Abstract
Free full text

Dissociation between Rewarding and Psychomotor Effects of Opiates: Differential Roles for Glutamate Receptors within Anterior and Posterior Portions of the Ventral Tegmental Area
Abstract
The rewarding effects of drugs of abuse are thought to be dependent on the mesocorticolimbic dopamine system, which originates in the ventral tegmental area (VTA) and projects into the nucleus accumbens (NAC) and other forebrain regions. Heroin, by inhibiting GABAergic interneurons in the VTA, induces local dopaminergic activation and release in the NAC terminals. The role of other basic neurotransmitter systems, such as glutamate in the VTA, in mediating the rewarding effect of addictive drugs, is less established. We explored whether blockade of glutamate receptors in subregions of the VTA modulate the rewarding properties and/or the development of psychomotor changes induced by opiates. Administration of 6-cyano-7-nitroquinoxaline-2,3-dione (CNQX; an AMPA/kainate receptor antagonist) into the anterior VTA blocked the rewarding effects of opiates in both the conditioned place preference and the self-administration paradigms without affecting the gradual increase of the psychomotor response to opiates. In contrast, administration of CNQX into the posterior VTA did not affect the rewarding properties of opiates, but blocked the initial sedative effect of opiates and the gradual increase of the psychomotor response to the drug. These findings suggest a critical role for glutamate receptors in the VTA in opiate reward, as well as behavioral and anatomical dissociation between the rewarding and psychomotor effects of opiates.
Introduction
Opiates are highly addictive drugs, whose rewarding effects were suggested to be partly mediated by suppression of GABA inhibitory inputs to dopamine (DA) neurons in the ventral tegmental area (VTA), thereby potentiating DA release in the nucleus accumbens (NAC) (Johnson and North, 1992). Addiction research has focused mainly on mechanisms involving the mesocorticolimbic DA system. The critical role of the mesocorticolimbic DA system in both reward function and psychomotor stimulation provides a basis for the psychomotor stimulant theory of addiction (Wise and Bozarth, 1987), which suggests that all positive reinforcers activate a common biologic mechanism associated with approach behaviors. However, glutamate transmission has also been implicated in various drug addiction processes (Tzschentke and Schmidt, 2003). Glutamatergic inputs to the VTA arising from the prefrontal cortex and laterodorsal tegmentum/pedunculopontine (Charara et al., 1996; Carr and Sesack, 2000) are important for driving the activity of DA neurons in the VTA (Wolf et al., 2004) and influence various physiologic and behavioral states mediated by the mesocorticolimbic pathway. For example, glutamate mediates the switch from a pacemaker-like firing to a burst-firing pattern of the DA neurons in the VTA (Gariano and Groves, 1988). Moreover, DA neurons react more intensely and more readily to excitatory inputs under the influence of drugs of abuse. For example, acute cocaine (Ungless et al., 2001) or nicotine (Mansvelder and McGehee, 2000; Schultz, 2002) exposure increases long-term potentiation in DA neurons of the VTA, which might be because of upregulation of glutamatergic receptors in the VTA, induced by repeated drug exposure (Fitzgerald et al., 1996; Saal et al., 2003). In addition, several studies indicate that the association of environmental cues with drug reinforcement is mediated by glutamate-related plasticity within the mesocorticolimbic system (Hyman and Malenka, 2001).
The importance of VTA AMPA/kainate glutamatergic receptors in establishing an association between morphine reinforcement and a specific environment was demonstrated in a study by Harris et al. (2004), in which microinjection of the AMPA/kainate antagonist 6-cyano-7-nitroquinoxaline-2,3-dione (CNQX) into the VTA blocked conditioned place preference (CPP) induced by morphine (Harris et al., 2004).
However, the VTA is not homogenous, anatomically and functionally, and can be divided into two subregions (Ikemoto et al., 1997, 1998; Ikemoto and Wise, 2002; Zangen et al., 2002; Zangen et al., 2006). For example, CPP can be established by microinjecting endomorphin-1 (a μ-opioid peptide) into the posterior, but not the anterior, portion of the VTA (Zangen et al., 2002). In addition, studies using viral induced modifications indicate that the anterior VTA (aVTA) and posterior VTA (pVTA) play different roles in modulating the rewarding properties of opiates and cocaine (Carlezon et al., 2000; Bolaños et al., 2003; Olson et al., 2005). Hence, overexpression of GluR1 (an AMPA receptor subunit) in the aVTA increases the rewarding effect of morphine, whereas its overexpression in the pVTA makes morphine aversive as indicated in the CPP paradigm (Carlezon et al., 2000).
The present study investigated the effects of CNQX-induced glutamatergic blockade in the anterior and posterior VTA on different properties of opiate addiction using CPP, self-administration (SA), and behavioral sensitization paradigms.
Materials and Methods
Animals
Male Sprague Dawley rats (n = 195; Harlan Laboratories) were used for the experiments. The rats weighed 280–340 g at the time of surgery and were housed individually after surgery on a 12 h light/dark schedule (lights on at 7:00 A.M.) with food and water available ad libitum. All experiments were conducted during the light phase of the cycle. All experiments were conducted in accordance with the guidelines of the Institutional Care and Use Committee of the Weizmann Institute of Science.
Intracranial and intravenous surgeries
For the microinjection procedure, stainless-steel guide cannulas (24 gauge; Plastics One) were implanted bilaterally while rats were under ketamine (170 mg/kg) and Promace (1.7 mg/kg) anesthesia. Each animal was bilaterally implanted with guide cannulas that ended 1.5 mm above the target site. The cannulas were inserted at 6° (left side) and 10° (right side) angles toward the midline for sites in the anterior or posterior VTA (Zangen et al., 2002, 2006) or a 12° angle (for each side) toward the midline for the supramammillary nucleus (SUM). Stereotaxic coordinates relative to the bregma and from the skull surface were as follows: anterior VTA: anteroposterior (AP) = −5.0; mediolateral (ML) = 1.4 (left), 1.9 (right); dorsoventral (DV) = −7.2 (left), −7.3 (right); posterior VTA: AP = −6.3, ML = 1.3 (left), 1.8 (right); DV = −7.1 (left), −7.2 (right); SUM: AP = −4.3, ML = 2.2, DV = −7.2.
For the osmotic minipump implantation procedure, modified internal cannulas (31 gauge; Plastics One) were implanted bilaterally in the anterior or posterior VTA and were connected via a SILASTIC catheter to the minipumps, which were placed subcutaneously in the rat's neck.
For the SA experiments, rats were implanted with a SILASTIC catheter to the right jugular vein before the guide cannula implantation. The catheter was secured to the vein with silk sutures and passed subcutaneously to the top of the skull, where it was connected to a modified 21 gauge cannula (Plastics One) mounted to the skull with miniscrews and dental cement. Catheters were flushed with sterile saline containing gentamicin (0.08 mg/ml) every 24 h during the recovery and training phases. Rats were allowed to recover for 4–6 d after surgery, before behavioral studies were conducted. During the recovery period, rats were handled daily to reduce stress effects that might occur during the behavioral experiments.
Microdialysis
Microdialysis procedures were performed as previously described (Zangen et al., 2001). A guide cannula for microdialysis probe (CMA; Carnegie Medicine) was implanted into the aVTA of each rat using the following stereotaxic coordinates: AP = −5.2; ML = 0; DV = −5.6. A microdialysis probe (2 mm long, 20 kDa cutoff value, CMA/10; Carnegie Medicine) was inserted into a guide cannula to a final depth of 7.8 mm from the skull surface.
The microdialysis probe was inserted after the termination of the last training session conducted, 5 h before the first test session. Probes were continuously perfused with artificial CSF (aCSF) containing 125 mm NaCl, 2.5 mm KCl, 0.5 mm NaH2PO4, 5.0 mm Na2HPO4, 1.0 mm MgCl2, and 1.2 mm CaCl2, pH 7.4, while the animals were in the self-administration chambers (40 × 40 × 30 cm) with food and water available ad libitum. On the following day, the infusion flow rate was set to 1.5 μl/min. Dialysates were continuously collected in 20 min intervals and immediately frozen until assayed for DA. The microdialysis session lasted 180 min. The session began with perfusion of aCSF (80 min) followed by CNQX diluted in aCSF (60 min), and ended in a washout period with aCSF alone (40 min). Dopamine was measured with HPLC coupled to an electrochemical detector as described previously (Zangen et al., 2001).
Drugs and microinjection procedure
For the microinjection experiment, the AMPA/kainate receptor antagonist CNQX (1 nmol/0.5 μl; Sigma) was dissolved in aCSF (pH adjusted to 7.3) and microinjected into the aVTA or pVTA. Bilateral microinjections of CNQX or aCSF (0.5 μl volume per infusion) were performed over 1 min. Injectors were left in place for 30 s after completing the injection to ensure adequate diffusion from the injector tip. For the CPP experiment, morphine hydrochloride (20 mg/kg per ampoule) was administered intraperitoneally in a total volume of 0.8 ml (10 mg/kg). For the locomotor activity experiment, diacetylmorphine hydrochloride powder (heroin), provided by the National Institute on Drug Abuse, was dissolved in sterile saline and administered intraperitoneally in a total volume of 0.8 ml (0.62 mg/kg). For the SA experiments, heroin was self-administered via the intravenous catheter in a total volume of 0.13 ml/infusion (0.1 mg/kg/inf). For evaluating the ascending and descending limbs of the heroin dose–response curve, four doses of heroin were used: 0.001, 0.01, 0.05, and 0.1 mg/kg/inf. For the CNQX dose–response experiment, three of the above heroin doses (0.001, 0.05, and 0.1 mg/kg/inf) and three doses of CNQX (0.1, 0.3, and 1 nmol/0.5 μl volume per infusion) were used.
Behavioral procedures
CPP.
Place preference conditioning was performed in 72 × 24.7 × 29.5 cm plastic boxes (TSE Systems). Each box contained two distinct conditioning environments (24.7 × 30 cm) with a house light, separated by a narrow neutral zone (24.7 × 10 cm). The two conditioning environments differed in several aspects to allow rats to distinguish between the compartments. The environments differed in the presence of bedding underneath the grid floor and the color pattern of the walls. During the conditioning phase, plastic walls were inserted to restrict the rats to their designated conditioning environment. Time spent (in seconds) and total distance traveled (in meters) in each chamber was recorded using infrared beam crossings. During conditioning and testing, the room was kept in darkness while the house lights were on. In a set of previous experiments (data not shown), we found that in this condition, the rats were not biased to either of the compartments. The conditioning phase included 8 d of training in which all rats were exposed to 4 d of morphine (10 mg/kg, i.p.) and 4 d of saline (i.p.) on alternate days. Half of the rats received the morphine in one compartment, and the other half received the morphine in the other compartment. Before each intraperitoneal injection of morphine or saline, an intra-VTA microinjection of CNQX (1 nmol/0.5 μl/side) or aCSF was infused bilaterally in the anterior or posterior VTA. Each group received an intra-VTA microinjection of the designated treatment, i.e., CNQX or aCSF, on the morphine as well as on the saline days. After the injections, rats were placed in the designated compartment, according to the procedure, for 30 min. Exposure to the conditioning compartments was counterbalanced. Two days after the last conditioning day, a test day was conducted to evaluate place preference.
During the test, rats were placed in the neutral compartment with the plastic walls removed, and time spent and distance traveled in each compartment were recorded over a 15 min period using the TSE system software.
Heroin self-administration.
The SA chambers, controlled by a Med Associates computer system, contain two levers located 9 cm above the grid floor, but only one lever (an active, retractable lever) activated the infusion pump for 4 s, resulting in a 0.13 ml heroin solution infusion, and turned on a cue light stationed above the active lever for 20 s. The intravenous catheter was held to the rat's skull by dental cement and was connected to a liquid swivel (Med Associates) with polyethylene-50 tubing that was protected by a metal spring and connected to the syringe (30 ml) of the infusion pump outside of the chamber. Each press of the active lever resulted in the delivery of 0.1 (for the microinjection experiments and in the first 7 d of the microdialysis experiment) or 0.05 mg/kg/inf heroin (from day 8 of the microdialysis experiment) and the initiation of a 20 s timeout period. During this period, lever presses were recorded, but not reinforced. Presses on the other lever (an inactive, stationary lever) were also recorded. At the end of each session, the house light was turned off and the active lever was retracted.
The animals were introduced, daily for 3 h, to the SA chamber and trained on the 0.1 mg/kg/inf dose, until a SA rate stabilized above 12 infusions per session for 3 consecutive days. The dose–response effect of CNQX on the various heroin self-administration doses was evaluated in separate groups of animals for each heroin dose to avoid excess of multiple intracranial injections and tests in the same animal and potential tissue damage. Each group of animals was trained on a specific dose until stabilized for three consecutive sessions according to the following criteria: 12 infusions per session for the 0.1 mg/kg/group, 20 infusions per session for the 0.05 mg/kg group, and 10 infusions per session for the 0.001 mg/kg group. After rats achieved the criterion, tests were conducted on two (microinjection of 1 nmol CNQX experiment) or four (CNQX dose–response experiments) consecutive test days.
For the heroin dose–response experiment, rats were trained using four heroin doses (0.001, 0.01, 0.05, and 0.1 mg/kg/inf), and data were analyzed after rats reached the criterion. Note that no test sessions were conducted in this experiment.
For the 1 nmol CNQX experiment, the first test day included intra-VTA microinjections of aCSF (0.5 μl/side), and the second test day included intra-VTA microinjections of CNQX (1 nmol/0.5 μl/side) just before the session onset. For the CNQX dose–response experiments, a CNQX dose–response regimen for each of three doses of heroin (0.1, 0.05, and 0.001 mg/kg/inf) was conducted for 4 consecutive test days. The first day included intra-VTA microinjections of aCSF, followed by 3 d of CNQX injections (0.1, 0.3, and 1 nmol/0.5 μl/side). The microinjections were conducted just before the session onset. In the heroin SA studies combined with microdialysis, the first test day included aCSF infusion via the microdialysis probe for the entire session, and the second test day comprised three phases. In the first 80 min, aCSF was infused followed by 60 min of CNQX infusion (1.5 μl/min, 0.02 m) and ended with an additional 40 min washout period with aCSF infusion alone.
Locomotor activity.
Locomotor activity was measured in a 48 × 48 cm open field box (Actimot; TSE Systems) made of clear Plexiglas. The rat behavioral data were extrapolated from the interruptions of 3 × 16 infrared light barriers located in each axis (X, Y, and Z). The Actimot software was used for data acquisition and analysis. The analyzed output was an array of behavioral indices including horizontal and vertical movement. The experiments were held in total darkness. This procedure included repeated daily heroin administration (0.62 mg/kg, i.p.) combined with intra-VTA microinjections of CNQX or aCSF for 5 consecutive days. The effect of heroin on locomotor activity was measured on each of these days. Each animal was placed inside the locomotion chamber for 25 min of habituation each day, followed by an intracranial microinjection of CNQX or aCSF before intraperitoneal injection of heroin or saline, and was placed back in the chamber for another 30 min of postinjection behavioral monitoring.
In a different set of experiments, the procedure included repeated heroin administration (0.62 mg/kg, i.p.) for 7 consecutive days combined with chronic microinfusions of CNQX (1 nmol/0.25 μl/h/14 d) via preimplanted osmotic minipumps (0.25 μl/h for 14 d; Alzet; Durect), which infused the CNQX throughout the 7 d of the locomotor activity experiment. Each animal was placed inside the locomotion chamber for 25 min of habituation each day, followed by an intraperitoneal injection of heroin or saline, and was placed back in the chamber for another 60 min of postinjection behavioral monitoring.
Histology
To anatomically verify intra-VTA cannula placements, brains were cut into 40-μm-thick coronal sections, stained with cresyl violet, and examined by light microscopy. The exclusion criteria were based on the borders of the regions as determined by the rat brain atlas (Paxinos and Watson, 1998). Anterior versus posterior VTA placements were determined according to the presence (posterior) or absence (anterior) of the interpeduncular nucleus just below the VTA in the slice that included the tip of the injection cannula. Representative microphotographs are depicted in Figure 1.
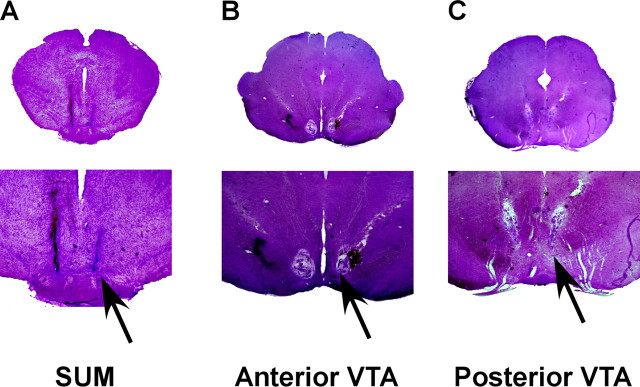
A photomicrograph of representative coronal sections stained with cresyl violet indicating the locations of microinjection sites. Each rat was implanted bilaterally with a guide cannula for microinjections aiming at the anterior VTA, posterior VTA, and SUM. A, SUM, which is defined as the region ventral to the anterior tip of the VTA. B, aVTA, which is defined as the region dorsal to the medial mammillary nucleus and medial to the substantia nigra. C, pVTA, which is defined as the region dorsal to the interpeduncular nucleus, medial to the substantia nigra, and ventral to the red nucleus. Arrows indicate the tip of the injection cannula.
Data analysis
CPP.
Place preference was analyzed using three-way ANOVA with drug (morphine vs saline), treatment (aCSF vs CNQX), and site (aVTA vs pVTA) as the main factors. As significant effects were observed, one-way ANOVA for each group (aVTA-CNQX, pVTA-CNQX, aVTA-aCSF, and pVTA-aCSF) was used with drug as the main factor. The dependent variable was the time spent in the saline- and morphine-associated compartments.
Activity data from the CPP boxes were analyzed using three-way repeated-measures ANOVA with time (4 d of morphine or saline injections) as the repeated factor and treatment (aCSF vs CNQX) and site (aVTA vs pVTA) as the between-subjects factors. Thereafter, one-way repeated ANOVA was used with time as the repeated factor to examine whether there was a gradual increase in locomotion along the conditioning days in each group. The dependent variable was the distance traveled (in meters) during the conditioning days in the saline- and morphine-associated compartments.
SA and microinjection.
For the experiment comparing the aVTA and pVTA groups, the effect of CNQX microinjection on heroin self-administration was analyzed using a three-way repeated-measures ANOVA with time (20 min blocks in the aCSF and CNQX sessions) and treatment (aCSF vs CNQX) as the repeated factors and site (aVTA vs pVTA) as the between-subjects factor. In addition, the first 20 min block was analyzed using a two-way repeated-measures ANOVA with treatment (aCSF vs CNQX) as the repeated factor and site (aVTA vs pVTA) as the between-subjects factor.
For the CNQX dose–response experiment, we analyzed the first 20 min of each session using a three-way repeated-measures ANOVA with CNQX (aCSF, 1, 0.3, and 0.1 nmol/0.5 μl/side) as the repeated factor and site (aVTA vs SUM) and heroin (0.1, 0.005, and 0.001 mg/kg/inf) as the between-subjects factors. Thereafter, to examine the effect of CNQX on each heroin dose, we conducted additional analyses using two-way repeated ANOVA for each heroin dose with CNQX as the repeated factor and site (aVTA vs SUM) as the between-subjects factor. Finally, t test was conducted to examine specific differences between the aVTA and SUM for each CNQX dose in each heroin dose.
SA and microdialysis.
The effect of continuous CNQX infusion on heroin self-administration was analyzed using a one-way repeated-measures ANOVA with treatment as the repeated factor. Three treatment phases were analyzed: 60 min before CNQX infusion during the CNQX session (preinfusion), 60 min after CNQX infusion (postinfusion), and the equivalent 60 min of the postinfusion during the aCSF session (aCSF infusion). The comparisons made were preinfusion versus postinfusion and in a different ANOVA, postinfusion versus aCSF infusion.
DA levels in the VTA.
The effect of heroin self-administration and CNQX infusion on DA levels in the VTA was analyzed using one-way ANOVA with treatment as the repeated factor. Four treatment phases were analyzed: baseline DA levels before session onset (baseline), preinfusion, postinfusion (see previous section for details), and a washout phase with aCSF (washout). The comparisons made were baseline versus preinfusion, preinfusion versus postinfusion, and postinfusion versus washout. The dependent variable was DA level in the VTA calculated as percentage from baseline level.
Locomotor activity.
The effect of repeated administration of heroin and CNQX administration on locomotor activity was analyzed using repeated-measures ANOVA, with time [day 1 vs day 5 (for the microinjection experiment) or day 1 vs day 7 (for the minipump experiment)] as the repeated factor and group (aVTA-CNQX, pVTA-CNQX, pVTA-aCSF, and saline) as the between-subjects factor. Thereafter, one-way repeated ANOVA was used with time as the repeated factor to examine whether there was a gradual increase in locomotion along the conditioning days in each group. In addition, the effect of heroin and CNQX on the first day was analyzed using one-way ANOVA with group as the main factor followed by Fisher's protected least significant difference (PLSD) post hoc analysis. The dependent variable was distance traveled (in meters) in the open field.
For all analyses conducted, data are expressed as the mean ± SEM of values obtained from the indicated number of rats. A p value of <0.05 was considered significant.
Results
Morphine-induced CPP is blocked by CNQX in the aVTA, but not the pVTA
Rats were divided into four different treatment groups: aVTA-CNQX (CNQX injected into the anterior VTA); aVTA-aCSF (aCSF injected into the anterior VTA); pVTA-CNQX (CNQX injected into the posterior VTA); and pVTA-aCSF (aCSF injected into the posterior VTA). After each daily intracranial injection, rats immediately received morphine or a saline intraperitoneal injection and were placed in the designated compartment for conditioning. After the conditioning phase (8 d), a 15 min test session was conducted in which the time spent in each compartment was recorded and analyzed.
A three-way ANOVA revealed a significant drug main effect (F(1,35) = 3.12; p < 0.05), indicating that rats spent more time in the morphine-associated compartment and hence developed a preference for the morphine-associated compartment. In addition, significant drug × treatment (F(1,35) = 5.58; p < 0.03) and drug × site × treatment (F(1,35) = 4.13; p < 0.05) interactions were observed. Thereafter, a separate one-way ANOVA on each intracranial treatment was conducted and found that only the aVTA-CNQX group did not develop a significant preference for the morphine-associated compartment (F(1,12) = 1.04; p = 0.32). This indicates that CNQX (and not aCSF) microinjections to the aVTA but not the pVTA prevented the development of preference for the morphine-associated compartment (Fig. 2A).
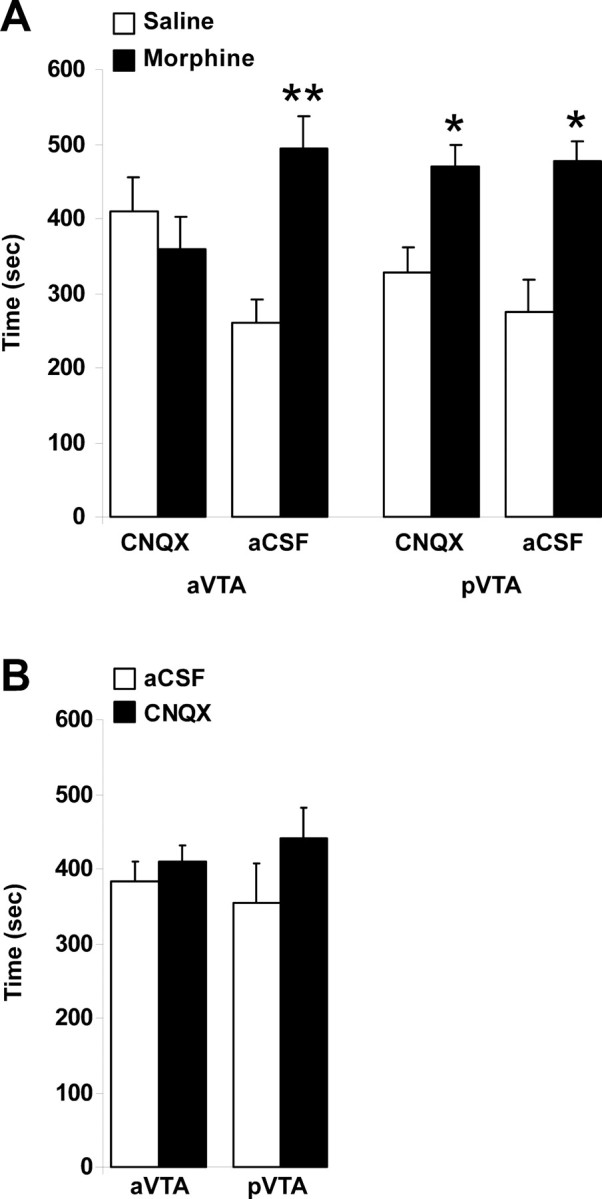
The effect of CNQX in anterior and posterior VTA on conditioned place preference induced by morphine. A, Each group of rats (aVTA-CNQX, n = 14; aVTA-aCSF, n = 10; pVTA-CNQX, n = 9; pVTA-aCSF, n = 9) received intraperitoneal injections of morphine (10 mg/kg) or saline, in two different compartments of the CPP boxes, on alternate days. CNQX (1 nmol/0.5 μl/side) or aCSF (0.5 μl/side) was microinjected bilaterally into subregions of the VTA, immediately before morphine or saline intraperitoneal injections. B, Without morphine: each group of rats (aVTA, n = 8; pVTA, n = 7) received daily, bilateral microinjections of CNQX (1 nmol/0.5 μl/side) or aCSF (0.5 μl/side) into subregions of the VTA, in two different compartments of the CPP boxes, on alternate days. Data are presented as the mean ± SEM time spent in each compartment during the 15 min test session. *p < 0.05 and **p < 0.005 compared with the saline-associated compartment.
In different groups of animals, we tested whether intra-VTA injection of CNQX alone (using the same dose of 1 nmol/0.5 μl/side) induced any place preference or aversion. Repeated-measures ANOVA revealed no significant effect of CNQX microinjections to either subregion of the VTA (F(1,13) = 0.007; p = 0.933) (Fig. 2B), indicating no rewarding or aversive effects of CNQX in the VTA.
The effect of CNQX in the aVTA and pVTA on morphine-induced locomotion
During the CPP experiments, distance traveled in each compartment was measured to examine the effect of repeated morphine administration combined with CNQX microinjections into VTA subregions on locomotion. Three-way repeated-measures ANOVA over time for the morphine conditioning sessions revealed significant time (F(3,35) = 11.73; p < 0.0001) and site (F(1,35) = 5.88; p < 0.03) main effects and a treatment × site interaction (F(1,35) = 3.89; p = 0.05), indicating a gradual increase in locomotor activity induced by repeated morphine injections (Fig. 3A,B). A separate one-way repeated-measures ANOVA over time (morphine sessions) for each group revealed that only in the pVTA-CNQX group there was no significant time main effect (F(3,6) = 0.543; p = 0.659), indicating that CNQX in the pVTA blocked the gradual increase in psychomotor response to morphine induced by repeated administration of the drug (Fig. 3B). Importantly, analyzing the distance traveled during the first morphine session using a two-way ANOVA revealed a significant site main effect (F(1,35) = 9.49; p < 0.005) and drug × site interaction (F(1,35) = 4.45; p < 0.05), indicating that the distance traveled by pVTA-CNQX rats receiving morphine for the first time was higher than that of any of the other groups of rats (Fig. 3B) and not significantly different from the distance they traveled during their first saline session (paired t test, p = 0.892).
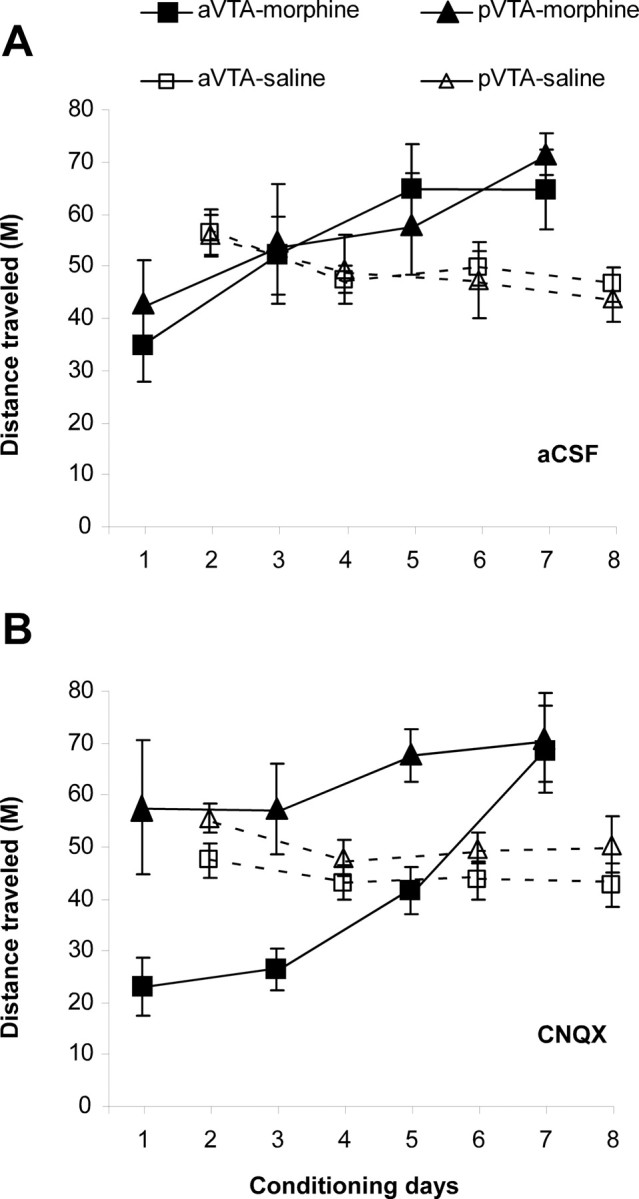
The effect of CNQX in the anterior and posterior VTA, on the distance traveled during morphine-induced place preference. A, B, aCSF (A) or CNQX (B; 1 nmol/0.5 μl/side) were microinjected daily, bilaterally into subregions of the VTA, immediately before morphine (10 mg/kg) or saline intraperitoneal injections. Morphine or saline injections were given on alternate days. Data are presented as mean ± SEM distance traveled during the conditioning sessions (30 min) in the CPP boxes.
Note that in the aCSF groups and in the group receiving CNQX to the aVTA, locomotion during the first morphine session was lower than the first saline session, possibly indicating the sedative effect of morphine and blockade of novelty-induced locomotion that is evident in the first saline session. The gradual increase in the psychomotor response to morphine probably reflects desensitization of this sedative effect and even the development of behavioral sensitization because locomotion during the last morphine session was markedly higher than the last saline session.
The effect of CNQX microinjection into the VTA on self-administration of heroin
To assess the effects of CNQX microinjections into VTA subregions on heroin intake in the SA paradigm, we trained rats with a continuous reinforcement schedule (fixed ratio 1), in which each lever press was followed by a heroin infusion. Rats rapidly learned the operant task and reliably self-administered heroin after two to three training sessions. Repeated-measures ANOVA on the eight training sessions revealed no significant difference in the number of infusions per session between aVTA and pVTA groups (F(1,13) = 1.04; p = 0.32) (Fig. 4A). After the first eight training sessions, rats underwent an aCSF session (session 9), in which they received intra-VTA microinjection of aCSF immediately before the session onset. In session 10, rats received intra-VTA microinjection of CNQX (1 nmol/0.5 μl/side) immediately before the session onset. Three-way repeated-measures ANOVA revealed significant site (F(1,13) = 8.21; p < 0.02) and time (F(1,8) = 20.81; p < 0.0001) main effects and site × time interaction (F(1,8) = 8.99; p < 0.001). This was followed by analyzing the first 20 min of the session during the “drug-loading phase” (in which microinjection has the most prominent effect) using repeated-measures ANOVA, which revealed a significant site effect (F(1,13) = 11.63; p < 0.01) (Fig. 4B) and a site × session interaction (F(1,13) = 7.8; p < 0.02) (Fig. 4B), indicating that microinjection of CNQX to the aVTA but not pVTA significantly increased the number of heroin infusions during the first 20 min of the session compared with microinjection of aCSF.
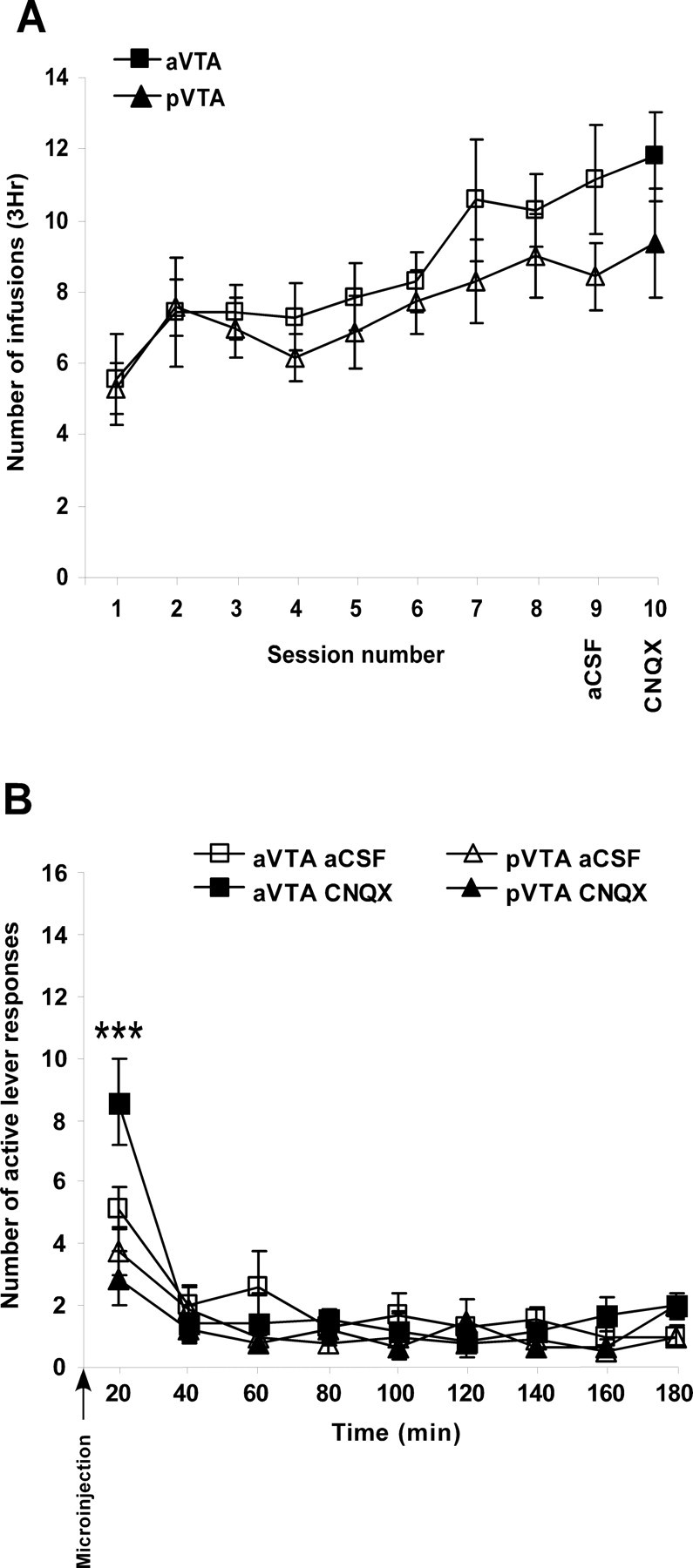
The effect of CNQX in the anterior and posterior VTA, on heroin self-administration. A, Number of heroin infusions, during 3 h self-administration sessions, into rats implanted with bilateral cannulas into either the anterior (n = 7) or posterior (n = 8) VTA. In sessions 9 (aCSF) and 10 (CNQX), rats were microinjected bilaterally with aCSF (0.5 μl/side) or CNQX (1 nmol/0.5 μl/side), respectively, immediately before the beginning of the session. B, Data are presented as the mean ± SEM number of active lever responses during 20 min periods within the aCSF and CNQX sessions. Note that during the first 20 min, only CNQX in the aVTA increased the number of active lever responses. Each symbol represents the mean ± SEM number of heroin infusions (A) during the 3 h sessions or active lever responses (B) during 20 min periods within the aCSF and CNQX sessions. ***p < 0.0001 compared with both the pVTA-CNQX and aVTA-aCSF groups.
Heroin self-administration dose–response curve
To examine whether the increase in lever presses after CNQX microinjection to the aVTA relates to a reduction in the rewarding effect of heroin, it was necessary to characterize the inverted U-shape dose–response curve of the heroin self-administration in our system. Specifically, it was important to verify that the (0.1 mg/kg) dose used in the previous experiment is on the descending limb of the inverted U-shape dose–response curve. The dose–response curve was studied in a separate group of rats using four heroin doses (Fig. 5A).
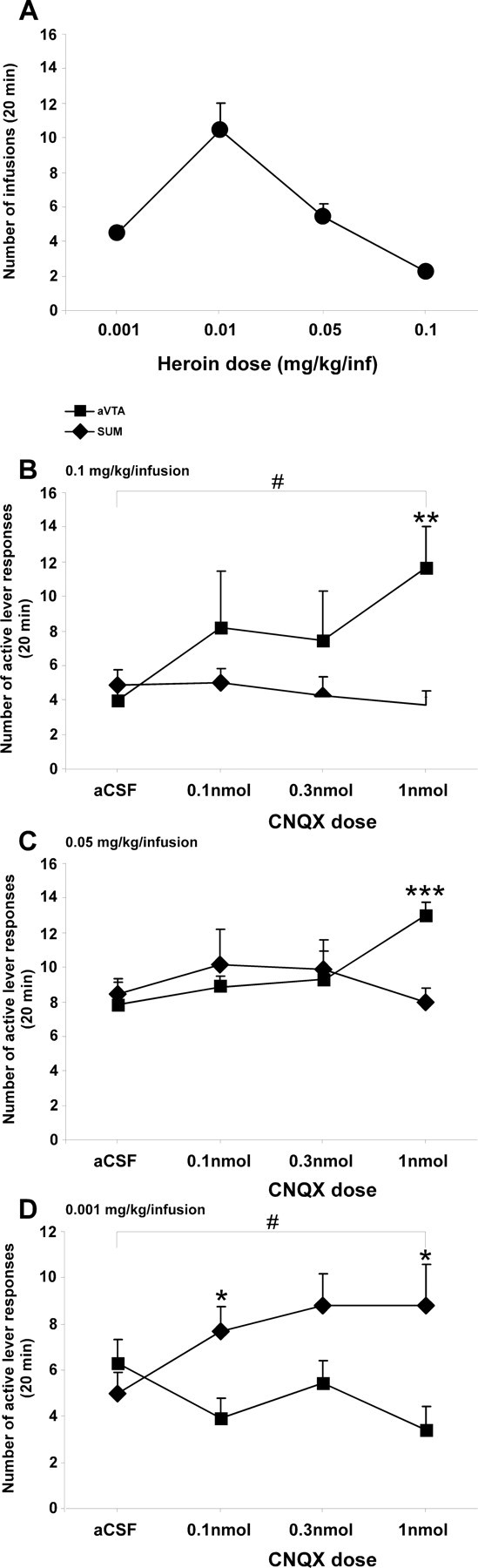
The effect of CNQX in the aVTA and SUM on heroin SA dose–response curve. A, A heroin SA dose–response curve. Data are presented as the mean ± SEM number of infusions for each heroin dose during the first 20 min of a self-administration session after rats (n = 14) met the criterion (see Materials and Methods for details). Note that no test sessions were conducted, and CNQX was not microinjected. B–D, Mean ± SEM number of active lever responses during the first 20 min of the aCSF and CNQX sessions (0.1, 0.3, 1 nmol/0.5 μl/side) microinjected to the aVTA (n = 8, 7, and 7) or SUM (n = 12, 9, and 10) in animals pressing for 0.1, 0.05, and 0.001 mg/kg/inf of heroin, respectively. #p < 0.05 for the repeated main factor of CNQX; *p < 0.05, **p < 0.005, and ***p < 0.0001 comparing aVTA to SUM.
The effect of CNQX in the aVTA and the SUM on heroin self-administration dose–response curve
In different groups of rats, we assessed the effect of several doses of CNQX microinjected into the aVTA (where a significant effect was observed in the previous experiment) and into the SUM, an adjacent brain area that is associated with glutamatergic reward function (Ikemoto et al., 2004), on the heroin self-administration dose–response curve. Three-way repeated ANOVA on the number of active lever responses during the first 20 min of the session revealed a significant CNQX-dose effect (F(3,129) = 3.91; p < 0.02) and significant CNQX × site (F(3,129) = 2.78; p < 0.05), heroin × site (F(2,43) = 3.71; p < 0.04), and CNQX × site × heroin (F(6,129) = 6.0; p < 0.0001) interactions. To examine the effect of CNQX on each heroin dose, we conducted additional analyses using two-way repeated ANOVA for each heroin dose with CNQX as the repeated factor and site (aVTA vs SUM) as the between-subjects factor. For the high heroin dose (0.1 mg/kg/inf) (Fig. 5B), we found a significant CNQX main effect (F(3,51) = 3.2; p < 0.04) and a CNQX × site interaction (F(3,51) = 5.2; p < 0.005). For the medium heroin dose (0.05 mg/kg/inf) (Fig. 5C), we found a significant CNQX × site interaction (F(3,36) = 5.24; p < 0.005). This was followed by a separate one-way repeated ANOVA for each brain site, which revealed a significant CNQX main effect only in the aVTA groups [high, n = 8; F(3,21) = 4.63; p < 0.02 (Fig. 5B); medium, n = 7; F(3,18) = 7.80; p < 0.002 (Fig. 5C)] but not in the SUM groups [high, n = 11; F(3,30) = 0.48; p = 0.7 (Fig. 5B); medium, n = 7; F(3,18) = 0.77; p = 0.52 (Fig. 5C)], indicating that for heroin doses in the descending limb of the dose–response curve, microinjection of CNQX into the aVTA but not the SUM increased the number of active lever responses, and this increase was evident in a CNQX mediated dose–response manner.
For the low heroin dose (0.001 mg/kg/inf) (Fig. 5D), there was an opposite effect of CNQX on heroin self-administration. We found a significant site main effect (F(1,14) = 5.15; p < 0.04) and a CNQX × site interaction (F(3,42) = 4.70; p < 0.01). This was followed by a separate one-way repeated ANOVA for each brain site, which revealed only a nonsignificant CNQX main effect trend in the aVTA group (n = 7; F(3,18) = 2.38; p = 0.1). However, there was a significant CNQX main effect in the SUM group (n = 9; F(3,24) = 3.60; p < 0.03). These results indicate that for a heroin dose in the ascending limb of the dose–response curve, microinjection of CNQX into the aVTA had a tendency to decrease the number of active lever responses, whereas microinjection of CNQX into the SUM increased the number of active lever responses in a dose–response manner.
The effect of continuous infusion of CNQX into the aVTA on heroin self-administration
In the previous experiment, the effect of CNQX in the aVTA on heroin SA was observed only for a short period after the microinjection of CNQX and was evident only during the first 20 min of the session. To test the hypothesis that CNQX microinjection into the aVTA blocked the rewarding effects of heroin by altering DA transmission, we conducted a microdialysis experiment in which DA was measured in the aVTA during heroin SA. This procedure also allowed us to test the effect of continuous infusion of CNQX during the SA session separately from the acute effects on the initiation of the session (the “loading phase”). Rats rapidly learned the operant task and reliably self-administered heroin after two to three training sessions (Fig. 6A). After 15 training sessions, two test sessions were conducted. Five hours before the first test session, microdialysis probes were inserted into the aVTA followed by aCSF infusion for the entire duration of the session (aCSF session) and until the following session. The next test session included 80 min of aCSF infusion via the microdialysis probes followed by 60 min of CNQX infusion and ended with an additional 40 min washout of aCSF infusion (CNQX session). One-way repeated-measures ANOVA of the total number of active lever responses during the postinfusion phase compared with the preinfusion phase and with the aCSF-infusion phase revealed a significant treatment main effect (F(1,6) = 8.27, p < 0.03 and F(1,6) = 6.05, p < 0.05, respectively). This indicates that CNQX infusion into the aVTA significantly increased the number of active lever responses compared with aCSF infusion (Fig. 6B). During the CNQX session, DA levels were measured in microdialysis samples taken from the aVTA. Repeated-measures ANOVA comparing baseline DA levels to DA levels during the first block of aCSF revealed a significant main effect (F(1,6) = 25.04; p < 0.005), indicating that heroin intake induced a significant increase in extracellular DA levels. This increase reverted back to baseline levels by CNQX infusion into the aVTA (F(1,6) = 24.38; p < 0.005) (Fig. 6C). Importantly, the CNQX-mediated DA decrease was accompanied by a large increase in the number of active lever responses (Fig. 6B). After a washout of CNQX with aCSF, DA levels returned to pre-CNQX levels (F(1,6) = 21.14; p < 0.005) (Fig. 6C) accompanied by a decrease in the number of active lever responses (Fig. 6B).
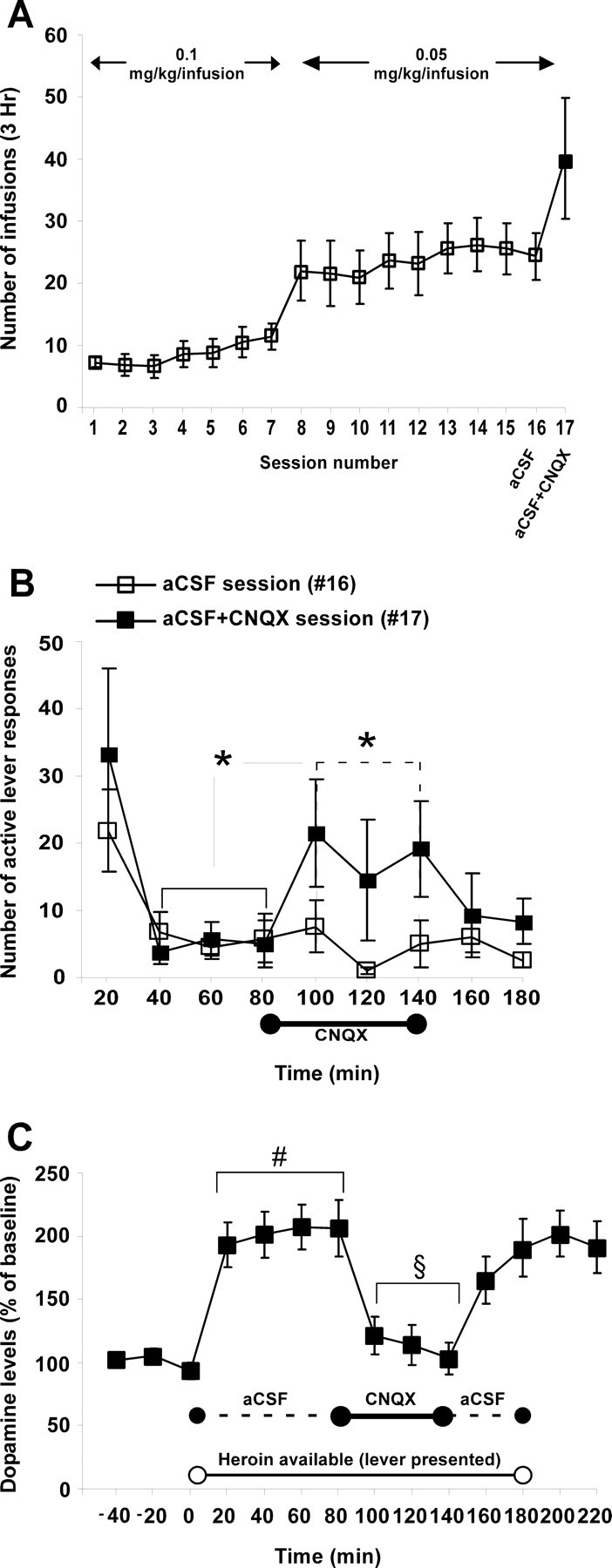
The effect of CNQX infusion into the aVTA on local dopamine levels and heroin self-administration. A, Data are presented as the mean ± SEM number of heroin infusions during 3 h self-administration sessions (both in training and tests) of rats implanted with guide cannulas for a microdialysis probe directed to the aVTA (n = 9). Before session 16 (aCSF), aCSF was constantly infused during the entire 3 h session. In session 17 (aCSF + CNQX), aCSF and then CNQX (1.5 μl/min, 0.02 m) were infused via the microdialysis probe into the aVTA. B, Mean ± SEM number of active lever responses during 20 min periods of the aCSF and aCSF + CNQX sessions. C, Mean ± SEM extracellular levels of dopamine measured from the aVTA during the aCSF + CNQX session. Data represent the changes in extracellular DA levels compared with baseline levels obtained before session onset. The line near the time axis (labeled “Heroin available”) with the empty circles represents the time period when the active lever was available. The dashed line represents aCSF infusion, and the continuous line represents CNQX infusion time period. *p < 0.05, significant difference between the 20 min time periods before CNQX infusion and the 20 min time periods during CNQX infusion in the aCSF + CNQX session (solid line; B) and a significant difference between aCSF + CNQX session to the aCSF session throughout the 20 min time periods while CNQX was infused (dashed line; B). #p < 0.05 compared with baseline fractions. §p < 0.05 compared with aCSF fractions within the aCSF + CNQX session.
The effect of CNQX in the VTA on the psychomotor effects of heroin
To test whether the results observed during intermittent morphine administration in the small CPP boxes were replicable, we administered heroin to rats daily in standard locomotion boxes. We did not expect aCSF to have different effects when injected into the anterior or posterior VTA, and in the CPP experiments we did not find any differential effect of aCSF injected into the anterior or posterior VTA. Therefore, the following experiments on the effect of opioids and CNQX on locomotion included only aCSF injection into the pVTA control group. In this paradigm, we examined the effect of daily (via bilateral microinjections, for 5 consecutive days) and chronic (via bilateral preimplanted osmotic minipumps, for 7 consecutive days) CNQX administration on locomotion in rats receiving daily injections of heroin intraperitoneally. Repeated-measures ANOVA revealed a significant group main effect in the microinjection experiment (F(3,31) = 13; p < 0.0001) and a significant group × time interaction in the microinjection and minipump experiments [F(3,31) = 7.830; p < 0.005; microinjection (Fig. 7A); F(3,31) = 7; p < 0.005; minipump (Fig. 7B)]. Further analysis comparing the first and last session in each group separately revealed that whereas repeated administration of heroin induced an increase in the psychomotor response to the drug in the aVTA-CNQX groups [F(1,5) = 21.006; p < 0.01; microinjection (Fig. 7A); F(1,6) = 10.68; p < 0.05; minipump (Fig. 7B)] and in the pVTA-aCSF groups [F(1,6) = 12.63; p < 0.05; microinjection (Fig. 7A); F(1,10) = 6.81; p < 0.05; minipump (Fig. 7B)], such effect was absent in the pVTA-CNQX groups [F(1,6) = 0.15; p = 0.71; microinjection (Fig. 7A); F(1,8) = 0.97; p = 0.35; minipump (Fig. 7B)]. Furthermore, in the saline groups there was a significant reduction in the distance traveled when comparing the first and last session [F(1,6) = 96.78; p < 0.0001; microinjection (Fig. 7A); F(7,1) = 5.39; p = 0.053; minipump (Fig. 7B)], indicating a habituation effect caused by repeated saline administration. Importantly, analyzing the effect of heroin and CNQX on locomotion in the first day using one-way ANOVA revealed a significant group effect in the microinjection experiment and a nearly significant effect in the minipump experiment [F(3,23) = 9.53; p < 0.0005; microinjection (Fig. 7A); F(3,31) = 2.31; p = 0.09; minipump (Fig. 7B)]. This was followed by Fisher's PLSD post hoc analyses revealing that aVTA-CNQX group had lower locomotor activity compared with saline-treated rats (p < 0.05, microinjection; p < 0.05, minipump) and to pVTA-CNQX group (p < 0.0005, microinjection; p = 0.05, minipump) and that pVTA-CNQX group had higher locomotor activity (p < 0.05; microinjection), which was not different from the saline-treated rats (p = 0.77; minipump).
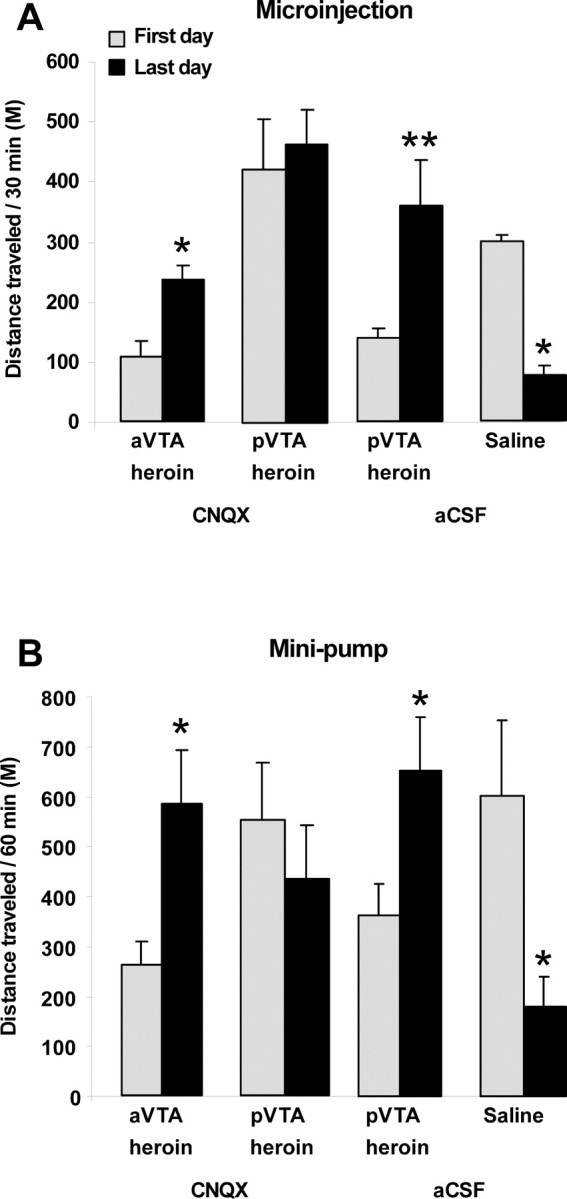
The effect of CNQX in the anterior and posterior VTA on heroin-induced locomotion. A, Each group of rats (aVTA-CNQX, n = 6; pVTA-CNQX, n = 7; pVTA-aCSF, n = 7; saline-aCSF, n = 7) received repeated bilateral microinjections of CNQX (1 nmol/0.5 μl/side) or aCSF to subregions of the VTA, immediately before each daily intraperitoneal injection of heroin (0.62 mg/kg) or saline for 5 consecutive days. B, Each group of rats (aVTA-CNQX, n = 6; pVTA-CNQX, n = 10; pVTA-aCSF, n = 11; saline-aCSF, n = 8) was chronically infused with CNQX (1 nmol/0.25 μl/h/10 d) or aCSF to subregions of the VTA through bilateral preimplanted minipumps. Three days after surgery (during which time CNQX or aCSF was continuously delivered via the minipumps), rats began to receive daily intraperitoneal injections of heroin or saline for 7 consecutive days, and locomotion was recorded. Mean ± SEM values of the distance traveled during 30 min (A) or 60 min (B) sessions are presented for the first and last heroin or saline sessions. *p < 0.05 and **p < 0.005 compared with distance traveled during the first day of heroin or saline intraperitoneal injection.
Discussion
The CPP experiments together with the SA and the locomotion measurements reveal that glutamate transmission in the aVTA is critical for opiate reward, without affecting the psychomotor alterations induced by opiates. On the other hand, glutamate transmission in the pVTA was found to influence strongly the psychomotor effects of opiates, without affecting their rewarding properties.
We demonstrated that CNQX administration into the aVTA, but not the pVTA, blocked morphine's ability to induce a place preference. However, the CPP paradigm might not reflect the rewarding effect directly and model only the cue-elicited conditioning that motivates drug-taking behavior, whereas the SA paradigm more accurately represents drug-taking behavior per se (Sanchis-Segura and Spanagel, 2006). Therefore we used a heroin SA paradigm to further clarify the role of glutamate transmission within the VTA in opiate reward. In the SA paradigm, after some training sessions the rate of infusions reaches a plateau, and the behavior stabilizes and appears to become regulated by the systemic drug level. Rats respond rapidly for the first few minutes of a session (the “loading” phase) and thereafter settle into a pattern of spaced responding (the “maintenance” phase) that is predictable based on the pharmacokinetics of the rewarding drug (Dougherty and Pickens, 1973) and the DA level within the mesolimbic system (Ranaldi et al., 1999). In the first SA experiment, in which we used the high heroin dose, we showed that administration of CNQX into the aVTA (but not the pVTA) before the SA session onset increases the number of heroin SA responses during the “loading” phase of the session. To verify that CNQX within the aVTA can increase lever responses also during the maintenance phase of the SA session, we examined the effect of continuous CNQX infusion via a microdialysis probe and found a similar increase in lever pressing for heroin reward.
Well-trained rats compensate for a decrease in a drug dose by an increase in the response rate, such that a relatively constant drug level is maintained (Pickens and Thompson, 1968). Similarly, reduction in the rewarding effect of the drug would initially cause an increase in the response rate. This is true when testing SA using doses within the “descending limb” of the inverted-U-shape dose–response curve. On the other hand, when the SA dose is very low (on the “ascending limb”), the reinforcing effect of the drug is suboptimal, and then increased lever presses could indicate an increased reinforcing effect of the drug (Tsibulsky and Norman, 1999). To clarify the interpretation of the increase in lever pressing induced by CNQX in the present study, we constructed a heroin SA dose–response curve and determined that the dose used was within the “descending limb” of the curve (Fig. 5A), and further tested the effect of CNQX in animals self-administrating other heroin doses. Indeed, CNQX in the aVTA increased responding rate on the descending limb experiments and tended to decrease the responding rate in the ascending limb experiments. Therefore, CNQX in the aVTA reduces the rewarding effect of heroin, and the effect found in the CPP experiment cannot be attributed merely to interference with contextual learning.
Additional evidence for this notion comes from analyzing the microdialysis samples during the maintenance phase, which revealed that CNQX reduced somatodendritic DA levels and was accompanied by increased active lever responses. Elevated DA transmission in the VTA can be achieved either by indirect inhibition of GABA transmission or by directly enhancing glutamate transmission within the VTA (Vanderschuren and Kalivas, 2000). Heroin administration results in burst firing of DA neurons within the VTA, thought to mediate its rewarding effect (Yokel and Wise, 1975). In addition, somatodendritic DA levels are elevated by opiate administration and during increases in the firing rate of VTA DA neurons (Cheramy et al., 1981; Klitenick et al., 1992; Wang et al., 1994; Iravani et al., 1996). Hence, we hypothesize that the decrease in somatodendritic DA levels observed after CNQX administration reflects a reduction in the firing rate of DA neurons, which results in a decreased rewarding effect of heroin. Overall, the present study indicates that opiate rewarding properties are strongly affected by glutamate transmission in the aVTA, but not pVTA, and are consistent with the hypothesis that endogenous glutamatergic transmission within the VTA plays an important role in mediating opiate reinforcement (Carlezon et al., 2000; Xi and Stein, 2002). The present finding seems somewhat contradictory to our previous findings on rewarding effects of opiates injected to the pVTA, but not the aVTA (Zangen et al., 2002), and an aversive effect of AMPA itself when injected into the aVTA (Ikemoto et al., 2004). Nevertheless, here we found that blockade of AMPA receptors by CNQX in the aVTA does not produce place preference or aversion (Fig. 2b), indicating that tonic activity of AMPA receptors per se is not aversive. Therefore, a potential explanation for the different observations is that the reward signal of opiates originates at the pVTA by disinhibition of GABAergic interneurons (Johnson and North, 1992) that enhance DA activity in the aVTA. Such enhanced DA activity (or at least the rewarding effect) is dependent on tonic activity of glutamate in the aVTA, whereas overactivation of AMPA in the aVTA (without the presence of opiates) is aversive.
Although CNQX affected heroin SA in a dose–response manner (Fig. 5), it is difficult to conclude that the effect of CNQX on opiate reward is mediated merely by blockade of AMPA/kainate receptors. High doses of CNQX can act as a noncompetitive antagonist of the NMDA receptor via the glycine site (Kessler et al., 1989). Indeed, the highest CNQX dose used in this study produced the greatest effects on heroin SA. Therefore, we cannot rule out the possibility that the CNQX effects were mediated by blocking NMDA receptor function.
The effects of CNQX microinjected to the aVTA could be attributed to diffusion of the compound to the adjacent SUM region, where AMPA can produce a robust rewarding effect, which is blocked by CNQX (Ikemoto et al., 2004). However, the present study shows that CNQX in the SUM has no effect on heroin SA when standard heroin doses (within the descending limb of the heroin SA dose–response curve) are used. Nevertheless, CNQX significantly increased lever responses, in a dose–response manner, when using the low heroin dose (within the ascending limb of the curve). Given the lack of effect of CNQX in the SUM on SA of higher heroin doses and the notion that AMPA is actually rewarding in the SUM (Ikemoto et al., 2004), it is unlikely that CNQX in the SUM enhances the rewarding effect of heroin. A more plausible explanation is that CNQX in the SUM has an aversive effect, and that heroin can compensate for such aversion. Therefore, CNQX microinjection into the SUM might serve as a negative reinforcer. On the other hand, high doses of heroin immediately produce a euphoric effect that masks the aversive effect of CNQX in the SUM and therefore make it negligible. Hence, we hypothesize that the effect of CNQX in the aVTA is directly linked to neurochemical mechanisms of reward induced by opiates (reducing opiate reward), whereas CNQX in the SUM has an independent effect and does not interfere with heroin reward mechanisms, therefore not always altering the behavioral response induced by heroin.
Repeated opiate administration results in sensitization to their psychomotor effects (Kalivas and Stewart, 1991; Kuribara, 1996), which is partly mediated by drug-induced neuroadaptive changes in a circuit involving dopaminergic and glutamatergic neurotransmission including the VTA (Vanderschuren and Kalivas, 2000). Moreover, repeated opiate administration enhances motor activity by stimulating μ-opioid receptors, which, in the VTA, enhances mesolimbic DA neurotransmission and increases both somatodendritic and axonal DA release (Kalivas and Duffy, 1990; Klitenick et al., 1992). Indeed, analyzing the locomotion data from the CPP boxes revealed a gradual increase in responsiveness to repeated intermittent administration of morphine in the aCSF groups and in the group receiving CNQX to the aVTA. However, the reduced locomotion on the first day and the following gradual increase in the locomotor response to morphine was not evident in the group receiving CNQX into the pVTA. In fact, CNQX into the pVTA blocked morphine-induced reduction in locomotion during the first session and even produced a remarkable increase in locomotion. However, no additional increase in psychomotor activation was observed in the CNQX–pVTA group during the following morphine sessions. Similar results were obtained with heroin, in the standard locomotion boxes (Fig. 7), where we used daily rather than intermittent drug injections. In these experiments, we have verified that administration of CNQX (microinjected or continuously infused) into the pVTA influenced locomotor activity by blocking the sedative effect of heroin leading to heightened (and maximal) response already on the first session, whereas CNQX into the aVTA did not significantly influence the psychomotor effects of opiates.
The VTA has an important role in establishing both rewarding and stimulant properties of opiates (Kalivas, 1993; Wise, 1998). Anatomic differences within the VTA, however, indicate a functional heterogeneity. For example, overexpression of the AMPA receptor subunit GluR1 (Carlezon et al., 2000), phospholipase Cγ1 (Bolaños et al., 2003), or CREB (cAMP response element-binding protein) (Olson et al., 2005) in the aVTA potentiates systemic morphine reinforcement, whereas overexpression of these agents in the pVTA makes morphine aversive. It is possible that different populations of DA and GABA neurons, which are the predominant neuron types within the VTA (Carr and Sesack, 2000), mediate the divergent behavioral effects observed in the anterior and posterior portions, and are affected differently by CNQX administration. Recent studies revealed that the aVTA contains more glutamatergic (Yamaguchi et al., 2007) and GABAergic neurons (Olson and Nestler, 2007), whereas the pVTA has a majority population of dopaminergic neurons (Ikemoto, 2007). Thus, the behavioral effects observed might reflect the predominant population in each subregion that is affected by the CNQX-induced blockade of excitation.
Another possible explanation for the differential anterior and posterior effects is that each of these subregions contains different dopaminergic projections to distinct regions of the mesocorticolimbic system. This was demonstrated in a comprehensive and elaborative study (Ikemoto, 2007) showing that the dopaminergic neurons from the pVTA project mainly into the medial shell of the NAC, whereas those of the aVTA are directed mainly into its lateral shell and core. It appears that the medial shell does not play a major role in opiate reward, because lesions in this area did not affect morphine CPP (Sellings and Clarke, 2003). This is consistent with our findings regarding the lack of effect of CNQX administered to the pVTA on opiate CPP and self-administration.
A previous study showed that morphine-pretreated rats responded to a subsequent morphine challenge with hyperlocomotion, whereas saline-pretreated rats responded to the first morphine challenge with hypolocomotion. Interestingly, the hyperlocomotion was accompanied by increased DA levels in the NAC core but decreased DA levels in the NAC shell, whereas the hypolocomotion was accompanied by opposite effects on DA within the NAC (Cadoni and Di Chiara, 1999). It is reasonable to assume that CNQX in the pVTA reduced the glutamatergic tone and excitation drive of dopaminergic neurons in this region, resulting in reduced dopaminergic secretion in the target areas of the pVTA, including the NAC medial shell (Ikemoto, 2007). Therefore, in accordance with the work by Cadoni and Di Chiara (1999), reduced DA levels in the medial NAC shell induced by CNQX injection to the pVTA were accompanied by hyperlocomotion already after the first exposure to opiates.
Our results provide further support for the different roles of the anterior and posterior portions of the VTA in reward function and for a specific role of glutamatergic transmission in the aVTA in opiate reward. Furthermore, we demonstrate dissociation between the rewarding effect and the psychomotor responses induced by opiates, and that each of these processes is differentially affected by glutamatergic transmission in the anterior and posterior portions of the VTA.
Footnotes
This work was supported by the Rosenzweig-Coopersmith Foundation and by the Joseph and Celia Reskin fund.
References
- Bolaños CA, Perrotti LI, Edwards S, Eisch AJ, Barrot M, Olson VG, Russell DS, Neve RL, Nestler EJ. Phospholipase Cγ in distinct regions of the ventral tegmental area differentially modulates mood-related behaviors. J Neurosci. 2003;23:7569–7576. [Europe PMC free article] [Abstract] [Google Scholar]
- Cadoni C, Di Chiara G. Reciprocal changes in dopamine responsiveness in the nucleus accumbens shell and core and in the dorsal caudate-putamen in rats sensitized to morphine. Neuroscience. 1999;90:447–455. [Abstract] [Google Scholar]
- Carlezon WA, Jr, Haile CN, Coppersmith R, Hayashi Y, Malinow R, Neve RL, Nestler EJ. Distinct sites of opiate reward and aversion within the midbrain identified using a herpes simplex virus vector expressing GluR1. J Neurosci. 2000;20(RC62):1–5. [Abstract] [Google Scholar]
- Carr DB, Sesack SR. Projections from the rat prefrontal cortex to the ventral tegmental area: target specificity in the synaptic associations with mesoaccumbens and mesocortical neurons. J Neurosci. 2000;20:3864–3873. [Abstract] [Google Scholar]
- Charara A, Smith Y, Parent A. Glutamatergic inputs from the pedunculopontine nucleus to midbrain dopaminergic neurons in primates: Phaseolus vulgaris-leucoagglutinin anterograde labeling combined with postembedding glutamate and GABA immunohistochemistry. J Comp Neurol. 1996;364:254–266. [Abstract] [Google Scholar]
- Cheramy A, Leviel V, Glowinski J. Dendritic release of dopamine in the substantia nigra. Nature. 1981;289:537–542. [Abstract] [Google Scholar]
- Dougherty J, Pickens R. Fixed-interval schedules of intravenous cocaine presentation in rats. J Exp Anal Behav. 1973;20:111–118. [Europe PMC free article] [Abstract] [Google Scholar]
- Fitzgerald LW, Ortiz J, Hamedani AG, Nestler EJ. Drugs of abuse and stress increase the expression of GluR1 and NMDAR1 glutamate receptor subunits in the rat ventral tegmental area: common adaptations among cross-sensitizing agents. J Neurosci. 1996;16:274–282. [Europe PMC free article] [Abstract] [Google Scholar]
- Gariano RF, Groves PM. Burst firing induced in midbrain dopamine neurons by stimulation of the medial prefrontal and anterior cingulate cortices. Brain Res. 1988;462:194–198. [Abstract] [Google Scholar]
- Harris GC, Wimmer M, Byrne R, Aston-Jones G. Glutamate-associated plasticity in the ventral tegmental area is necessary for conditioning environmental stimuli with morphine. Neuroscience. 2004;129:841–847. [Abstract] [Google Scholar]
- Hyman SE, Malenka RC. Addiction and the brain: the neurobiology of compulsion and its persistence. Nat Rev Neurosci. 2001;2:695–703. [Abstract] [Google Scholar]
- Ikemoto S. Dopamine reward circuitry: two projection systems from the ventral midbrain to the nucleus accumbens-olfactory tubercle complex. Brain Res Rev. 2007;56:27–78. [Europe PMC free article] [Abstract] [Google Scholar]
- Ikemoto S, Wise RA. Rewarding effects of the cholinergic agents carbachol and neostigmine in the posterior ventral tegmental area. J Neurosci. 2002;22:9895–9904. [Abstract] [Google Scholar]
- Ikemoto S, Kohl RR, McBride WJ. GABA(A) receptor blockade in the anterior ventral tegmental area increases extracellular levels of dopamine in the nucleus accumbens of rats. J Neurochem. 1997;69:137–143. [Abstract] [Google Scholar]
- Ikemoto S, Murphy JM, McBride WJ. Regional differences within the rat ventral tegmental area for muscimol self-infusions. Pharmacol Biochem Behav. 1998;61:87–92. [Abstract] [Google Scholar]
- Ikemoto S, Witkin BM, Zangen A, Wise RA. Rewarding effects of AMPA administration into the supramammillary or posterior hypothalamic nuclei but not the ventral tegmental area. J Neurosci. 2004;24:5758–5765. [Europe PMC free article] [Abstract] [Google Scholar]
- Iravani MM, Muscat R, Kruk ZL. Comparison of somatodendritic and axon terminal dopamine release in the ventral tegmental area and the nucleus accumbens. Neuroscience. 1996;70:1025–1037. [Abstract] [Google Scholar]
- Johnson SW, North RA. Opioids excite dopamine neurons by hyperpolarization of local interneurons. J Neurosci. 1992;12:483–488. [Europe PMC free article] [Abstract] [Google Scholar]
- Kalivas PW. Neurotransmitter regulation of dopamine neurons in the ventral tegmental area. Brain Res Brain Res Rev. 1993;18:75–113. [Abstract] [Google Scholar]
- Kalivas PW, Duffy P. Effect of acute and daily cocaine treatment on extracellular dopamine in the nucleus accumbens. Synapse. 1990;5:48–58. [Abstract] [Google Scholar]
- Kalivas PW, Stewart J. Dopamine transmission in the initiation and expression of drug- and stress-induced sensitization of motor activity. Brain Res Brain Res Rev. 1991;16:223–244. [Abstract] [Google Scholar]
- Kessler M, Baudry M, Lynch G. Quinoxaline derivatives are high-affinity antagonists of the NMDA receptor-associated glycine sites. Brain Res. 1989;489:377–382. [Abstract] [Google Scholar]
- Klitenick MA, DeWitte P, Kalivas PW. Regulation of somatodendritic dopamine release in the ventral tegmental area by opioids and GABA: an in vivo microdialysis study. J Neurosci. 1992;12:2623–2632. [Europe PMC free article] [Abstract] [Google Scholar]
- Kuribara H. Effects of interdose interval on ambulatory sensitization to methamphetamine, cocaine and morphine in mice. Eur J Pharmacol. 1996;316:1–5. [Abstract] [Google Scholar]
- Mansvelder HD, McGehee DS. Long-term potentiation of excitatory inputs to brain reward areas by nicotine. Neuron. 2000;27:349–357. [Abstract] [Google Scholar]
- Olson VG, Nestler EJ. Topographical organization of GABAergic neurons within the ventral tegmental area of the rat. Synapse. 2007;61:87–95. [Abstract] [Google Scholar]
- Olson VG, Zabetian CP, Bolanos CA, Edwards S, Barrot M, Eisch AJ, Hughes T, Self DW, Neve RL, Nestler EJ. Regulation of drug reward by cAMP response element-binding protein: evidence for two functionally distinct subregions of the ventral tegmental area. J Neurosci. 2005;25:5553–5562. [Europe PMC free article] [Abstract] [Google Scholar]
- Paxinos G, Watson C. Ed 4. San Diego: Academic; 1998. The rat brain in stereotaxic coordinates. [Google Scholar]
- Pickens R, Thompson T. Cocaine-reinforced behavior in rats: effects of reinforcement magnitude and fixed-ratio size. J Pharmacol Exp Ther. 1968;161:122–129. [Abstract] [Google Scholar]
- Ranaldi R, Pocock D, Zereik R, Wise RA. Dopamine fluctuations in the nucleus accumbens during maintenance, extinction, and reinstatement of intravenous d-amphetamine self-administration. J Neurosci. 1999;19:4102–4109. [Abstract] [Google Scholar]
- Saal D, Dong Y, Bonci A, Malenka RC. Drugs of abuse and stress trigger a common synaptic adaptation in dopamine neurons. Neuron. 2003;37:577–582. [Abstract] [Google Scholar]
- Sanchis-Segura C, Spanagel R. Behavioural assessment of drug reinforcement and addictive features in rodents: an overview. Addict Biol. 2006;11:2–38. [Abstract] [Google Scholar]
- Schultz W. Getting formal with dopamine and reward. Neuron. 2002;36:241–263. [Abstract] [Google Scholar]
- Sellings LH, Clarke PB. Segregation of amphetamine reward and locomotor stimulation between nucleus accumbens medial shell and core. J Neurosci. 2003;23:6295–6303. [Europe PMC free article] [Abstract] [Google Scholar]
- Tsibulsky VL, Norman AB. Satiety threshold: a quantitative model of maintained cocaine self-administration. Brain Res. 1999;839:85–93. [Abstract] [Google Scholar]
- Tzschentke TM, Schmidt WJ. Glutamatergic mechanisms in addiction. Mol Psychiatry. 2003;8:373–382. [Abstract] [Google Scholar]
- Ungless MA, Whistler JL, Malenka RC, Bonci A. Single cocaine exposure in vivo induces long-term potentiation in dopamine neurons. Nature. 2001;411:583–587. [Abstract] [Google Scholar]
- Vanderschuren LJ, Kalivas PW. Alterations in dopaminergic and glutamatergic transmission in the induction and expression of behavioral sensitization: a critical review of preclinical studies. Psychopharmacology. 2000;151:99–120. [Abstract] [Google Scholar]
- Wang T, O'Connor WT, Ungerstedt U, French ED. N-methyl-d-aspartic acid biphasically regulates the biochemical and electrophysiological response of A10 dopamine neurons in the ventral tegmental area: in vivo microdialysis and in vitro electrophysiological studies. Brain Res. 1994;666:255–262. [Abstract] [Google Scholar]
- Wise RA. Drug-activation of brain reward pathways. Drug Alcohol Depend. 1998;51:13–22. [Abstract] [Google Scholar]
- Wise RA, Bozarth MA. A psychomotor stimulant theory of addiction. Psychol Rev. 1987;94:469–492. [Abstract] [Google Scholar]
- Wolf ME, Sun X, Mangiavacchi S, Chao SZ. Psychomotor stimulants and neuronal plasticity. Neuropharmacology. 2004;47(Suppl 1):61–79. [Abstract] [Google Scholar]
- Xi ZX, Stein EA. Blockade of ionotropic glutamatergic transmission in the ventral tegmental area reduces heroin reinforcement in rat. Psychopharmacology. 2002;164:144–150. [Abstract] [Google Scholar]
- Yamaguchi T, Sheen W, Morales M. Glutamatergic neurons are present in the rat ventral tegmental area. Eur J Neurosci. 2007;25:106–118. [Europe PMC free article] [Abstract] [Google Scholar]
- Yokel RA, Wise RA. Increased lever pressing for amphetamine after pimozide in rats: implications for a dopamine theory of reward. Science. 1975;187:547–549. [Abstract] [Google Scholar]
- Zangen A, Nakash R, Overstreet DH, Yadid G. Association between depressive behavior and absence of serotonin-dopamine interaction in the nucleus accumbens. Psychopharmacology. 2001;155:434–439. [Abstract] [Google Scholar]
- Zangen A, Ikemoto S, Zadina JE, Wise RA. Rewarding and psychomotor stimulant effects of endomorphin-1: anteroposterior differences within the ventral tegmental area and lack of effect in nucleus accumbens. J Neurosci. 2002;22:7225–7233. [Europe PMC free article] [Abstract] [Google Scholar]
- Zangen A, Solinas M, Ikemoto S, Goldberg SR, Wise RA. Two brain sites for cannabinoid reward. J Neurosci. 2006;26:4901–4907. [Europe PMC free article] [Abstract] [Google Scholar]
Articles from The Journal of Neuroscience are provided here courtesy of Society for Neuroscience
Full text links
Read article at publisher's site: https://doi.org/10.1523/jneurosci.1958-08.2008
Read article for free, from open access legal sources, via Unpaywall:
https://www.jneurosci.org/content/jneuro/28/34/8406.full.pdf
Free to read at www.jneurosci.org
http://www.jneurosci.org/cgi/content/abstract/28/34/8406
Free after 6 months at www.jneurosci.org
http://www.jneurosci.org/cgi/content/full/28/34/8406
Free after 6 months at www.jneurosci.org
http://www.jneurosci.org/cgi/reprint/28/34/8406.pdf
Citations & impact
Impact metrics
Citations of article over time
Article citations
Ventral tegmental area glutamate neurons establish a mu-opioid receptor gated circuit to mesolimbic dopamine neurons and regulate opioid-seeking behavior.
Neuropsychopharmacology, 48(13):1889-1900, 05 Jul 2023
Cited by: 12 articles | PMID: 37407648
Heroin and its metabolites: relevance to heroin use disorder.
Transl Psychiatry, 13(1):120, 08 Apr 2023
Cited by: 6 articles | PMID: 37031205 | PMCID: PMC10082801
Review Free full text in Europe PMC
Impact of high-access exercise prior to and during early adolescence on later vulnerability to opioid use and relapse in male rats.
Transl Psychiatry, 12(1):425, 03 Oct 2022
Cited by: 1 article | PMID: 36192388 | PMCID: PMC9529880
Glutamatergic Systems and Memory Mechanisms Underlying Opioid Addiction.
Cold Spring Harb Perspect Med, 11(3):a039602, 01 Mar 2021
Cited by: 13 articles | PMID: 32341068 | PMCID: PMC7718856
Review Free full text in Europe PMC
Rewarding effects of M4 but not M3 muscarinic cholinergic receptor antagonism in the rostromedial tegmental nucleus.
Behav Brain Res, 379:112340, 04 Nov 2019
Cited by: 1 article | PMID: 31697984 | PMCID: PMC6917983
Go to all (44) article citations
Similar Articles
To arrive at the top five similar articles we use a word-weighted algorithm to compare words from the Title and Abstract of each citation.
Blockade of ionotropic glutamatergic transmission in the ventral tegmental area reduces heroin reinforcement in rat.
Psychopharmacology (Berl), 164(2):144-150, 05 Sep 2002
Cited by: 45 articles | PMID: 12404076
Persistent Adaptations in Afferents to Ventral Tegmental Dopamine Neurons after Opiate Withdrawal.
J Neurosci, 35(28):10290-10303, 01 Jul 2015
Cited by: 48 articles | PMID: 26180204 | PMCID: PMC4502267
Glutamate-associated plasticity in the ventral tegmental area is necessary for conditioning environmental stimuli with morphine.
Neuroscience, 129(3):841-847, 01 Jan 2004
Cited by: 101 articles | PMID: 15541905
Localization of brain reinforcement mechanisms: intracranial self-administration and intracranial place-conditioning studies.
Behav Brain Res, 101(2):129-152, 01 Jun 1999
Cited by: 368 articles | PMID: 10372570
Review