Abstract
Free full text

The ribosome cooperates with a chaperone to guide multi-domain protein folding
SUMMARY.
Multi-domain proteins, containing several structural units within a single polypeptide, constitute a large fraction of all proteomes. Co-translational folding is assumed to simplify the conformational search problem for large proteins, but the events leading to correctly folded, functional structures remain poorly characterized. Similarly, how the ribosome and molecular chaperones promote efficient folding remains obscure. Using optical tweezers, we have dissected early folding events of nascent elongation factor G, a multi-domain protein that requires chaperones for folding. The ribosome and the chaperone trigger factor reduce inter-domain misfolding, permitting folding of the N-terminal G-domain. Successful completion of this step is a crucial prerequisite for folding of the next domain. Unexpectedly, co-translational folding does not proceed unidirectionally: emerging unfolded polypeptide can denature an already folded domain. Trigger factor, but not the ribosome, protects against denaturation. The chaperone thus serves a previously unappreciated function, helping multi-domain proteins overcome inherent challenges during co-translational folding.
eTOC Blurb
Liu et al. show that domain-wise folding of nascent proteins can be reversed by denaturing interactions with emerging polypeptide. The chaperone trigger factor blocks denaturation and, together with the ribosome, reduces misfolding. The chaperone thus serves a dual function in promoting efficient folding of multi-domain proteins.
INTRODUCTION.
Combining several functional domains into a single polypeptide is a widespread evolutionary strategy for generating proteins with novel functions (Han et al., 2007). How these complex proteins fold into the specific three-dimensional structures that are required for function remains poorly understood. Beginning with the pioneering experiments by Anfinsen (Anfinsen, 1973), protein folding mechanisms have chiefly been studied using small proteins that fold rapidly and reversibly (Braselmann et al., 2013). Even though many of the mechanistic details remain elusive, several general principles have emerged from these studies (Dill and MacCallum, 2012; Sosnick and Barrick, 2011). For instance, folding transitions are largely cooperative, but “molten globule” intermediates, containing secondary structure while lacking stable tertiary interactions are frequently populated along folding pathways. Comparatively little is known about the folding mechanisms of multi-domain proteins (Jahn et al., 2016; Walters et al., 2013). The population of multiple intermediate states (Brockwell and Radford, 2007) and slow overall folding result in a high propensity of multi-domain proteins to form insoluble aggregates in vitro, hampering mechanistic folding studies.
Folding of multi-domain proteins begins co-translationally (Frydman et al., 1999; Nicola et al., 1999), while the ribosome still elongates the nascent polypeptide chain. As a consequence, these proteins acquire stable structure during synthesis, and co-translational folding has long been recognized as a potential mechanism of facilitating their conformational search (Fedorov and Baldwin, 1997). However, it remains unclear to what degree sequential domain-wise folding is necessary for productive folding of natural multi-domain proteins (Han et al., 2007), whether a particular folding order is required (Batey et al., 2008), and how well biophysical studies of small single-domain proteins reflect folding events in larger multi-domain proteins (Braselmann et al., 2013). In addition, it has recently become apparent that interactions with the ribosome decrease the folding rate (Kaiser et al., 2011) and the stability (Cabrita et al., 2016; Holtkamp et al., 2015; Samelson et al., 2016) of co-translationally formed structures. Whether and how these interactions contribute to productive folding remains unknown.
Molecular chaperones are crucial for cellular protein folding and maintenance, preventing aggregation and guiding the conformational search (Kim et al., 2013). Specialized nascent chain-binding chaperones interact with polypeptides as soon as they emerge from the ribosome during synthesis (Kramer et al., 2009). In bacteria, the chaperone trigger factor (TF) binds to the ribosome near the polypeptide exit tunnel (Kramer et al., 2002). As such, TF is the first chaperone to interact with emerging nascent proteins and, together with the bacterial Hsp70 system, helps the folding of newly synthesized proteins (Genevaux et al., 2004). TF has been reported to increase folding yield by reducing folding rates (Agashe et al., 2004; Hoffmann et al., 2012). However, a mechanistic understanding of TF action is lacking. More generally, the importance of chaperones for the biogenesis of functional proteins is firmly established (Kim et al., 2013), but how they contribute to productive folding remains largely unclear.
Experimentally studying early folding of nascent multi-domain proteins is challenging (Kaiser and Liu, 2018). Many of the optical methods that are traditionally used to follow protein folding, such as circular dichroism spectroscopy or tryptophan fluorescence measurements, cannot be applied in the presence of the ribosome and molecular chaperones. Moreover, transiently populated and potentially heterogeneous states along the folding pathway often remain unresolved in ensemble measurements. Mechanical manipulation of individual protein molecules with optical tweezers is a powerful approach for following folding directly (Zoldak and Rief, 2013), yielding folding rates and one-dimensional structural information. This single-molecule technique is ideally suited for studies of complex proteins (Jahn et al., 2016; Liu et al., 2017; Stigler et al., 2011; Yu et al., 2012) and circumvents the complication of protein aggregation.
We have used optical tweezers to explore early folding steps during the synthesis of elongation factor G (EF-G), a GTPase that is required for efficient polypeptide elongation. EF-G is composed of five domains (AEvarsson et al., 1994; Czworkowski et al., 1994) and has orthologs in all kingdoms of life. We have previously reported (Liu et al., 2017) that interactions among unfolded domains globally interfere with productive folding of EF-G. Here, we show that folding of the N-terminal G-domain of EF-G has to precede folding of the following domain II, imposing a hierarchical folding order. Depending on nascent chain length, the ribosome either accelerates or decelerates G-domain folding. Misfolding between the two domains slows productive folding, but is ameliorated by the ribosome and the chaperone TF. Surprisingly, we find that unfolded parts of the nascent protein can denature natively folded structures, resulting in an unanticipated complication of folding. TF, but not the ribosome, prevents this denaturation. Our studies show how the ribosome and the chaperone together guide early folding to set the protein on the right path for productive folding.
RESULTS.
The ribosome modulates G-domain folding
Domain-wise folding during protein synthesis represents a conceptually straightforward way of simplifying the conformational search for the native state. We generated stalled ribosome-nascent chain complexes (RNCs) of EF-G to study early co-translational folding with optical tweezers (Fig. 1A, and STAR Methods: Methods details). When translation is stalled at codon 328 of the EF-G coding sequence (328RNC), the N-terminal G-domain (aa 1-293 of EF-G) has fully emerged from the ribosome, whereas the following 35 residues (aa 294-328) of domain II span the exit tunnel in the large ribosomal subunit (fig. S1). While this approach does not capture the coupling of polypeptide elongation and folding (Thommen et al., 2017), it enables the dissection of folding processes at defined stages of synthesis. 328RNC thus represents a “snapshot” of EF-G synthesis where the first domain has emerged from the ribosome and can fully fold.
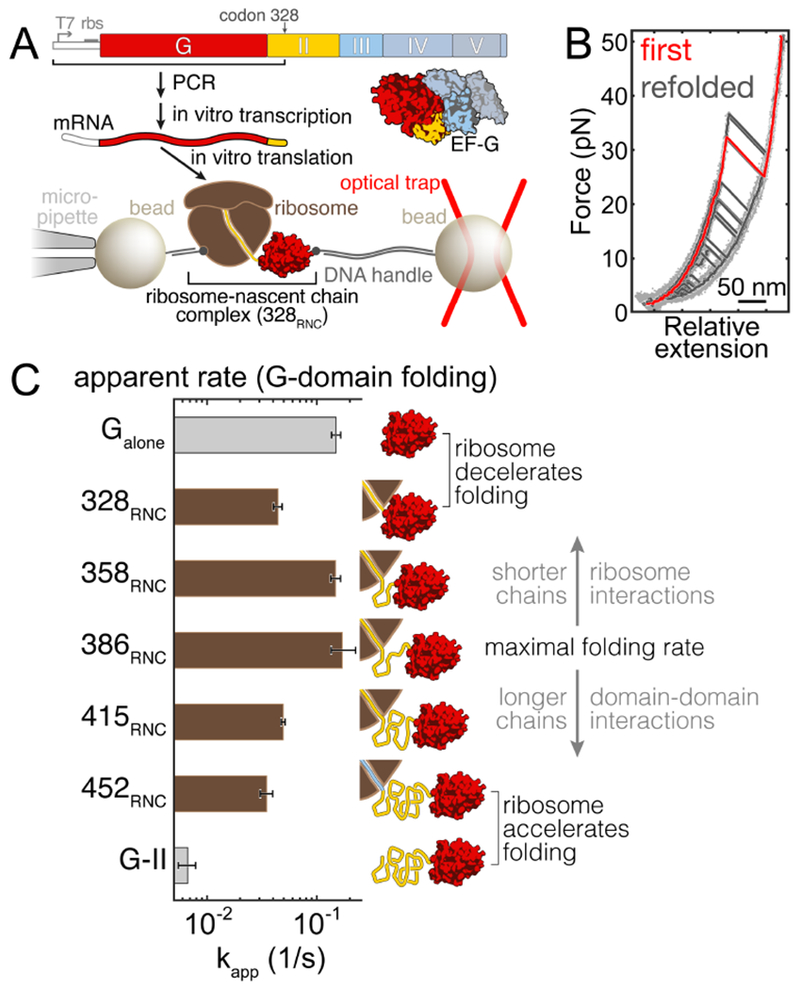
A. Experimental scheme. The bar diagram on top illustrates the EF-G domain topology. For optical tweezers experiments, stalled ribosome-nascent chain complexes (RNCs), generated in vitro using stop codon-less mRNAs, are tethered between two polystyrene beads. B. Example force-extension curves (FECs) for 328RNC. The native G-domain unfolds in the initial FEC (red trace). In subsequent pulls (black), separated by 10 second refolding pauses, unfolding transitions from the fully structured state are apparent in some of the traces. In addition, partially structured and molten globule-like states are observed. C. Apparent folding rates of the G-domain for the isolated Galone polypeptide (top, grey bar) and RNCs with varying lengths (brown bars). The cartoons on the right indicate the stalling positions for the respective constructs. The ribosome decelerates folding at short nascent chain lengths (328RNC vs. Galone). After reaching a maximum, folding rates drop in longer nascent chains due to non-productive interactions within the nascent polypeptide. This interference is even more pronounced in the isolated G-II protein comprised of the G-domain and domain II (bottom, grey bar), indicating that the ribosome reduces non-productive inter-domain interactions.
We applied a continuously increasing force (“force ramp”) to individual 328RNC molecules (see STAR Methods: Methods details). The resulting force extension curves (FECs) showed transitions that indicated cooperative unfolding of the natively structured G-domain (Fig. 1B, red trace). Measuring the unfolding force and extension change (fig. S2) of the initial unfolding transitions, we obtained a contour length change of ΔL = 103.2 nm ± 1.8 nm (mean ± standard deviation, std; 30 molecules). This value is very close to the expected contour length change of ΔLcalc = 103.0 nm (see STAR Methods: Quantification and statistical analysis), calculated from the E. coli EF-G structure (Pulk and Cate, 2013), indicating that the G-domain adopts its native structure in 328RNC (Liu et al., 2017) and suggesting that its folding is an important waypoint toward native EF-G.
After complete unfolding, we relaxed the force to 2 pN for 10 seconds to permit refolding. Subsequently, we pulled again to probe which structures had formed. Repeated cycles of pulling and relaxation yielded heterogeneous transitions (Fig. 1B, black traces, and fig. S2). The G-domain refolded in 35.8% of attempts, as indicated by unfolding transitions similar to the initial one (fig. S2). In addition, mechanically weak molten-globule-like structures (Cecconi et al., 2005) and partially folded intermediates are observed frequently in 328RNC (Fig. 1B, fig. S2E). These states are detected much more rarely in an isolated polypeptide comprising the G-domain (termed Galone here) (fig. S2F). Instead, Galone exhibits a higher fraction of fully folded structures, suggesting that folding proceeds more rapidly in the isolated protein than on the ribosome.
The population of several partially structured states (fig. S2E) suggests an intricate folding mechanism for the G-domain that is challenging to fully characterize. We quantified overall folding rates by analyzing the formation of fully structured states during repeated force ramp cycles (fig. S3, and STAR Methods: Quantification and statistical analysis). By fitting the cumulative folding probability to a geometric distribution (fig. S3D), we determined an apparent folding rate of kapp(328RNC) = 0.044 s−1 (95% confidence intervals, ci: 0.040 s−1, 0.048 s−1) for 328RNC, slower than that of the free protein (kapp(Galone) = 0.149 s−1 (ci: 0.136 s−1, 0.165 s−1)) (Fig. 1C, fig. S3E). Thus, the overall G-domain folding rate is reduced in close proximity to the ribosome, presumably due to interactions of the ribosome with the nascent polypeptide. Such a deceleration of folding is consistent with previous reports (Kaiser et al., 2011; Liu et al., 2017).
Shifting the stalling position from codon 328 to codon 358, and further to codon 386 in the EF-G coding sequence (358RNC and 386RNC, respectively; fig. S1) gradually restored the G-domain folding rate [kapp(358RNC) = 0.148 s−1 (ci: 0.134 s−1, 0.164 s−1; kapp(386RNC) = 0.170 s−1 (ci: 0.135 s−1, 0.225 s−1)] (Fig. 1C, fig. S3E). The polypeptide exit tunnel that traverses the large ribosomal subunit is approximately 100 Å long, accommodating about 35 amino acids of unfolded polypeptide. Therefore, approximately 60 aa of domain II have fully emerged from ribosome in 386RNC, and the effect of the ribosome on G-domain folding appears to be minimal. These findings suggest that the distance-dependent modulation of folding kinetics observed previously with artificial T4 lysozyme nascent chains (Kaiser et al., 2011) is relevant during the folding of an authentic multi-domain protein. During EF-G synthesis, folding of the N-terminal G-domain is hence expected to occur with high probability soon, although not immediately, after it has emerged from the ribosome. Given the lower and upper boundaries for the folding rate of the nascent G-domain from 328RNC and 386RNC, G-domain folding should be complete with very high probability before domain II is fully synthesized.
Interference among domains slows down folding
Elongating the nascent chain further to include 415 and 452 residues of EF-G (415RNC and 452RNC; fig. S1) resulted in progressively decreased folding rates [kapp(415RNC) = 0.049 s−1 (ci: 0.047 s−1, 0.051 s−1); kapp(452RNC) = 0.035 s−1 (ci: 0.031 s−1, 0.040 s−1)] (Fig. 1C, fig. S3E). Since the ribosome does not modulate folding over long distances, interactions within the nascent polypeptide must be responsible for the observed effect. Most likely, the unfolded domains form off-pathway structures that slow down overall folding. Frustration of G-domain folding was even more pronounced in an isolated polypeptide containing the first two EF-G domains (aa 1-410 of EF-G, termed G-II here). Compared to 452RNC, the rate of G-domain folding in G-II is much reduced (kapp(GG-II) = 0.006 s−1 (ci: 0.004 s−1, 0.007 s−1), Fig. 1C, fig. S3E).
Our findings imply that the G-domain is likely to fold within a narrow window during EF-G synthesis. The folding rate reaches a maximum when ~100 residues of domain II have been synthesized (386RNC). Folding is slowed down by the ribosome in shorter nascent chains (358RNC), and by interactions within the nascent polypeptide in longer ones (415RNC, 452RNC) Previously, the ribosome was thought to exclusively delay folding (Cabrita et al., 2016; Kaiser et al., 2011). Here, it appears that the effect of the ribosome on the folding of a particular domain is context specific: when close to the ribosome, G-domain folding is slowed (328RNC vs. Galone). In longer nascent chains, the ribosome accelerates G-domain folding, relative to an analogous isolated polypeptide (452RNC vs. G-II). Therefore, presumably similar interactions between the ribosome and the nascent polypeptide result in distinct outcomes, depending on the stage of synthesis.
The ribosome and TF reduce inter-domain misfolding
To characterize the structures that the two-domain EF-G constructs populate during refolding in the presence (452RNC) and absence (G-II) of the ribosome, we carried out “force clamp” experiments. We subjected these molecules alternatingly to high force (30 pN), favoring unfolding, and low force (3.5 pN), permitting refolding. When held at this low force, the isolated G-II polypeptide transitions between several states (Fig. 2A). Hopping between intermediate states ceases when the G-domain adopts its folded structure (“GN-IIU”, Fig. 2A). Refolding of domain II is not observed under these conditions (3.5 pN, time cutoff: 120 seconds).
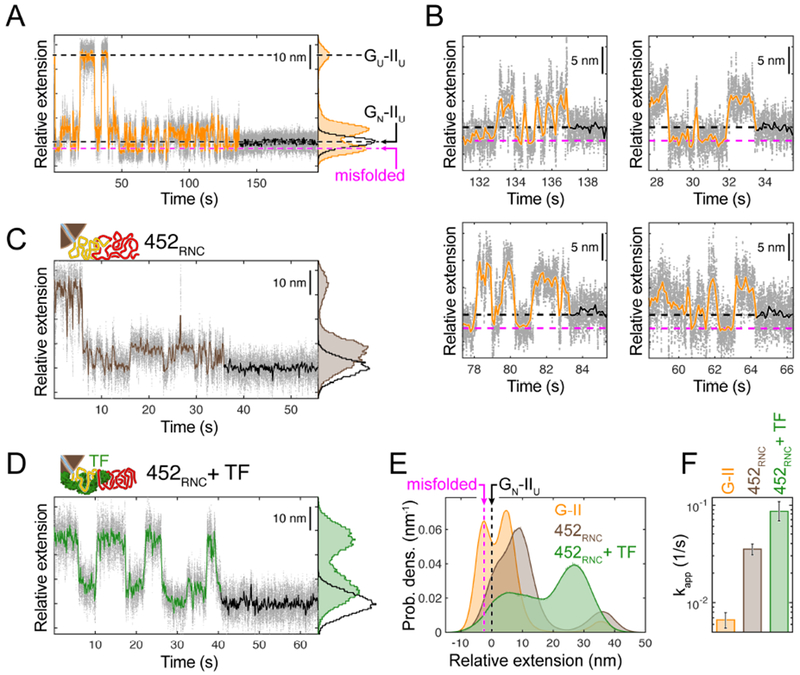
A. Example of G-domain refolding against a constant force of 3.5 pN for G-II. Raw data (1 kHz) is shown as grey dots, time-averaged data (10 Hz) as a line (orange before G-domain folding, black thereafter). Black dashed lines indicate states before and after G-domain folding (GU-IIU, GN-IIU). G-II also populates a misfolded state with a shorter extension (magenta dashed line). Histogram (right): extensions populated before (orange) and after (black) G-domain folding. B. Close-up view of the refolding transitions for four G-II example traces. In all cases, the misfolded state partially unfolds before productive folding occurs (black and magenta dashed lines as in A). C. Constant force refolding of 452RNC. The population of the compact misfolded species is reduced, as apparent from the extension-vs.-time trace and the extension histogram. D. Refolding in the presence of TF. The chaperone shifts the population away from the misfolded state. E. Aggregated extension histograms for the equilibrium portion of constant force refolding traces, aligned to the GN-IIU state. The misfolded state is highly populated in G-II (orange), but less so in 452RNC without (brown) and with (green) TF. F. Apparent G-domain refolding rate for G-II, 452RNC (as in Fig. 1C) and 452RNC + TF. Reduced misfolding in the presence of the ribosome and the chaperone results in faster folding.
Notably, the extension of one G-II folding intermediate (magenta arrow, Fig. 2A) is shorter than that of GN-IIU. This intermediate must therefore contain parts of both domains (fig. S4A and B, and STAR Methods: Quantification and statistical analysis). Consistent with this interpretation, the state is not observed with the Galone construct (fig. S4C). Because the short intermediate does not transition directly to the GN-IIU state (Fig. 2B), it most likely represents a misfolded, off-pathway species. Our force clamp measurements thus directly reveal inter-domain misfolding in G-II that interferes with G-domain folding.
Remarkably, the population of the short misfolded state, which is prominent in G-II, is much reduced in the nascent polypeptide (Fig. 2C and E). It therefore appears that the ribosome reduces the propensity of domain II to form misfolded states with the G-domain. As a result, the rate of G-domain folding is higher in 452RNC compared to G-II (Fig. 2F), by either reducing the rate of entering in the misfolded state or by increasing the rate of leaving the misfolded state, or a combination of both. The folding rate is not restored to its intrinsic value, suggesting that the nascent polypeptide still populates off-pathway species, but to a lesser degree. Hence, the ribosome appears to alleviate some of the frustration that arises when both domains navigate the folding landscape simultaneously. As such, ribosome-nascent chain interactions may constitute a first line of defense against inter-domain misfolding.
We next assessed how the ribosome-binding chaperone TF might influence folding and misfolding of nascent EF-G. TF binds to a specific site on the ribosome near the polypeptide exit tunnel with moderate affinity (Kramer et al., 2002), and more strongly to RNCs (Kaiser et al., 2006). Significantly, EF-G has been demonstrated to be a bona fide TF substrate (Deuerling et al., 2003). Measurements with 452RNC in the presence of TF revealed a pronounced shift in the extensions of the intermediates populated during G-II folding (Fig. 2D and E). The chaperone causes an overall compaction of the unfolded protein and shifts the intermediate extensions away from the misfolded state more strongly than the ribosome (Fig. 2E). This remodeling of the folding landscape results in a G-domain folding rate of 0.087 s−1 (ci: 0.068 s−1, 0.109 s−1) (Fig. 2F), higher than that observed in the absence of the chaperone and closer to the intrinsic rate of G-domain folding in isolation. TF therefore appears to sequester the unfolded domain II, preventing it from inducing misfolding and thereby neutralizing its effect on G-domain folding rate. Our measurements thus show how the chaperone promotes folding of an authentic client protein.
Contacts with the G-domain stabilize domain II
In 452RNC, the nascent chain is sufficiently long for domain II to emerge from the ribosome (fig. S1) and fold. Indeed, the initial pull on 452RNC reveals sequential unfolding transitions of the G-domain and domain II (fig. S5B). A very similar unfolding pattern is observed for G-II (Fig. 3A). In both cases, each domain unfolds with a characteristic pattern of either one single step or two steps (fig. S5C and D). This apparent heterogeneity is explained by stochastic lifetimes of the unfolding intermediate that are short on average. Consequently, the intermediate is resolved in only a fraction of events at our temporal resolution of 1 ms. Very similar transitions are observed during the initial unfolding of full-length EF-G (Liu et al., 2017) (fig. S5E), indicating that the two domains adopt the same structure in G-II and 452RNC as in the full-length protein. After complete unfolding, the G-domain refolds sporadically after the polypeptide is relaxed to 2 pN (Fig. 3A, fig. S5). In contrast, refolding of domain II does not occur under these conditions (Fig. 3A, fig. S5).
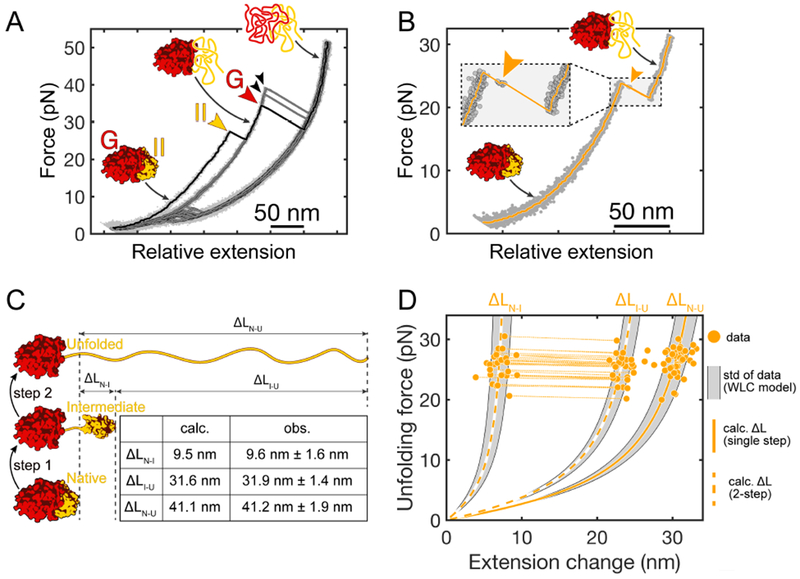
A. Example FECs for G-II. Clear signatures of both domains (colored arrowheads) are apparent in the initial trace (black). The G-domain refolds in some of the attempts (black arrowheads), but refolding of domain II is not observed subsequent traces (grey). B. Transient population of an unfolding intermediate (arrowhead) during II unfolding. Inset: magnified view of the unfolding transition. The short lifetime of the intermediate indicates that it is unstable. C. Schematic illustrating the likely pathway for two-step unfolding of domain II in G-II. The interface between the G-domain and domain II breaks first, followed by unfolding of the remaining domain II structure. The table lists the contour length changes expected based on the EF-G structure (“calc.”) and the observed values (“obs”) for the transitions. D. Scatter plot of domain II unfolding transitions in G-II. Each dot represents an unfolding transition. Transitions from two-step unfolding via the intermediate are connected by dotted lines. Dashed and solid lines represent WLC models calculated for two-step (ΔLN-I, ΔLI-U) and one-step (ΔLN-U) unfolding (see panel C). The shaded areas indicate the respective standard deviations of the observed contour length changes.
In the folded state, the G-domain and domain II form an extensive interface with a buried surface area of almost 2000 Å2 (Pulk and Cate, 2013). These contacts conceivably contribute to the structural stability of domain II. We speculated that this interface may break when tension is applied during force ramp experiments. In such a scenario, the two domains would reorient under the applied tension, and a largely unstructured region connecting them (aa 286 – 317) would be stretched, resulting in a calculated contour length change of ΔLN-I(calc.) = 9.5 nm (Fig. 3C). Subsequently, unfolding of the remaining domain II structure would yield a further increase of ΔLI-U(calc.) = 31.6 nm. The experimentally observed length changes (ΔLN-I(obs.) = 9.6 nm ± 1.6 nm, ΔLI-U(obs.) = 31.9 nm ± 1.4 nm; mean ± standard deviation; Fig. 3D) are very similar to these values, suggesting that domain II unfolds through this pathway in our experiments. Rapid unfolding after interface rupture indicates that domain II is unstable by itself, whereas the G-domain remains folded until the force is further increased (Fig. 3A, fig. S5). Contacts with the folded G-domain therefore appear to be important for the stability of domain II.
Domain II folding requires the folded G-domain
We hypothesized that the dependence of domain II stability on the interface with the folded G-domain might impose a sequential order on domain folding during EF-G synthesis: domain II can stably fold only after the G-domain has acquired its native structure. We used the G-II construct to test whether folding of domain II (IIG-II) indeed requires the folded G-domain (GG-II), exploiting the differential mechanical stability of the two domains (fig. S5F). Domain II always unfolds before the G-domain in force ramp experiments, providing an opportunity to assess the importance of native interface contacts for folding.
We selectively unfolded IIG-II in force ramp experiments, keeping GG-II folded, before lowering the force to initiate refolding (Fig. 4A). Domain II refolds under these conditions, typically after several force ramp cycles (Fig. 4A). Unfolding transitions after refolding are indistinguishable from the initial ones (Fig. 4B, fig. S6A to C), indicating that IIG-II regained its native structure under these conditions. Contacts with the natively folded G-domain therefore appear to be required for stable structuring of domain II. Consistent with this notion, a polypeptide containing only domain II did not accumulate upon expression of a recombinant construct in E. coli (data now shown), presumably because the translation product is not stably structured and quickly degraded by cellular proteases. Our results imply a requirement for ordered domain-wise folding: IIG-II folds only after GG-II has folded (Fig. 4C).
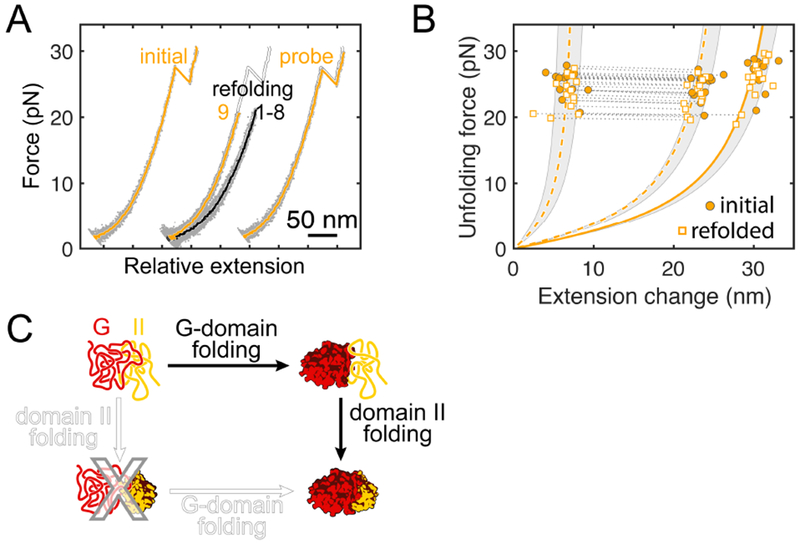
A. Example FECs showing slow folding of domain II. Domain II is selectively unfolded while leaving the G-domain structured (yellow trace, “initial”). Refolding is apparent in trace “9” (yellow) after several futile attempts (black traces, “1-8”). Subsequent forced unfolding confirms domain II refolding (yellow trace, “probe”). Traces are plotted with an offset along the x axis for clarity, the “initial” trace is re-plotted in white as a reference. B. Scatter plot for unfolding of renatured domain II, confirming folding to the native structure in the presence of the folded G-domain. Dashed and solid yellow lines represent WLC models for two-step and one-step unfolding, shaded regions indicate the standard deviation of the experimental data. C. Domain II does not stably fold by itself because it requires stabilizing contacts at the interface with the G-domain. Consequently, the G-domain has to fold first, imposing a hierarchical folding order.
Unfolded domain II destabilizes the native G-domain
Strikingly, refolding of domain II was not the only outcome we observed after selectively unfolding IIG-II. In some refolding attempts, GG-II unfolded at uncharacteristically low forces, often in the range between 5 and 10 pN (Fig. 5A and B). In contrast, unfolding of native GG-II invariably occurred between ~30 and 50 pN (Fig. 5B), similar to Galone and all nascent chains. The low force transitions are well outside this range (Fig. 5B), indicating that GG-II had assumed a non-native conformation before we probe its structure mechanically. The transitions resemble partially folded and molten globule-like states populated by 328RNC (Fig. 5C) and G-II (fig. S6D) before G-domain refolding, suggesting that GG-II progressively loses its native structure and subsequently enters into misfolding interactions with domain II. Low-force unfolding is not observed with Galone (0 events in 399 trials, p-value: 1.1×10−27, see STAR Methods: Quantification and Statistical Analysis), confirming that interactions with the unstructured domain II pull the G-domain out of its native state. It thus appears that unfolded IIG-II “denatures” GG-II, leading to a complete loss of nativeness (Fig. 5E).

A. Example of G-domain denaturation. After selectively unfolding domain II (yellow, “initial”), unfolding of the G-domain at low force during the second cycle (magenta, “2”) indicates denaturation. The initial trace is re-plotted in white as a reference. B. Distribution of unfolding forces. The native G-domain (red) unfolds in a force range that is well separated from that after denaturation (magenta). C. Scatter plot showing individual unfolding events after G-domain denaturation (magenta). The distribution resembles that of molten globule-like and partially structured states observed after mechanical unfolding of 328RNC (grey dots, data from fig. S2E), indicating that denaturation ultimately results in complete unfolding. D. Cumulative probability of domain II refolding (yellow) and G-domain denaturation (magenta) during repeated force ramp cycles. Both events occur with similar probability and thus on similar timescales. E. In addition to folding productively, domain II can cause G-domain denaturation, which then results in complete unfolding of the polypeptide. The two processes compete with each other.
During EF-G synthesis, the G-domain is likely to fold while domain II is being translated, as described above (Fig. 1). Our experiments indicate that the polypeptide can then proceed along one of two routes, leading to either folding of domain II (Fig. 4) after its complete synthesis, or to denaturation of the G-domain (Fig. 5) and subsequent misfolding. To determine which outcome is more likely, we compared the cumulative probabilities of the two processes. G-domain denaturation and domain II folding occur on similar timescales in the G-II construct (Fig. 5D). This observation suggests that folding and denaturation compete with each other in the isolated G-II polypeptide (Fig. 5E).
TF protects against denaturation
To determine whether the ribosome protects the folded G-domain against denaturation by unfolded domain II, we repeated the experiment with the 452RNC construct. In contrast to the frustration resulting from interactions among unfolded domains, denaturation of natively folded GG-II is not alleviated by the ribosome (Fig. 6A). Consequently, even after the G-domain in nascent EF-G has folded, it is still at risk of losing its native structure as long as domain II is unfolded. Subsequently, the two non-native domains likely enter into misfolding, as observed after complete unfolding of G-II (Fig. 2). Denaturation of the G-domain therefore competes with folding of domain II (Fig. 6B) and thus has the potential to derail productive co-translational folding.

A. Cumulative probability distributions of G-domain denaturation. The ribosome does not protect against denaturation (G-II vs. 452RNC), which already occurs after partial synthesis of domain II (386RNC). TF blocks denaturation (452RNC + TF). B. Before domain II has been fully synthesized (386RNC), it cannot fold, but already destabilizes the neighboring folded G-domain. After domain II has emerged from the ribosome (452RNC), competing pathways result in either G-domain denaturation or folding of domain II. TF blocks denaturation, favoring folding.
Because folding of protein domains is cooperative, domain II can adopt its native structure only after it has fully emerged from the ribosome. Consistent with this notion, we did not observe any stable structures attributable to domain II in experiments with 415RNC (fig. S5) or shorter nascent chains. Denaturation of the G-domain, on the other hand, is already observed when only 386 codons have been translated (386RNC, Fig. 6A). In 386RNC, only about half of domain II has exited the ribosome exit tunnel, but destabilization of the folded G-domain structure is already observed (Fig. 6A), and constructs containing truncated domain II aggregated upon expression in E. coli (data not shown). These results suggest that denaturation can begin before productive folding is possible. In the context of elongating nascent EF-G, the balance between productive folding and denaturation is therefore skewed toward the non-productive pathway (Fig. 6B).
Denaturation of the G-domain by unfolded, elongating domain II represents an unanticipated complication of domain-wise co-translational folding. How is the nascent polypeptide kept on a productive folding track in the cell? As shown above, the ribosome does not protect against denaturation. In contrast, TF completely prevented denaturation of the G-domain (Fig. 6A). Given a base probability of denaturation of p = 0.144 per cycle (see Supplementary Information), the probability that we missed similar denaturation events in the presence of TF is very low (0 events in 167 trials, p-value: 5.3×10−12, see STAR Methods: Quantification and statistical analysis). Our experiments thus reveal a dual role of TF during early EF-G folding: The chaperone not only helps to resolve the frustration resulting from inter-domain misfolding (Fig. 2), but also protects the folded G-domain against denaturation by the unfolded domain II (Fig. 6).
DISCUSSION.
We have defined early events during the folding of a nascent multi-domain protein, EF-G (summarized in Fig. 7). Interactions of the nascent polypeptide with the ribosome are beneficial for folding soon, but not immediately after the domain has been synthesized (Fig. 1). Experiments with full-length EF-G (Liu et al., 2017) and other multi-domain proteins (Jahn et al., 2016; Mashaghi et al., 2014; Scholl et al., 2017) have previously suggested misfolding among domains. Here, we directly observe misfolding among EF-G domains (Fig. 2), and further demonstrate that a natively structured N-terminal G-domain is a prerequisite for stable folding of the subsequently synthesized domain II (Fig. 3, Fig. 4). In the case of EF-G, domain folding is therefore hierarchical and occurs in the order in which domains are synthesized, promoting productive co-translational folding. Failure of the G-domain to fold soon after domain II begins to emerge likely has deleterious consequences for overall EF-G folding. As more and more unfolded polypeptide accumulates during synthesis, misfolding makes completion of the required early folding steps increasingly difficult, resulting in global misfolding and irreversible aggregation. Folding of the N-terminal domain therefore constitutes an important waypoint along the folding pathway.
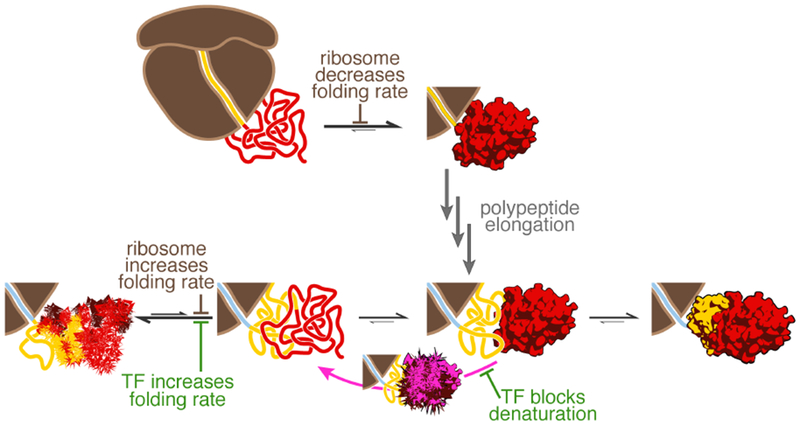
After the G-domain has emerged from the ribosome (top), its folding rate is reduced by interactions with the ribosome. Presumably similar interactions, now with domain II, reduce inter-domain misfolding in longer nascent chains (bottom), effectively increasing the G-domain folding rate. How the ribosome affects a particular domain thus depends on nascent chain length. The reduction in inter-domain misfolding is reinforced by TF. G-domain folding has to precede folding of domain II. Domain II folding competes with denaturation of the already folded G-domain. The latter process is prevented by TF, but not the ribosome. Hence, TF has a dual function in promoting early folding events in nascent EF-G.
Previous studies with small proteins artificially tethered to the ribosome (Hoffmann et al., 2012; Kaiser et al., 2011; Samelson et al., 2016), have suggested that the ribosome helps to prevent premature folding by destabilizing folded structures and reducing folding rates. Here, a natural multi-domain protein also shows this effect (Fig. 1). However, we observe an additional consequence of ribosome-nascent chain contacts that is potentially more important for multi-domain proteins (Fig. 2): sequestering the proximal part of the nascent chain (domain II in 452RNC) reduces the formation of misfolded states with more distal parts (the G-domain in 452RNC). The effect of the ribosome on overall folding is therefore highly dependent on nascent chain length and can manifest as either decelerated (328RNC vs. Galone) or accelerated (452RNC vs. G-II) folding (Fig. 1). Our findings provide a framework for studying the bioinformatically (Chaney et al., 2017; Pechmann and Frydman, 2013) and experimentally (Buhr et al., 2016; Mercier and Rodnina, 2018; Zhang et al., 2009) observed tuning of synthesis and folding rates.
TF has been proposed to act as a “holdase” for nascent proteins, preventing and even reversing premature folding (Hoffmann et al., 2012). Here, we show that TF prevents inter-domain misfolding within nascent EF-G chains, amplifying a similar contribution from the ribosome (Fig. 2). As a result, the chaperone can effectively speed up folding (Fig. 2F), in contrast to the previously observed global deceleration (Agashe et al., 2004). Interestingly, our data suggest that the states along the G-domain folding pathway change in the presence of TF, as indicated by the population of intermediate states with altered molecular extensions (Fig. 2D and E). A previous study, using the periplasmic maltose binding protein (Mashaghi et al., 2013), suggested that TF stabilizes partially folded structures. A simpler explanation, consonant with a recent structural characterization of TF-substrate interactions (Saio et al., 2014), could be that the chaperone, by simultaneously binding to several sites, reduces the extension of the protein while keeping it largely unfolded. The changes in state extensions that we observe in our constant force refolding measurements are consistent with this interpretation (see STAR Methods: Quantification and statistical analysis). A consequence of forming multiple contacts that are spaced far apart in the client protein primary structure is a reduction in chain entropy, which may facilitate subsequent folding (Haldar et al., 2017).
Unexpectedly, our measurements revealed that an unfolded part of the nascent polypeptide can denature an already folded domain (Fig. 5). The folded G-domain might transiently visit a partially unfolded state that is then captured by unfolded domain II. Given that such an unfolding intermediate is not detected in our measurements and thus does clearly not result in a large change in molecular extension, it appears unlikely that the force applied during the measurement favors its formation. An alternative explanation could be that domain II, rather than capturing transient intermediates, “invades” the folded G-domain and causes denaturation. Regardless of the underlying mechanism, the interaction ultimately results in loss of native structure (Fig. 5C). While the ribosome does not protect against denaturation, the chaperone TF effectively prevents it (Fig. 6), presumably by sequestering unfolded domain II and preventing it from interacting with the G-domain. Therefore, in addition to preventing the misfolding of unfolded or partially structured states, the chaperone also protects already folded structures (Fig. 7).
While some proteins utilize disordered sequences for function and regulation (Motlagh et al., 2014), others have evolved to remain stably folded. Intertwined domain topologies (Shank et al., 2010) and interactions among topologically separate domains (Flaugh et al., 2005; Han et al., 2007) may serve to minimize the population of partially unfolded states that could potentially initiate more global unfolding. Nevertheless, unfolded segments are necessarily present during protein synthesis by the ribosome for extended periods of time, due to relatively slow elongation rates (~13-22 amino acids per second in bacteria (Bremer and Dennis, 2008)). An unanticipated major role of nascent chain-binding chaperones may thus be to protect folded domains against denaturation by unfolded sequence segments during co-translational folding. Whether chaperones functioning post-translationally to maintain a folded proteome act through similar mechanisms remains to be assessed in future studies.
STAR METHODS
CONTACT FOR REAGENT AND RESOURCE SHARING.
Further information and requests for resources should be directed to and will be fulfilled by the lead contact (Christian Kaiser, ude.uhj@resiak).
METHODS DETAILS.
Expression and purification of EF-G constructs
An overview of the isolated proteins used in the present study is provided in fig. S1B. Proteins (Galone and G-II) were overexpressed, purified and derivatized similarly to previously described procedures (Kaiser et al., 2011; Liu et al., 2017). We amplified the open reading frames encoding either G (residues 1-294 of EF-G) or G-II (residues 1-410 of EF-G) from E. coli MC4100 genomic DNA using polymerase chain reaction (PCR) and inserted it into the pBAD His6-SUMO TEV LIC cloning vector (Addgene Plasmid #37507), which had been engineered to encode an N-terminal Avi tag (Beckett et al., 1999) and C-terminal ybbR tag (Yin et al., 2006). These two tags are used for tethering proteins for optical tweezers experiments (Kaiser et al., 2011). The His6-SUMO tag served as a cleavable affinity tag to purify the recombinantly produced proteins. Plasmids were transformed into BL21-Gold(DE3) (Agilent Technologies) host cells for recombinant gene expression. Expression was induced with a final concentration of 0.2% (w/v) L-arabinose (AMRESCO) at 25°C when the OD600 reached ~0.5. Cells were harvested after induction for 5 h at 25°C. Cells were lysed in buffer HN (25 mM HEPES-KOH, 100 mM NaCl, pH 7.4) using an EmulsiFlex-C5 (Avestin). After cell lysis and centrifugation at 30,000g, 4°C to remove cell debris, proteins were affinity purified from the supernatant using a 5 ml HisTrap column (GE Healthcare) charged with Ni2+. Proteins were dialyzed against buffer HN overnight, together with 1:1000 (w/w) Ulp1 to cleave the His6-SUMO tag (Mossessova and Lima, 2000). The solution was applied again to the regenerated HisTrap column which retained uncleaved protein, the His6-SUMO moiety and the His6-tagged Ulp1 enzyme. The flow-through was then incubated with BirA biotin ligase (1 μM) in 1X biotinylation buffer (500 μM D-biotin, 5 mM ATP, and 5 mM Mg-acetate) at 25°C for 1 h to ensure complete biotinylation of the Avi-tagged proteins. The products were concentrated and loaded onto a HiPrep 16/60 Sephacryl S-300 HR column (GE Healthcare) and eluted with HKMD buffer (20 mM HEPES-KOH, 100 mM KCl, 5 mM MgCl2, 1 mM 1,4-dithiothreitol, pH 7.4).
Protein modification
To introduce DNA handles for tethering, we incubated the purified ybbR-tagged proteins with CoA-modified double-stranded DNA (dsOligo-CoA) and in-house purified Sfp enzyme (Yin et al., 2006) in HM buffer (50 mM HEPES-KOH, 10 mM MgCl2, pH 7.4) with final concentration of ~50 μM proteins, 50 μM dsOligo-CoA, and 5 μM Sfp. The enzyme couples the CoA-moiety to a specific residue in the ybbR-tag, resulting in position-specific, covalent coupling of the oligonucleotide to the protein. The dsOligo-CoA contained a 4-nucleotide single-stranded overhang at the end distal to the CoA moiety. The reaction products were subjected to a Superdex 200 10/30 GL column (GE Healthcare) in HKMD buffer to separate the protein-DNA handle products from unmodified protein, unreacted oligonucleotide and enzyme. Modified proteins were concentrated, flash-frozen and stored at −80°C.
Immobilization of DNA on polystyrene beads
To functionalize polystyrene microspheres with DNA, we mixed either single-stranded or double-stranded oligonucleotides containing a 5’ amino-modifier (Table s2) with 2.1 μm carboxyl-polystyrene beads (Spherotech). Coupling of the oligonucleotide to the bead surface was achieved by chemical crosslinking with 1-ethyl-3-[3-dimethylaminopropyl] carbodiimide hydrochloride (EDC; ThermoFisher) in the presence of N-hydroxysulfosuccinimide (Sulfo-NHS) in 100 mM MES-Na (pH 6) buffer. The reaction was quenched by addition of TE8 buffer (20 mM Tris-HCl, 1 mM EDTA, pH 8). Unreacted material was removed by washing the beads three times with TE8. Beads modified with single-stranded DNA (“ssDNA-beads”) were used to generate long DNA handles. Beads modified with double-stranded DNA (“dsDNA-beads”) were used to immobilize proteins and RNCs. In this case, the double-stranded oligonucleotides contained a 4-nucleotide single-stranded overhang with a sequence reverse-complementary to the overhang of the dsOligo-CoA DNA.
Preparation of DNA handle beads
To generate bead-immobilized DNA handles, the oligonucleotide on ssDNA-beads was extended by Taq polymerase. Beads were incubated with a linear DNA template that had the same sequence as the desired DNA handle, a 5’-biotinylated oligonucleotide that served as a reverse primer, and PCR mixture (containing Taq polymerase (New England Biolabs), manufacturer-provided buffer, and dNTPs). The reaction was subjected to thermal cycling, resulting in the synthesis of a 1789 bp long dsDNA handle on the bead-immobilized primer with a single biotin at the distal end. Beads were washed to remove unincorporated components and incubated with a large excess of streptavidin (ThermoFisher Scientific). Unbound streptavidin was removed by repeated washing of the beads. At the end of the procedure, “DNA handle-beads” contained covalently immobilized DNA handles (1789 bp long) which were stably bound at the distal end to a streptavidin molecule.
Tethering in optical tweezers experiments
We immobilized proteins or RNCs on polystyrene beads immediately prior to optical tweezers experiments by incubating the DNA-coupled protein with dsDNA-beads in 1× T4 ligase buffer (New England Biolabs) in the presence of T4 ligase. During ligation, the protein/RNC is coupled to the bead through a double-stranded DNA linker. Unreacted material was removed by sedimenting the beads three times and discarding the supernatant. Beads were then injected into channel 1 of the optical tweezers flow cell, and a single bead was immobilized the tip of a micropipette. DNA handle-beads were injected into channel 2 of the flow cell. A single DNA handle-bead was trapped and brought into close proximity of the bead on the micropipette to establish a connection with the biotinylated N-terminus of the protein or nascent chain, forming a tether (Fig. 1A) that was then used in optical tweezers experiments.
Preparation of ribosome-nascent chain complexes (RNCs) for optical tweezers experiments
An overview of nascent chain lengths used in this study is shown in fig. S1C. RNCs were prepared as described previously (Kaiser et al., 2011; Liu et al., 2017). We generated PCR products that share the same 5’ T7 promoter and ribosome binding site, but end at the indicated codon positions (Table s1). The PCR product did not encode a stop codon. PCR products were in vitro transcribed (T7 MegaScript Kit, Ambion), and the mRNA was isolated (MegaClear Kit, Ambion) after digestion of template DNA. Stalled RNCs were generated using a reconstituted in vitro translation system (PURExpress ΔRibosome Kit, New England Biolabs) supplemented with ribosomes (Kaiser et al., 2011). Prior to the translation reaction, ribosomes were reacted with CoA-modified oligonucleotides and isolated by centrifugation. We utilized a >3-fold molar excess of mRNA over ribosomes to favor the formation of monosomes. The translation reaction also contained biotin and the BirA enzyme, resulting in biotinylation of the nascent polypeptide at an N-terminal Avi tag, which provide an attachment point in optical tweezers experiments. Because the mRNAs did not contain a stop codon, the translation product accumulated as a stably stalled peptidyl-tRNA that was sensitive to puromycin treatment, as assessed by Western blotting (data not shown). These RNCs were isolated by centrifugation through a sucrose cushion, dissolved in buffer HKMβ, flash-frozen and stored at −80°C. Immediately prior to optical tweezers experiments, RNCs were coupled to dsDNA-beads and tethered as described above.
Expression and purification of TF
TF was overexpressed with a TEV protease cleavable N-terminal His6-tag from a pPROEX-HTa based plasmid (Kaiser et al., 2006) in BL21-Gold(DE3) (Agilent Technologies) cells at 37°C for 3 hours. The cells were lysed at in buffer PBSI20 (137 mM NaCl, 2.68 mM KCl, 10.1 mM Na2HPO4, 1.76 mM Na2HPO4, 20 mM Imidazole, pH 7.4) by three passages through an EmulsiFlex-C5 (Avestin) homogenizer at 15,000 psi. The supernatant after centrifugation at 30,000 g, 4°C for 30 minutes was recovered and applied to a HisTrap column (GE Healthcare) charged with Ni2+. The eluate was dialyzed against PBS buffer with 2 mM DTT together with His6-tagged TEV protease (2 mg/ml, purified in-house) at a ratio of 100:1 (v/v) overnight at 4°C. After digestion, the sample was passed over a HisTrap column again. His6-tagged TEV protease, uncleaved His6-tagged TF and the cleaved His6-peptide were retained on the column. The flow through, containing TF without the His6-tag, was collected, flash-frozen and stored at −80°C.
Optical tweezers force ramp experiments
Optical tweezers experiments were carried out on a home-built single-trap instrument (Smith et al., 2003). All optical tweezers experiments were performed in buffer HKMβ. For G-domain refolding experiments, the protocol was set to 2-50 pN with 10 seconds folding time at 2 pN. The trap was moved at a constant velocity of 100 nm/s. For domain II folding quantification, the protocol was set to 2-20 pN with 10 seconds folding time at 2 pN. For determining the kinetics of G-domain denaturation, the protocol was set to 2-20 pN or 2-30 pN with 10 seconds folding time at 2 pN. All force ramp data was collected with a sampling rate of 1000 Hz and time-averaged to 33 Hz for plotting.
Our instrument independently records the trap position and the force on the tethered molecule (see fig. S7). After recording the initial unfolding transition, the force ramp program is paused during relaxation, and the position of the micropipette is adjusted to bring the two beads into the same plane (fig. S7A). Subsequently, the force ramp program is restarted, and force extension curves are collected until the tether breaks (fig. S7B).
Optical tweezers force clamp experiments
Prior to force-clamp measurements, we subjected each tethered molecule to several force ramp cycles to verify that it exhibited the expected characteristic unfolding signature. In force clamp measurements, tethered proteins or RNCs were first stretched to 30 pN until unfolding was observed. Subsequently, the force was set to 3.5 pN in one step. While holding the molecule at this force, we followed changes in molecular extension over time to monitor the folding process of the molecules at a sampling frequency of 1000 Hz. After refolding of the G-domain, the process of unfolding at 30 pN and refolding at 3.5 pN was repeated. If refolding was not observed within 120 seconds, we reset the system by increasing the force to 30 pN, ensuring complete unfolding, before lowering the force again to 3.5 pN.
For comparing extension histograms from force clamp measurements of several molecules (Fig. 2E), the state containing the folded G-domain was used as the reference state and set to an extension of 0 nm. Raw extension traces were aligned to this reference state, binned (bin size: 0.25 nm) and plotted. The lines in Fig. 2E are kernel density estimates, plotted on top of the actual extension histogram.
QUANTIFICATION AND STATISTICAL ANALYSIS.
Force ramp raw data processing
Determination of unfolding forces and length changes is carried out using raw data without further processing. For the purpose of plotting data into figures as force extension curves, we remove mechanical drift of the instrument and convert the recorded trap position to relative molecular extension (as shown in fig. S7). To correct for slow drift of the micropipette during the measurement, we determine the mean trap position after complete unfolding of the molecule within a narrow window of high forces (typically around 48 pN; fig. S7D). Because the molecule is fully unfolded at this force, variations in the recorded extension are not due to different structural states, but due to mechanical drift. The mean trap position is then used to align traces, removing drift (fig. S7E). The trap position is then converted into relative molecular extension, xrel, according to xrel = xtrap − F/ktrap, where xtrap is the recorded trap position, F is the force, and ktrap is the independently determined trap stiffness (typically 0.1 to 0.12 pN/nm). We note that the analysis of structural states and unfolding transitions (fig. S2) is carried out using the unprocessed raw data, and that it is independent of the data manipulations described here, which is carried out solely for visually representing force extension curves.
Unfolding transition analysis (force ramp experiments)
During force ramp experiments, we continuously moved the optical trap, resulting in a continuously increasing force on the tethered molecule. The unfolding rate increases exponentially with the applied force and therefore continuously increases in this type of experiment until the molecule ultimately unfolds. Unfolding transitions are apparent as “rips” in the force extension curves (FECs). Each rip is characterized by an unfolding force (Funf) and a change in molecular extension (Δxunf) (fig. S2). As the protein unfolds, it transitions from a stiff, compactly folded structure to a soft, extended polypeptide. As a consequence, the stiffness of the tether decreases, while simultaneously the contour length increases, causing a drop in the force. Subsequently, the force increases again because the trap continues to move.
Because the lifetimes of a given folded structure at any given force are stochastic, unfolding does not occur at a single discrete force in single-molecule force ramp experiments. Instead, the unfolding forces for a particular structure follow a characteristic distribution (Dudko et al., 2008) (see fig. S5F for an example). We determined the Funf by locating discontinuities in the FECs using a custom Matlab script. To determine Δxunf, we measured the difference in extension before and after the rip at Funf (fig. S2B). Because the extensions are measured at the same force, parts of the tether that do not change (e.g. the ribosome, domains that remain folded, DNA handles) have no effect on the measured extension change. The measured change therefore reflects only the contribution from the part of the polypeptide that has unfolded in a particular transition. The measured extension change is a relative change, i.e. it is independent of the absolute length of the tether.
For the G-domain and domain II, unfolding intermediates are apparent in some, but not all unfolding events. This apparent heterogeneity is observed for all constructs containing the foldable domains (G-domain: all constructs used in this study; domain II: full-length EF-G, G-II and 452RNC). The intermediates had short lifetimes, typically in the millisecond range. The reason for resolving the unfolding intermediates in only a fraction of FECs is likely due to the finite time resolution of our measurements (1 ms sampling rate). We only considered states that were visited for at least 5 samples (5 ms). Assuming a lifetime of the intermediate in the millisecond range and the stochastic distribution of lifetimes, these intermediates are then resolved in only a fraction of the FECs, explaining the apparent heterogeneity in the unfolding transitions for the G-domain and domain II.
When the intermediate is resolved, unfolding usually proceeds in two steps that usually occur in rapid succession. If it is not possible to obtain a direct measurement of the extension change for the first transition (e.g. as in panels iii and iv, fig. S2C), we determined the unfolding forces for each transition, Funf,1 and Funf,2, as well as the extension changes for both transitions combined (Δxunf,total = Δxunf,1 + Δxunf,2) and for the second transition (Δxunf,2) (see fig. S2C). Δxunf,1 is then calculated as Δxunf,1 = Δxunf,total − Δxunf,2.
Structure-based interpretation of unfolding transitions
We used the structure of E. coli EF-G (pdb code: 4v9p) to interpret the measured extension changes upon mechanical unfolding. The native state extension, xfolded, was determined from the Cα coordinates of the first and last residue in the folded structure. For the G-domain, encompassing residues 1 to 293, the native state extension determined from the 4v9p structure is xfolded (G) = 2.5 nm. The contour length of the fully unfolded G-domain, which has naa = 293 amino acids, is Lunfolded(G) = naa * 0.36 nm/aa = 105.5 nm. The expected contour length change for unfolding of the G-domain is therefore
The experimentally determined average contour length change for unfolding of the Galone construct is ΔL(Galone) = 103.2 nm ± 1.8 nm. The calculated and observed values are very similar, indicating complete unfolding of the natively folded G-domain when we pulled on Galone.
When unfolding occurs in two steps, the structure remaining after the first step rapidly re-orients itself along the axis of applied force. This is important when considering unfolding of domain II in the two-domain G-II construct. The native state extension of G-II (aa 1 to 410), calculated from the EF-G structure, is xnative(GNIIN) = 3.5 nm. After domain II unfolding, the resulting state (GNIIU) has a contour length of
Again considering the states prior to and after unfolding, the expected contour length change for domain II unfolding in the G-II construct is
which is close to the experimentally determined value of ΔL(IIG-II) = 41.2 nm ± 1.9 nm.
In 39% of events, domain II unfolding occurs in two steps. When the interface between the G-domain and domain II is disrupted, a loop region at the beginning of domain II (aa 294-317; see Fig. 3) likely unfolds. The resulting unfolding intermediate Iunf quickly transitions to GfoldedIIunfolded. Iunf consists of the folded G-domain, the now unfolded loop and the remaining core structure of domain II (aa 318-410). In this case, the extension of the species after the first transition is
The length change associated with the first transition then is
and for the second transition,
To compare the experimentally determined extension changes to the expected contour length changes, we used the WLC model (Bustamante et al., 1994) to convert extension changes into contour length changes according to
using a Boltzmann constant of kB = 0.0138065 pN*nm/K, a temperature of T = 296 K, a persistence length of p = 0.65 nm, and a contour length of L = 0.36 nm/aa * naa.
The experimentally measured values of ΔL(step 1) = 9.6 nm ± 1.6 nm and ΔL(step 2) = 31.9 nm ± 1.4 nm are in excellent agreement with the calculated values for the unfolding scenario described above. While other unfolding pathways are possible, this result strongly suggests that unfolding of domain II in the G-II construct proceeds by first breaking the domain interface and releasing the loop region in step 1, and then unfolding the remaining core structure of domain II in step 2.
Interpretation of force clamp refolding experiments
In force clamp measurements, the unfolded polypeptide refolds against a constant force. Changes in extension, monitored over time, reflect structural transitions in the polypeptide. At the beginning of the trace shown in Fig. 2A, the polypeptide is in the unfolded state (GU-IIU), at the end, the G-domain has folded (GN-IIU). In addition, the molecule populates several partially folded states with extensions between these two states, as well as a state that is shorter than GN-IIU by 2.5 nm. Because this short state does not directly transition to the GN-IIU state, we interpret it to be a misfolded state:
In force clamp measurements, the observed difference in extension is the difference in end-to-end distance between the initial and the final states:
In the case of the initial and final state during G-II refolding measurements (fig. S4A), the observed change is
The extension of GU-IIU depends only on force, which is constant in this experiment. The shorter extension of the misfolded state must therefore be due to a change in the second term. The extension of GN-IIU is the sum of the extensions of the two domains:
The larger observed extension change for the misfolded state could in principle be due to a smaller x(GN), i.e. the G-domain adopts a state with a shorter end-to-end distance. However, the end-to-end distance of the natively folded G-domain is x(GN) = 0.86 nm. A change in x(GN) therefore cannot account for an observed difference of 2.5 nm. Thus, the state with the misfolded state must involve both domains (fig. S4B).
Changes in extension by TF binding
Prior to folding, the extension of the 452RNC nascent chain is shorter by ~9 nm in the presence of TF, compared to the unfolded state in the absence of the chaperone (Fig. 2D and E). NMR studies of TF binding to PhoA (Saio et al. 2014) indicate that multiple interaction sites in the chaperone sequester up to 140 amino acid of unfolded substrate protein. The substrate binding sites in the TF structure are within ~5 nm. The extension of an unfolded 140 aa polypeptide at 3.5 pN is 15.1 nm (calculated with a WLC model with a persistence length of 0.65 nm). It is therefore expected that this mode of TF binding causes the end-to-end distance of an unfolded 140 aa polypeptide at 3.5 pN to decrease by about 10 nm (from 15.1 nm to 5 nm), even though the substrate protein remains unfolded. The reduced extension of the 452RNC nascent chain in the presence of TF is consistent with this scenario and might thus be due to binding of the nascent chain to multiple sites on TF.
Folding Cycle Analysis
To determine refolding probabilities from force ramp experiments, we subjected proteins or RNCs to repeated cycles of denaturation (pulling to the maximum force, see above) and refolding (relaxing to a force of 2 pN and holding that force for 10 seconds). After initial denaturation, we counted the number of cycles until refolding occurred (fig. S3). From a large number (>100) of cycles, we obtained a distribution of refolding events as a function of cycle number and converted it into a probability distribution. This distribution should follow a geometric distribution, assuming that folding occurs with a constant probability within any one cycle. Since we have a limited amount of data (i.e., coverage over all possible cycle numbers is finite), we use the following function to fit our data:
where Pi is the probability of waiting i cycles to observe folding, p is the folding probability within one cycle, and α is a scaling factor to account for incomplete sampling of the geometric distribution. Therefore, the cumulative distribution for the first n cycles is
where Pn is the cumulative probability up to cycle n.
Folding rate estimation
To estimate apparent folding rates (“Apparent rate” in Fig. 1C and Fig. 2F) from different proteins and RNCs from the folding probability p obtained from FCA, we used the first order approximation
where k is the first order apparent rate constant, p is the folding probability within one cycle, and t is the folding time, which equals 10 seconds in our study. In constant force refolding measurements, we observed multiple intermediate states during G-domain folding at 3.5 pN. The refolding therefore is not 2-state, and the first-order approximation represents an oversimplification. However, refolding times from force clamp measurements are well described by a single-exponential process (data not shown), indicating that the simplified assumption of a single rate-limiting step yields a reasonable estimate of the overall folding rate. In addition, the distribution of folding events along repeated force ramp cycles is well described by the geometric distribution (fig. S3D and E).
G-domain denaturation and II-folding probability estimation
Refolding of domain II and denaturation of the G-domain by interactions with domain II are both slow processes. As a consequence, the available dataset is limited. In addition, the two types of events are mutually exclusive. We therefore modified our analysis to estimate the probabilities. Assuming that the number of either event D(n) (D1(n) for G-domain denaturation, and D2(n) for domain II folding) occurring after n cycles is described by a Poisson distribution, the maximum likelihood probability Pimax of either event happening within one cycle is
where #events(i) is the number of observed events of either G-domain denaturation (i=1) or domain II folding (i=2), and N0 is total number of cycles in which we do not observe either event before tether breaking. Using this approach, we estimate that the probability of G-domain denaturation in any cycle is P1max = 0.144, and that of domain II folding is P2max = 0.10.
Binomial test
To assess whether Galone spontaneously undergoes denaturation in the absence of domain II, we conducted control experiments in which we subjected Galone to the same force ramp conditions as G-II to detect possible denaturation. From total of 7 molecules with 399 trials, we observed no denaturation events with Galone. A binomial test with a denaturation probability per cycle of 0.144, which is estimated from G-domain denaturation experiments performed with G-II, yielded a p-value of 1.1×10−27. It is thus extremely unlikely that Galone exhibits the denaturation observed with G-II. Domain II therefore is responsible for pulling the G-domain out of its native state. A similar analysis was performed to confirm that we did not miss G-domain denaturation in the presence of TF, which is also very unlikely (p-value: 5.3×10−12).
Kolmogorov-Smirnov test (K-S test)
A one dimensional two-sample K-S test (Massey, 1951) was performed to evaluate whether initial unfolding transitions of domain II are different from those obtained after refolding. We compared the distributions of unfolding forces and contour length changes separately. In both cases, the results suggest that it is very likely that we are sampling the same underlying distribution, which indicates that the structure of domain II is the same in both cases (fig. S6A and B). In addition, we conducted a two-dimensional two-sample K-S test (Peacock, 1983) to compare unfolding events (each characterized by unfolding force and extension change), which confirmed that the distributions for native and refolded domain II are not significantly different (fig. S6C).
The two-dimensional two-sample K-S test was also performed to evaluate whether the transitions from denatured G-domain are different from those of G-II or 328RNC (fig. S6D and E). The results suggest that the distributions for G-II and 328RNC are distinct, but neither one is different from that of the denatured G-domain. This observation suggests that denaturation of the G-domain leads to the population of molten globule-like and partially folded states (similar to those populated during 328RNC refolding) and G-domain/domain II misfolded states (similar to those populated during G-II refolding after complete mechanical unfolding). It therefore appears that denaturation of the G-domain by domain II leads to complete unfolding via the intermediates that are observed during refolding of 328RNC.
DATA AND SOFTWARE AVAILABILITY:
Data and scripts for data analysis are available upon request.
Key resources table:
Reagent or Resource | Source | Identifier |
---|---|---|
Bacterial Strains | ||
Escherichia coli BL21-Gold (DE3) | Agilent Technologies | Cat#230132 |
Chemicals, Peptides, and Recombinant Proteins | ||
EF-G (purified protein) | This paper | N/A |
Galone (purified protein) | This paper | N/A |
G-II (purified protein) | This paper | N/A |
TF (purified protein) | This paper | N/A |
BirA (purified protein) | This paper | N/A |
Sfp (purified protein) | This paper | N/A |
TEV protease (purified protein) | This paper | N/A |
Ulp1 protease (purified protein) | This paper | N/A |
T4 DNA ligase | New England Biolabs | M0202 |
RNase A | ThermoFisher | Cat#EN0531 |
PURExpress ΔRibosome Kit | New England Biolabs | Cat#E3313S |
T7 MegaScript Kit | Ambion | Cat#AM1333 |
MEGAclear Kit | Ambion | Cat#AM1908 |
EDC | ThermoFisher | Cat#22980 |
Sulfo-NHS | ThermoFisher | Cat#24510 |
D-Biotin | ThermoFisher | Cat#B1595 |
Coenzyme A trilithium salt | Sigma-Aldrich | Cat#C3019 |
Ribosomes | (Kaiser et al., 2011) | N/A |
Oligonucleotides | ||
Oligonucleotides for DNA handles, see Table S1 | IDT DNA | N/A |
Primers for RNC generation, see Table S2 | IDT DNA | N/A |
Recombinant DNA | ||
plasmid: pCK_Avi_EFG_y01 | This paper | N/A |
plasmid: pCK_sAvi_EFG_y01 | This paper | N/A |
plasmid: pCK_sAvi_G_294_y01 | This paper | N/A |
plasmid: pCK_sAvi_GII_410_y01 | This paper | N/A |
plasmid: pCK_TF3B | (Kaiser et al., 2006) | N/A |
Software and Algorithms | ||
MATLAB | Mathworks | R2017b |
Supplementary Material
2
fig. S1. Overview of constructs used in the present study. Related to Figs. Figs.11–6.
fig. S2. Characterization of states populated during G-domain refolding. Related to Fig. 1.
fig. S3 Folding cycle analysis of G-domain folding. Related to Fig. 1
fig. S4 Interpretation of extension changes from force clamp experiments. Related to Fig. 2.
fig. S5. Folding signatures of the G-domain and domain II. Related to Fig. 3 and and44.
fig. S6. Domain II refolding and G-domain denaturation. Related to Fig. 4 and and55.
fig. S7. Raw data and data processing. Related to Fig. 1.
Table s1: DNA oligonucleotides for generating EF-G RNC templates. Related to STAR Methods.
Table s2: DNA oligonucleotides for generating DNA handles. Related to STAR Methods.
ACKNOWLEDGMENTS.
We thank members of the Kaiser and Hilser labs for helpful discussions. C.M.K. acknowledges funding from the NIH (5R01GM121567) and the Pew Scholars Program. K.M. acknowledges support from the CMDB graduate program (NIH 5T32GM007231).
Footnotes
Publisher's Disclaimer: This is a PDF file of an unedited manuscript that has been accepted for publication. As a service to our customers we are providing this early version of the manuscript. The manuscript will undergo copyediting, typesetting, and review of the resulting proof before it is published in its final citable form. Please note that during the production process errors may be discovered which could affect the content, and all legal disclaimers that apply to the journal pertain.
DECLARATION OF INTERESTS.
The authors declare no competing interests.
REFERENCES.
- AEvarsson A, Brazhnikov E, Garber M, Zheltonosova J, Chirgadze Y, al-Karadaghi S, Svensson LA, and Liljas A (1994). Three-dimensional structure of the ribosomal translocase: elongation factor G from Thermus thermophilus. The EMBO journal 13, 3669–3677. [Europe PMC free article] [Abstract] [Google Scholar]
- Agashe VR, Guha S, Chang HC, Genevaux P, Hayer-Hartl M, Stemp M, Georgopoulos C, Hartl FU, and Barral JM (2004). Function of trigger factor and DnaK in multidomain protein folding: increase in yield at the expense of folding speed. Cell 117, 199–209. [Abstract] [Google Scholar]
- Anfinsen CB (1973). Principles that govern the folding of protein chains. Science 181, 223–230. [Abstract] [Google Scholar]
- Batey S, Nickson AA, and Clarke J (2008). Studying the folding of multidomain proteins. HFSP J 2, 365–377. [Europe PMC free article] [Abstract] [Google Scholar]
- Beckett D, Kovaleva E, and Schatz PJ (1999). A minimal peptide substrate in biotin holoenzyme synthetase-catalyzed biotinylation. Protein Sci 8, 921–929. [Europe PMC free article] [Abstract] [Google Scholar]
- Braselmann E, Chaney JL, and Clark PL (2013). Folding the proteome. Trends in biochemical sciences 38, 337–344. [Europe PMC free article] [Abstract] [Google Scholar]
- Bremer H, and Dennis PP (2008). Modulation of Chemical Composition and Other Parameters of the Cell at Different Exponential Growth Rates. EcoSal Plus 3. [Abstract] [Google Scholar]
- Brockwell DJ, and Radford SE (2007). Intermediates: ubiquitous species on folding energy landscapes? Curr Opin Struct Biol 17, 30–37. [Europe PMC free article] [Abstract] [Google Scholar]
- Buhr F, Jha S, Thommen M, Mittelstaet J, Kutz F, Schwalbe H, Rodnina MV, and Komar AA (2016). Synonymous Codons Direct Cotranslational Folding toward Different Protein Conformations. Mol Cell 61, 341–351. [Europe PMC free article] [Abstract] [Google Scholar]
- Bustamante C, Marko JF, Siggia ED, and Smith S (1994). Entropic elasticity of lambda-phage DNA. Science 265, 1599–1600. [Abstract] [Google Scholar]
- Cabrita LD, Cassaignau AM, Launay HM, Waudby CA, Wlodarski T, Camilloni C, Karyadi ME, Robertson AL, Wang X, Wentink AS, et al. (2016). A structural ensemble of a ribosome-nascent chain complex during cotranslational protein folding. Nat Struct Mol Biol 23, 278–285. [Europe PMC free article] [Abstract] [Google Scholar]
- Cecconi C, Shank EA, Bustamante C, and Marqusee S (2005). Direct observation of the three-state folding of a single protein molecule. Science 309, 2057–2060. [Abstract] [Google Scholar]
- Chaney JL, Steele A, Carmichael R, Rodriguez A, Specht AT, Ngo K, Li J, Emrich S, and Clark PL (2017). Widespread position-specific conservation of synonymous rare codons within coding sequences. PLoS Comput Biol 13, e1005531. [Europe PMC free article] [Abstract] [Google Scholar]
- Czworkowski J, Wang J, Steitz TA, and Moore PB (1994). The crystal structure of elongation factor G complexed with GDP, at 2.7 A resolution. The EMBO journal 13, 3661–3668. [Europe PMC free article] [Abstract] [Google Scholar]
- Deuerling E, Patzelt H, Vorderwulbecke S, Rauch T, Kramer G, Schaffitzel E, Mogk A, Schulze-Specking A, Langen H, and Bukau B (2003). Trigger Factor and DnaK possess overlapping substrate pools and binding specificities. Mol Microbiol 47, 1317–1328. [Abstract] [Google Scholar]
- Dill KA, and MacCallum JL (2012). The protein-folding problem, 50 years on. Science 338, 1042–1046. [Abstract] [Google Scholar]
- Dudko OK, Hummer G, and Szabo A (2008). Theory, analysis, and interpretation of single-molecule force spectroscopy experiments. Proc Natl Acad Sci U S A 105, 15755–15760. [Europe PMC free article] [Abstract] [Google Scholar]
- Fedorov AN, and Baldwin TO (1997). Cotranslational protein folding. J Biol Chem 272, 32715–32718. [Abstract] [Google Scholar]
- Flaugh SL, Kosinski-Collins MS, and King J (2005). Contributions of hydrophobic domain interface interactions to the folding and stability of human gammaD-crystallin. Protein Sci 14, 569–581. [Europe PMC free article] [Abstract] [Google Scholar]
- Frydman J, Erdjument-Bromage H, Tempst P, and Hartl FU (1999). Co-translational domain folding as the structural basis for the rapid de novo folding of firefly luciferase. Nat Struct Biol 6, 697–705. [Abstract] [Google Scholar]
- Genevaux P, Keppel F, Schwager F, Langendijk-Genevaux PS, Hartl FU, and Georgopoulos C (2004). In vivo analysis of the overlapping functions of DnaK and trigger factor. EMBO Rep 5, 195–200. [Europe PMC free article] [Abstract] [Google Scholar]
- Haldar S, Tapia-Rojo R, Eckels EC, Valle-Orero J, and Fernandez JM (2017). Trigger factor chaperone acts as a mechanical foldase. Nat Commun 8, 668. [Europe PMC free article] [Abstract] [Google Scholar]
- Han JH, Batey S, Nickson AA, Teichmann SA, and Clarke J (2007). The folding and evolution of multidomain proteins. Nat Rev Mol Cell Biol 8, 319–330. [Abstract] [Google Scholar]
- Hoffmann A, Becker AH, Zachmann-Brand B, Deuerling E, Bukau B, and Kramer G (2012). Concerted action of the ribosome and the associated chaperone trigger factor confines nascent polypeptide folding. Mol Cell 48, 63–74. [Abstract] [Google Scholar]
- Holtkamp W, Kokic G, Jager M, Mittelstaet J, Komar AA, and Rodnina MV (2015). Cotranslational protein folding on the ribosome monitored in real time. Science 350, 1104–1107. [Abstract] [Google Scholar]
- Jahn M, Buchner J, Hugel T, and Rief M (2016). Folding and assembly of the large molecular machine Hsp90 studied in single-molecule experiments. Proc Natl Acad Sci U S A. [Europe PMC free article] [Abstract] [Google Scholar]
- Kaiser CM, Chang HC, Agashe VR, Lakshmipathy SK, Etchells SA, Hayer-Hartl M, Hartl FU, and Barral JM (2006). Real-time observation of trigger factor function on translating ribosomes. Nature 444, 455–460. [Abstract] [Google Scholar]
- Kaiser CM, Goldman DH, Chodera JD, Tinoco I Jr., and Bustamante C (2011). The ribosome modulates nascent protein folding. Science 334, 1723–1727. [Europe PMC free article] [Abstract] [Google Scholar]
- Kaiser CM, and Liu K (2018). Folding up and Moving on—Nascent Protein Folding on the Ribosome. Journal of Molecular Biology 430, 4580–4591. [Europe PMC free article] [Abstract] [Google Scholar]
- Kim YE, Hipp MS, Bracher A, Hayer-Hartl M, and Hartl FU (2013). Molecular chaperone functions in protein folding and proteostasis. Annual Review of Biochemistry 82, 323–355. [Abstract] [Google Scholar]
- Kramer G, Boehringer D, Ban N, and Bukau B (2009). The ribosome as a platform for co-translational processing, folding and targeting of newly synthesized proteins. Nat Struct Mol Biol 16, 589–597. [Abstract] [Google Scholar]
- Kramer G, Rauch T, Rist W, Vorderwulbecke S, Patzelt H, Schulze-Specking A, Ban N, Deuerling E, and Bukau B (2002). L23 protein functions as a chaperone docking site on the ribosome. Nature 419, 171–174. [Abstract] [Google Scholar]
- Liu K, Rehfus JE, Mattson E, and Kaiser CM (2017). The ribosome destabilizes native and non-native structures in a nascent multidomain protein. Protein Sci 26, 1439–1451. [Europe PMC free article] [Abstract] [Google Scholar]
- Mashaghi A, Kramer G, Bechtluft P, Zachmann-Brand B, Driessen AJ, Bukau B, and Tans SJ (2013). Reshaping of the conformational search of a protein by the chaperone trigger factor. Nature 500, 98–101. [Abstract] [Google Scholar]
- Mashaghi A, Mashaghi S, and Tans SJ (2014). Misfolding of luciferase at the single-molecule level. Angew Chem Int Ed Engl 53, 10390–10393. [Abstract] [Google Scholar]
- Massey FJ (1951). The Kolmogorov-Smirnov Test for Goodness of Fit. J Am Stat Assoc 46, 68–78. [Google Scholar]
- Mercier E, and Rodnina MV (2018). Co-Translational Folding Trajectory of the HemK Helical Domain. Biochemistry. [Abstract] [Google Scholar]
- Mossessova E, and Lima CD (2000). Ulp1-SUMO crystal structure and genetic analysis reveal conserved interactions and a regulatory element essential for cell growth in yeast. Mol Cell 5, 865–876. [Abstract] [Google Scholar]
- Motlagh HN, Wrabl JO, Li J, and Hilser VJ (2014). The ensemble nature of allostery. Nature 508, 331–339. [Europe PMC free article] [Abstract] [Google Scholar]
- Nicola AV, Chen W, and Helenius A (1999). Co-translational folding of an alphavirus capsid protein in the cytosol of living cells. Nat Cell Biol 1, 341–345. [Abstract] [Google Scholar]
- Peacock JA (1983). Two-Dimensional Goodness-of-Fit Testing in Astronomy. Mon Not R Astron Soc 202, 615–627. [Google Scholar]
- Pechmann S, and Frydman J (2013). Evolutionary conservation of codon optimality reveals hidden signatures of cotranslational folding. Nat Struct Mol Biol 20, 237–243. [Europe PMC free article] [Abstract] [Google Scholar]
- Pulk A, and Cate JH (2013). Control of ribosomal subunit rotation by elongation factor G. Science 340, 1235970. [Europe PMC free article] [Abstract] [Google Scholar]
- Saio T, Guan X, Rossi P, Economou A, and Kalodimos CG (2014). Structural basis for protein antiaggregation activity of the trigger factor chaperone. Science 344, 1250494. [Europe PMC free article] [Abstract] [Google Scholar]
- Samelson AJ, Jensen MK, Soto RA, Cate JH, and Marqusee S (2016). Quantitative determination of ribosome nascent chain stability. Proc Natl Acad Sci U S A 113, 13402–13407. [Europe PMC free article] [Abstract] [Google Scholar]
- Scholl ZN, Yang W, and Marszalek PE (2017). Competing Pathways and Multiple Folding Nuclei in a Large Multidomain Protein, Luciferase. Biophys J 112, 1829–1840. [Europe PMC free article] [Abstract] [Google Scholar]
- Shank EA, Cecconi C, Dill JW, Marqusee S, and Bustamante C (2010). The folding cooperativity of a protein is controlled by its chain topology. Nature 465, 637–640. [Europe PMC free article] [Abstract] [Google Scholar]
- Smith SB, Cui Y, and Bustamante C (2003). Optical-trap force transducer that operates by direct measurement of light momentum. Methods Enzymol 361, 134–162. [Abstract] [Google Scholar]
- Sosnick TR, and Barrick D (2011). The folding of single domain proteins--have we reached a consensus? Current opinion in structural biology 21, 12–24. [Europe PMC free article] [Abstract] [Google Scholar]
- Stigler J, Ziegler F, Gieseke A, Gebhardt JC, and Rief M (2011). The complex folding network of single calmodulin molecules. Science 334, 512–516. [Abstract] [Google Scholar]
- Thommen M, Holtkamp W, and Rodnina MV (2017). Co-translational protein folding: progress and methods. Curr Opin Struct Biol 42, 83–89. [Abstract] [Google Scholar]
- Walters BT, Mayne L, Hinshaw JR, Sosnick TR, and Englander SW (2013). Folding of a large protein at high structural resolution. Proc Natl Acad Sci U S A 110, 18898–18903. [Europe PMC free article] [Abstract] [Google Scholar]
- Yin J, Lin AJ, Golan DE, and Walsh CT (2006). Site-specific protein labeling by Sfp phosphopantetheinyl transferase. Nat Protoc 1, 280–285. [Abstract] [Google Scholar]
- Yu H, Liu X, Neupane K, Gupta AN, Brigley AM, Solanki A, Sosova I, and Woodside MT (2012). Direct observation of multiple misfolding pathways in a single prion protein molecule. Proc Natl Acad Sci U S A 109, 5283–5288. [Europe PMC free article] [Abstract] [Google Scholar]
- Zhang G, Hubalewska M, and Ignatova Z (2009). Transient ribosomal attenuation coordinates protein synthesis and co-translational folding. Nat Struct Mol Biol 16, 274–280. [Abstract] [Google Scholar]
- Zoldak G, and Rief M (2013). Force as a single molecule probe of multidimensional protein energy landscapes. Current opinion in structural biology 23, 48–57. [Abstract] [Google Scholar]
Full text links
Read article at publisher's site: https://doi.org/10.1016/j.molcel.2019.01.043
Read article for free, from open access legal sources, via Unpaywall:
http://www.cell.com/article/S1097276519300632/pdf
Citations & impact
Impact metrics
Article citations
Resolving chaperone-assisted protein folding on the ribosome at the peptide level.
Nat Struct Mol Biol, 10 Jul 2024
Cited by: 2 articles | PMID: 38987455
AutoRNC: An automated modeling program for building atomic models of ribosome-nascent chain complexes.
Structure, 32(5):621-629.e5, 29 Feb 2024
Cited by: 0 articles | PMID: 38428431
Life under tension: the relevance of force on biological polymers.
Biophys Rep, 10(1):48-56, 01 Feb 2024
Cited by: 0 articles | PMID: 38737478 | PMCID: PMC11079598
Folding pathway of a discontinuous two-domain protein.
Nat Commun, 15(1):690, 23 Jan 2024
Cited by: 0 articles | PMID: 38263337 | PMCID: PMC10805907
Folding correctors can restore CFTR posttranslational folding landscape by allosteric domain-domain coupling.
Nat Commun, 14(1):6868, 27 Oct 2023
Cited by: 4 articles | PMID: 37891162 | PMCID: PMC10611759
Go to all (44) article citations
Data
Data behind the article
This data has been text mined from the article, or deposited into data resources.
BioStudies: supplemental material and supporting data
Protein structures in PDBe
-
(2 citations)
PDBe - 4v9pView structure
Similar Articles
To arrive at the top five similar articles we use a word-weighted algorithm to compare words from the Title and Abstract of each citation.
The ribosome destabilizes native and non-native structures in a nascent multidomain protein.
Protein Sci, 26(7):1439-1451, 19 May 2017
Cited by: 29 articles | PMID: 28474852 | PMCID: PMC5477528
Energetic dependencies dictate folding mechanism in a complex protein.
Proc Natl Acad Sci U S A, 116(51):25641-25648, 27 Nov 2019
Cited by: 20 articles | PMID: 31776255 | PMCID: PMC6925980
Synthesis runs counter to directional folding of a nascent protein domain.
Nat Commun, 11(1):5096, 09 Oct 2020
Cited by: 10 articles | PMID: 33037221 | PMCID: PMC7547688
How Does the Ribosome Fold the Proteome?
Annu Rev Biochem, 89:389-415, 01 Jun 2020
Cited by: 38 articles | PMID: 32569518
Review
Funding
Funders who supported this work.
CMDB
NIGMS NIH HHS (2)
Grant ID: T32 GM007231
Grant ID: R01 GM121567
NIH (2)
Grant ID: 5R01GM121567
Grant ID: 5T32GM007231