanyl-related deaths in Denmark and in vitro metabolite profiling in pooled human hepatocytes. Forensic Sci. Int. 290 310–317. 10.1016/j.forsciint.2018.07.020 [Abstract] [CrossRef] [Google Scholar]
- Martin W. R. (1967). Opioid antagonists. Pharmacol. Rev. 19 463–521. [Abstract] [Google Scholar]
- Martucci H. F. H., Ingle E. A., Hunter M. D., Rodda L. N. (2018). Distribution of furanyl fentanyl and 4-ANPP in an accidental acute death: a case report. Forensic Sci. Int. 283 e13–e17. 10.1016/j.forsciint.2017.12.005 [Abstract] [CrossRef] [Google Scholar]
- Maryanoff B. E., Simon E. J., Gioannini T., Gorissen H. (1982). Potential affinity labels for the opiate receptor based on fentanyl and related compounds. J. Med. Chem. 25 913–919. 10.1021/jm00350a006 [Abstract] [CrossRef] [Google Scholar]
- Melent’ev A. B., Kataev S. S., Dvorskaya O. N. (2015). Identification and analytical properties of acetyl fentanyl metabolites. J. Anal. Chem. 70 240–248. 10.1134/S1061934815020124 [CrossRef] [Google Scholar]
- Mercadante S. (2015). Opioid metabolism and clinical aspects. Eur. J. Pharmacol. 769 71–78. 10.1016/j.ejphar.2015.10.049 [Abstract] [CrossRef] [Google Scholar]
- Meyer M. R., Dinger J., Schwaninger A. E., Wissenbach D. K., Zapp J., Fritschi G., et al. (2012). Qualitative studies on the metabolism and the toxicological detection of the fentanyl-derived designer drugs 3-methylfentanyl and isofentanyl in rats using liquid chromatography–linear ion trap–mass spectrometry (LC-MSn). Anal. Bioanal. Chem. 402 1249–1255. 10.1007/s00216-011-5528-8 [Abstract] [CrossRef] [Google Scholar]
- Mohr A. L. A., Friscia M., Papsun D., Kacinko S. L., Buzby D., Logan B. K. (2016). Analysis of novel synthetic opioids U-47700, U-50488 and furanyl fentanyl by LC–MS/MS in postmortem casework. J. Anal. Toxicol. 40 709–717. 10.1093/jat/bkw086 [Abstract] [CrossRef] [Google Scholar]
- National Institute on Drug Abuse [NIDA] (2016). Drug Facts Fentanyl. National Institutes of Health; U.S. Department of Health and Human Services. Available at: https://www.drugabuse.gov/publications/drugfacts/fentanyl [Google Scholar]
- Palaty J., Konforte D., Karakosta T., Wong E., Stefan C. (2018). Rapid identification of cyclopropyl fentanyl/crotonyl fentanyl in clinical urine specimens: a case study of clinical laboratory collaboration in Canada. Clin. Biochem. 53 164–167. 10.1016/j.clinbiochem.2018.01.013 [Abstract] [CrossRef] [Google Scholar]
- Pan L., Xu J., Yu R., Xu M. M., Pan Y. X., Pasternak G. W. (2005). Identification and characterization of six new alternatively spliced variants of the human μ opioid receptor gene, Oprm. Neuroscience 133 209–220. 10.1016/j.neuroscience.2004.12.033 [Abstract] [CrossRef] [Google Scholar]
- Panzer O., Moitra V., Sladen R. N. (2009). Pharmacology of sedative-analgesic agents: dexmedetomidine, remifentanil, ketamine, volatile anesthetics, and the role of peripheral Mu antagonists. Crit. Care Clin. 25 451–469. 10.1016/j.ccc.2009.04.004 [Abstract] [CrossRef] [Google Scholar]
- Patton A. L., Seely K. A., Pulla S., Rusch N. J., Moran C. L., Fantegrossi W. E., et al. (2014). Quantitative measurement of acetyl fentanyl and acetyl norfentanyl in human urine by LC-MS/MS. Anal. Chem. 86 1760–1766. 10.1021/ac4036197 [Abstract] [CrossRef] [Google Scholar]
- Pert C. B., Snyder S. H. (1973). Opiate receptor: demonstration in nervous tissue. Science 179 1011–1014. 10.1126/science.179.4077.1011 [Abstract] [CrossRef] [Google Scholar]
- Pichini S., Pacifici R., Marinelli E., Busardò F. P. (2017). European Drug Users at Risk from Illicit Fentanyls Mix. Front. Pharmacol. 8:785. 10.3389/fphar.2017.00785 [Europe PMC free article] [Abstract] [CrossRef] [Google Scholar]
- Pichini S., Solimini R., Berretta P., Pacifici R., Busardò F. P. (2018). Acute intoxications and fatalities from illicit fentanyl and analogues: an update. Ther. Drug Monit. 40 38–51. 10.1097/FTD.0000000000000465 [Abstract] [CrossRef] [Google Scholar]
- Poonyachoti S., Portoghese P. S., Brown D. R. (2001). Pharmacological evidence for a 7-benzylidenenaltrexone-preferring opioid receptor mediating the inhibitory actions of Peptidic δ- and μ-Opioid agonists on neurogenic ion transport in porcine ileal mucosa. J. Pharmacol. Exp. Ther. 297 672–679. [Abstract] [Google Scholar]
- Portoghese P. S., Lunzer M. M. (2003). Identity of the putative δ1-opioid receptor as a δ–κ heteromer in the mouse spinal cord. Eur. J. Pharmacol. 467 233–234. 10.1016/S0014-2999(03)01599-1 [Abstract] [CrossRef] [Google Scholar]
- Riches J. R., Read R. W., Black R. M., Cooper N. J., Timperley C. M. (2012). Analysis of clothing and urine from moscow theatre siege casualties reveals carfentanil and remifentanil use. J. Anal. Toxicol. 36 647–656. 10.1093/jat/bks078 [Abstract] [CrossRef] [Google Scholar]
- Richeval C., Gicquel T., Hugbart C., Le Dare B., Allorge D., Morel I., et al. (2017). In vitro characterization of NPS metabolites produced by human liver microsomes and the HepaRG cell line using liquid chromatographyhigh resolution mass spectrometry (LC-HRMS) analysis: application to furanyl fentanyl. Curr. Pharm. Biotechnol. 18 806–814. 10.2174/1389201018666171122124401 [Abstract] [CrossRef] [Google Scholar]
- Rosow C. E. (1999). An overview of remifentanil. Anesth. Analg. 89 S1–S3. 10.1097/00000539-199910001-00001 [Abstract] [CrossRef] [Google Scholar]
- Rothberg R. L., Stith K. (2018). Fentanyl: a whole new world? J. Law Med. Ethics 46 314–324. 10.1177/1073110518782937 [Abstract] [CrossRef] [Google Scholar]
- Rothman R. B., France C. P., Bykov V., De Costa B. R., Jacobson A. E., Woods J. H., et al. (1989). Pharmacological activities of optically pure enantiomers of the κ opioid agonist, U50,488, and its cis diastereomer: evidence for three κ receptor subtypes. Eur. J. Pharmacol. 167 345–353. 10.1016/0014-2999(89)90443-3 [Abstract] [CrossRef] [Google Scholar]
- Sato S., Suzuki S., Lee X.-P., Sato K. (2010). Studies on 1-(2-phenethyl)-4-(N-propionylanilino)piperidine (fentanyl) and related compounds: VII. Quantification of α-methylfentanyl metabolites excreted in rat urine. Forensic Sci. Int. 195 68–72. 10.1016/j.forsciint.2009.11.014 [Abstract] [CrossRef] [Google Scholar]
- Schneider E., Brune K. (1986). Opioid activity and distribution of fentanyl metabolites. Naunyn. Schmiedebergs Arch. Pharmacol. 334 267–274. 10.1007/BF00508781 [Abstract] [CrossRef] [Google Scholar]
- Simon E. J., Hiller J. M., Edelman I. (1973). Stereospecific binding of the potent narcotic analgesic [3H]etorphine to rat-brain homogenate. Proc. Natl. Acad. Sci. U.S.A. 70 1947–1949. 10.1073/pnas.70.7.1947 [Europe PMC free article] [Abstract] [CrossRef] [Google Scholar]
- Skulska A., Kała M., Parczewski A. (2004). Fentanyl and its analogues in the forensic laboratory. Medical and analytical problems. Probl. Forensic. Sci. 59 127–142. [Google Scholar]
- Solassol I., Bressolle F., Caumette L., Garcia F., Poujol S., Culine S., et al. (2005). Inter- and intraindividual variabilities in pharmacokinetics of fentanyl after repeated 72-hour transdermal applications in cancer pain patients. Ther. Drug Monit. 27 491–498. 10.1097/01.ftd.0000160717.50704.42 [Abstract] [CrossRef] [Google Scholar]
- Staeheli S. N., Baumgartner M. R., Gauthier S., Gascho D., Jarmer J., Kraemer T., et al. (2016). Time-dependent postmortem redistribution of butyrfentanyl and its metabolites in blood and alternative matrices in a case of butyrfentanyl intoxication. Forensic Sci. Int. 266 170–177. 10.1016/j.forsciint.2016.05.034 [Abstract] [CrossRef] [Google Scholar]
- Steuer A. E., Williner E., Staeheli S. N., Kraemer T. (2017). Studies on the metabolism of the fentanyl-derived designer drug butyrfentanyl in human in vitro liver preparations and authentic human samples using liquid chromatography-high resolution mass spectrometry (LC-HRMS). Drug Test. Anal. 9 1085–1092. 10.1002/dta.2111 [Abstract] [CrossRef] [Google Scholar]
- Streisand J. B., Varvel J. R., Stanski D. R., Le Maire L., Ashburn M. A., Hague B. I., et al. (1991). Absorption and bioavailability of oral transmucosal fentanyl citrate. Anesthesiology 75 223–229. 10.1097/00000542-199108000-00009 [Abstract] [CrossRef] [Google Scholar]
- Subramanian G., Paterlini M. G., Portoghese P. S., Ferguson D. M. (2000). Molecular docking reveals a novel binding site model for fentanyl at the μ-Opioid receptor. J. Med. Chem. 43 381–391. 10.1021/jm9903702 [Abstract] [CrossRef] [Google Scholar]
- Tateishi T., Krivoruk Y., Ueng Y.-F., Wood A. J. J., Guengerich F. P., Wood M. (1996). Identification of human liver cytochrome P-450 3A4 as the enzyme responsible for fentanyl and sufentanil N-dealkylation. Anesth. Analg. 82 167–172. [Abstract] [Google Scholar]
- Terenius L. (1973). Characteristics of the “receptor” for narcotic analgesics in synaptic plasma membrane fraction from rat Brain. Acta Pharmacol. Toxicol. 33 377–384. 10.1111/j.1600-0773.1973.tb01539.x [Abstract] [CrossRef] [Google Scholar]
- Thompson R. G., Menking D., Valdes J. J. (1987). Opiate Receptor Binding Properties of Carfentanil. Baltimore, MD: Chemical Research, Development & Engineering Center; 10.21236/ADA187637 [CrossRef] [Google Scholar]
- Ujváry I., Jorge R., Christie R., Le Ruez T., Danielsson H. V., Kronstrand R., et al. (2017). Acryloylfentanyl, a recently emerged new psychoactive substance: a comprehensive review. Forensic Toxicol. 35 232–243. 10.1007/s11419-017-0367-8 [CrossRef] [Google Scholar]
- United Nations Office on Drugs and Crime [UNODC] (2017). Recommended Methods for the Identification and Analysis of Fentanyl and its Analogues in Biological Specimen. Vienna: UNODC [Google Scholar]
- US Drug Enforcement Administration [US DEA] (2015) National Drug Threat Assessment. Report DEA-DCT-DIR-008-16; October 2015 Available at: https://www.dea.gov/sites/default/files/2018-07/2015\%20NDTA\%20Report.pdf [Google Scholar]
- Valaer A. K., Huber T., Andurkar S. V., Clark C. R., DeRuiter J. (1997). Development of a gas chromatographic—mass spectrometric drug screening method for the n-dealkylated metabolites of fentanyl, Sufentanil, and Alfentanil. J. Chromatogr. Sci. 35 461–466. 10.1093/chromsci/35.10.461 [Abstract] [CrossRef] [Google Scholar]
- Van Bever W. F., Niemegeers C. J., Schellekens K. H., Janssen P. A. (1976). N-4-Substituted 1-(2-arylethyl)-4-piperidinyl-N-phenylpropanamides, a novel series of extremely potent analgesics with unusually high safety margin. Arzneimittelforschung 26 1548–1551. [Abstract] [Google Scholar]
- Van Bever W. F. M., Niemegeers C. J. E., Janssen P. A. J. (1974). Synthetic analgesics. Synthesis and pharmacology of the diastereoisomers of N-[3-methyl-1-(2-phenylethyl)-4-piperidyl]-N-phenylpropanamide and N-[3-methyl-1-(1-methyl-2-phenylethyl)-4-piperidyl]-N-phenylpropanamide. J. Med. Chem. 17 1047–1051. 10.1021/jm00256a003 [Abstract] [CrossRef] [Google Scholar]
- Van Daele P. G., De Bruyn M. F., Boey J. M., Sanczuk S., Agten J. T., Janssen P. A. (1976). Synthetic analgesics: N-(1-[2-arylethyl]-4-substituted 4-piperidinyl) N-arylalkanamides. Arzneimittelforschung 26 1521–1531. [Abstract] [Google Scholar]
- Van Praag D., Simon E. J. (1966). Studies on the intracellular distribution and tissue binding of dihydromorphine-7,8-H3 in the rat. Proc. Soc. Exp. Biol. Med. 122 6–11. 10.3181/00379727-122-31036 [Abstract] [CrossRef] [Google Scholar]
- Van Wijngaarden I., Soudijn W. (1968). The metabolism and excretion of the analgesic fentanyl (R 4263) by wistar rats. Life Sci. 7 1239–1244. 10.1016/0024-3205(68)90236-1 [Abstract] [CrossRef] [Google Scholar]
- Vardanyan R. S., Hruby V. J. (2014). Fentanyl-related compounds and derivatives: current status and future prospects for pharmaceutical applications. Fut. Med. Chem. 6 385–412. 10.4155/fmc.13.215 [Europe PMC free article] [Abstract] [CrossRef] [Google Scholar]
- Villemagne V. L., James Frost J., Dannals R. F., Lever J. R., Tanada S., Natarajan T. K., et al. (1994). Comparison of [11C]diprenorphine and [11C]carfentanil in vivo binding to opiate receptors in man using a dual detector system. Eur. J. Pharmacol. 257 195–197. 10.1016/0014-2999(94)90712-9 [Abstract] [CrossRef] [Google Scholar]
- Volpe D. A., Tobin G. A. M., Mellon R. D., Katki A. G., Parker R. J., Colatsky T., et al. (2011). Uniform assessment and ranking of opioid Mu receptor binding constants for selected opioid drugs. Regul. Toxicol. Pharmacol. 59 385–390. 10.1016/j.yrtph.2010.12.007 [Abstract] [CrossRef] [Google Scholar]
- Vuckovic S., Prostran M., Ivanovic M., Dosen-Micovic L., Todorovic Z., Nesic Z., et al. (2009). Fentanyl analogs: structure-activity-relationship study. Curr. Med. Chem. 16 2468–2474. 10.2174/092986709788682074 [Abstract] [CrossRef] [Google Scholar]
- Wang Z.-X., Zhu Y.-C., Jin W.-Q., Chen X.-J., Chen J., Ji R.-Y., et al. (1995). Stereoisomers of N-[1-(2-Hydroxy-2-phenylethyl)-3-methyl-4-piperidyl]- N-phenylpropanamide: synthesis, stereochemistry, analgesic activity, and opioid receptor binding characteristics. J. Med. Chem. 38 3652–3659. 10.1021/jm00018a026 [Abstract] [CrossRef] [Google Scholar]
- Watanabe S., Vikingsson S., Roman M., Green H., Kronstrand R., Wohlfarth A. (2017). In vitro and in vivo metabolite identification studies for the new synthetic opioids acetylfentanyl, acrylfentanyl, furanylfentanyl, and 4-fluoro-isobutyrylfentanyl. AAPS J. 19 1102–1122. 10.1208/s12248-017-0070-z [Abstract] [CrossRef] [Google Scholar]
- Wu F., Slawson M. H., Johnson-Davis K. L. (2017). Metabolic patterns of fentanyl, meperidine, methylphenidate, tapentadol and tramadol observed in urine, serum or plasma. J. Anal. Toxicol. 41 289–299. 10.1093/jat/bkx003 [Abstract] [CrossRef] [Google Scholar]
Articles from Frontiers in Pharmacology are provided here courtesy of Frontiers Media SA
Full text links
Read article at publisher's site: https://doi.org/10.3389/fphar.2019.00238
Read article for free, from open access legal sources, via Unpaywall:
https://www.frontiersin.org/articles/10.3389/fphar.2019.00238/pdf
Citations & impact
Impact metrics
Article citations
Molecular interaction analysis of ferulic acid (4-hydroxy-3-methoxycinnamic acid) as main bioactive compound from palm oil waste against MCF-7 receptors: An in silico study.
Narra J, 4(2):e775, 14 Aug 2024
Cited by: 0 articles | PMID: 39280296 | PMCID: PMC11391962
Physiologically based modeling reveals different risk of respiratory depression after fentanyl overdose between adults and children.
Clin Transl Sci, 17(4):e13780, 01 Apr 2024
Cited by: 2 articles | PMID: 38618722 | PMCID: PMC11017203
Fentanyl and its derivatives: Pain-killers or man-killers?
Heliyon, 10(8):e28795, 28 Mar 2024
Cited by: 3 articles | PMID: 38644874 | PMCID: PMC11031787
Review Free full text in Europe PMC
Comparative analysis of the metabolites and biotransformation pathways of fentanyl in the liver and brain of zebrafish.
Front Pharmacol, 14:1325932, 18 Dec 2023
Cited by: 0 articles | PMID: 38174219 | PMCID: PMC10764029
Are carfentanil and acrylfentanyl naloxone resistant?
Front Psychiatry, 15:1359851, 20 Feb 2024
Cited by: 0 articles | PMID: 38445085
Go to all (38) article citations
Other citations
Similar Articles
To arrive at the top five similar articles we use a word-weighted algorithm to compare words from the Title and Abstract of each citation.
In Vitro and In Vivo Metabolite Identification Studies for the New Synthetic Opioids Acetylfentanyl, Acrylfentanyl, Furanylfentanyl, and 4-Fluoro-Isobutyrylfentanyl.
AAPS J, 19(4):1102-1122, 05 Apr 2017
Cited by: 34 articles | PMID: 28382544
A 2017-2019 Update on Acute Intoxications and Fatalities from Illicit Fentanyl and Analogs.
J Anal Toxicol, 45(6):537-554, 01 Jul 2021
Cited by: 18 articles | PMID: 32860688
Review
Acute Intoxications and Fatalities From Illicit Fentanyl and Analogues: An Update.
Ther Drug Monit, 40(1):38-51, 01 Feb 2018
Cited by: 48 articles | PMID: 29120973
Review