Abstract
Free full text

Chlamydia and Its Many Ways of Escaping the Host Immune System
Abstract
The increasing number of new cases of Chlamydia infection worldwide may be attributed to the pathogen's ability to evade various host immune responses. Summarized here are means of evasion utilized by Chlamydia enabling survival in a hostile host environment. The pathogen's persistence involves a myriad of molecular interactions manifested in a variety of ways, e.g., formation of membranous intracytoplasmic inclusions and cytokine-induced amino acid synthesis, paralysis of phagocytic neutrophils, evasion of phagocytosis, inhibition of host cell apoptosis, suppression of antigen presentation, and induced expression of a check point inhibitor of programmed host cell death. Future studies could focus on the targeting of these molecules associated with immune evasion, thus limiting the spread and tissue damage caused by this pathogen.
1. Introduction
Chlamydiae are obligate, intracellular bacteria that target epithelial cells at different mucosal sites and give rise to a wide range of clinical presentations [1]. The most common species of the genus Chlamydia that colonizes the human host causing disease is Chlamydia trachomatis [2]. Depending on the bacterial outer membrane genotype, C. trachomatis isolated from patients is categorized into several different serovars that have been shown to target distinct tissues giving rise to different clinical presentations. Serovar types A, B, and C infect conjunctiva epithelial cells, and if left untreated can cause Trachomatous Trichiasis which can lead to irreversible corneal scarring and blindness [3]. Genital chlamydial infection is caused by C. trachomatis serovars D through K. Genital C. trachomatis is ranked as the most frequent sexually transmitted bacterial agent worldwide. The ability of C. trachomatis serovars D through K to ascend the upper genital tract leads to tubal inflammation, ectopic pregnancy, spontaneous abortion, and infertility in females [4, 5]. Conversely, infection with C. trachomatis serovars L1, 2, and 3 is able to spread to nearby lymph node tissue giving rise to Lymphogranuloma venereum (LGV) [6]. C. pneumoniae is another member species of the genus Chlamydia and is associated with pulmonary infection [7].
Upon infection, Chlamydia sp. can persist for long periods resulting in unevenly distributed, chronic inflammation of infected tissues, and long-term sequela [8]. The asymptomatic nature of chlamydial infection often leads to delayed diagnosis, and lack of proper antibiotic therapy results in to severe tissue damage [9]. This is a major contributing factor for increased prevalence and transmission of Chlamydia sp. infection in recent years. This situation is worrisome as there is no effective prophylactic vaccine available necessitating further investigation for better understanding of the host response to this bacterium. This review provides insight into the molecular means utilized by chlamydial species to the evade immune response.
2. The Chlamydia Life Cycle and ‘Persistence' in Host Cells
A characteristic of many pathogens is that of ‘persistence', i.e., the continued presence of the pathogen under stressful conditions such as limitation of required nutrient(s) and/or presence of antimicrobial/reagents or immune cells [8]. During the 'persistence' period, the pathogen remains viable but discontinues cell development and reproduction. In this stage, the pathogen is noninfectious and as such undetected by the host immune system. This stage will continue until which time a more favorable environment is re-established. Thus, in 'hide and seek' fashion, the pathogen reemerges once the immune system has been evaded and/or deceived at the infection site.
Chlamydia sp. has a unique biphasic life cycle; wherein, it alternates to and from ‘Elementary Body' and ‘Reticulate Body' forms, i.e., EB and RB, respectively [2]. The EB form is infectious and is metabolically inactive with a rigid outer membrane facilitating binding to and entry into the host cell. Following host cell entry, internalized EB fuses to form an intracytoplasmic inclusion which gives rise to the RB form. Although the RB form is noninfectious, it is metabolically active, and within eight hours after infection begins to multiply followed by release within 24 hours infecting neighboring cells [10–12]. The EB form differs from that of the RB in size, i.e., the EB is much smaller (0.2 μm) compared to RB (0.8 μm) [13]. The EB is often present in semen and/or female genital tract epithelial cell secretions, and thus is transmitted to partners during sexual intercourse [14]. Although the EB form first binds to the epithelial cell surface heparin sulfate proteoglycan [15], it readily interacts with other surface molecules such as the mannose receptor [16] or glycosylation-dependent galectin–receptor [15] to trigger and facilitate internalization.
Chlamydia sp. become persistent, i.e., enter the ‘persistence' stage between EB and RB stages enabling the bacterium to survive during unfavorable conditions facilitating its long-term survival in the host, e.g., cellular stress associated with immunological host response eliciting proinflammatory cytokines, antibodies, and antimicrobial substances [8]. Once the required nutrient, e.g., amino acid, or immunological host response mediator molecule(s) return to normal prestress levels, the 'persistence' phase is no longer needed.
For example, IFN-γ secreted from immune cells promotes C. trachomatis entry into the ‘persistence' stage. IFN-γ induces expression of Indoleamine-2,3-dioxygenase (IDO) enzyme which degrades and thus depletes tryptophan which is required for C. trachomatis growth (Figure 1(a)) [17, 18]. Therefore, the presence of host IDO brings about amino acid deprivation, i.e., stress that can lead to death and clearance of the pathogen. In order to avoid this specific stress scenario, C. trachomatis enters a ‘persistence' phase that negates the need to consume tryptophan becoming undetectable by immune cells [19]. Conversely, reduced IFN-γ production and concomitant increased tryptophan concentration promote C. trachomatis reverting to its normal RB-EB life cycle, and may lead to recurrences in patients [20]. Additionally, C. trachomatis avoids tryptophan depletion via release of Tryptophan synthase (TrpBA) protein [21, 22]. The α-subunit of the Tryptophan synthase converts indole glycerol 3-phosphate (IGP) to indole; whereas, the β-subunit converts indole into tryptophan. In the genital tract, this protein induces tryptophan storage, thus providing a continuous supply of tryptophan required for bacteria metabolism.
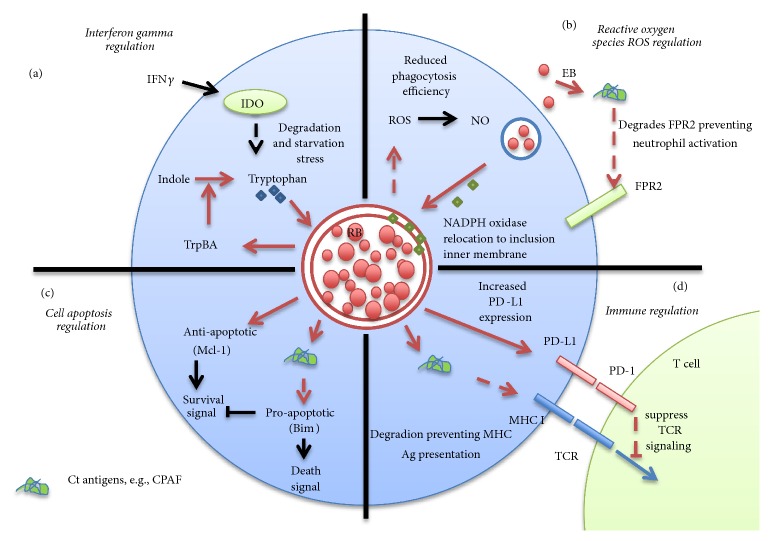
Immune evasion tactics utilized by Chlamydia sp. (a) Release of interferon γ (IFNγ) from immune cells induces expression of Indoleamine 2,3-dioxgenase (IDO) which degrades tryptophan, an essential amino acid required for Chlamydia sp. replication. IDO-mediated tryptophan depletion gives rise to bacterial stress, i.e., starvation. Under such conditions, Chlamydia sp. can produce tryptophan synthase (TrpBA) that converts indole to tryptophan. To avoid a ‘continuous' stress situation, Chlamydia sp. enters a ‘persistence' stage until which time the supply of tryptophan is restored. (b) Chlamydia sp. suppresses production of reactive oxygen species (ROS) and nitric oxide (NO) reducing the efficiency of phagocyte bacterial killing in phagolysosome. NADPH oxidase which is typically located on the phagolysomal membrane assists in production of bactericidal Myeloperoxidase (MPO) and hypochlorous acid (HOCl−). In Chlamydia-infected cells, the subunit of NADPH oxidase is relocated to the inner membrane of the inclusion rather than the phagolysosome. As a consequence, Chlamydia sp. are able to survive in the phagocyte. In the neutrophil, production of chlamydial antigens such as Chlamydial-protease-like activity factor (CPAF) causes degradation of neutrophil surface formal peptide receptor inhibiting activation of neutrophils impeding neutrophil extracellular trap (NET) activity. (c) Release of CPAF from Chlamydia sp. induces expression of antiapoptotic molecule myeloid leukemia cell differentiation protein (Mcl-1) promoting degradation of proapoptotic molecules such as BCL-2-like protein 11 (Bim). Thus, Chlamydia sp. block host cell apoptosis leading to a longer period of persistence, i.e., replication within host cells. (d) CPAF degrades the major histocompatibility complex (MHC) preventing antigen presentation to T cells. Additionally, Chlamydia sp. increases PD-L1 expression in host cells. Binding of PD-L1 to the PD-1 receptor on the T cell surface constitutes a negative signal suppressing T cell receptor (TCR) activation signaling. Broken arrows denote degradation.
Even in the ‘persistence' stage, C. trachomatis can cause damage to the host. Although C. trachomatis discontinues production of most structural and membrane components, it has been shown to synthesize and release a 60 kDa heat shock protein (Hsp60). The presence of Hsp60 protein is thought to cause trophoblast apoptosis leading to fallopian tube epithelial cell damage, and scar formation [23]. Since chlamydial Hsp60 shares high homology with that of human Hsp60 protein produced by human embryonic cells, the immune response elicited against chlamydial Hsp60 is thought to harm the developing embryo leading to spontaneous abortion. However, clinical data have yet to demonstrate a correlation between Hsp60 antibodies and recurrent abortion [24].
3. Chlamydial Infection Paralyses Neutrophil Extracellular Trap Formation
A number of studies have focused on characterization of polymorphic nuclear leukocytes (PMNs) or neutrophils in the pathologies caused by Chlamydia sp. given that a rapid influx of neutrophils frequently accompanies infection of either the genital or pulmonary tracts. Bacteria eradication by neutrophils usually include common neutrophil functions, i.e., phagocytosis, release of defensins, and Neutrophil extracellular trap (NET) formation. The process of neutrophil extravasation to the mucosal site of infection has been shown to be regulated by Surface beta-2 integrin CD18 in addition to cytokines such as IL-8 and IL-17 [25–27]. Lower IL-8 levels result in less efficient neutrophil transendothelial migration through C. trachomatis-infected human umbilical vein endothelial cells [26]. Reduced leukocyte influx to the site of chlamydial infection in the genital tract is also detected in IL-17RA deficient mice compared to wild type [27].
Zhang and coworkers have demonstrated recruitment of high numbers of neutrophils to the oviduct following intravaginal inoculation with C. muridarum which is associated with more rapid resolving of the hydrosalpinx in different animal models [28]. Conversely, neutrophil depletion using monoclonal antibodies demonstrate approximately 6-fold higher bacterial burden at day 7 following intravaginal bacteria inoculation [25, 29]. Additionally, Lee et al. using a similar monoclonal antibody approach to induce neutropenia in mouse demonstrated reduced histopathological parameters, and reduced rates of hydrosalpinx following resolution of the infection [30]. Bai et al. propose that neutrophils play a limited role in clearance of bacteria. In C. trachomatis-infected C3H mouse lung, severe pathology is observed in contrast to the C57BL/6 mouse model; however, the former displays persistence, and more abundant neutrophil infiltration [31]. Furthermore, using the C-X-C chemokine receptor 2 motif (CXCR2) deficient mouse model which is characterized by impaired neutrophil recruitment, no difference in C. trachomatis pulmonary infected and uninfected wild type animals is observed [31]. Surprisingly, rather than affording protection, Rodriguez et al. demonstrated the presence of GR1+/CD45+ neutrophils at the site of infection, and enhanced bacteria replication in lung epithelial cells with concomitant increased C. pneumoniae bacterial burden in infected mice [32]. Failure to recruit neutrophils to the infection site has been suggested to be the primary reason for low bacterial burden, and less pathology in chlamydial infected MycD88-deficient mice [32]. However, the presence of neutrophils in the genital tract can also have negative effects, i.e., facilitation of infection by human immunodeficiency virus (HIV) [33].
In addition to infection by C. trachomatis and C. pneumoniae, neutrophil involvement has been observed to play a role in infection by other species such as C. psittaci and C. caviae. Greater ability of C57BL/6 mice in eliminating Chlamydia sp. is correlated with early neutrophil response as well as cytotoxic T cells [34]. When neutrophils are depleted by administration of RB6-8C5 monoclonal antibody intraperitoneally to C. psittaci infected mice, infection-induced abortion is accelerated with infected animals exhibiting a 100-fold higher bacteria burden with widespread necrosis of the uteroplacenta and increased mortality [35]. This could be due to a decrease in the general immune response manifested as a lower number of other leukocytes including macrophages and T cells; however, an altered TH1 response is not observed in the absence of neutrophils, and no clinical changes are observed during secondary infection in neutrophil depleted mice [36]. In ocular C. caviae infected guinea pigs with neutrophil depletion, ocular pathology as well as increased serum IgA, IL-5, and TGF-β but decreased CCL5 are observed [37].
Recently, Rajeeva et al. have suggested a neutrophil evasion strategy utilized by C. trachomatis resulting in paralysis of host cell extrusion of NET (which contains chromatin DNA and proteolytic enzymes released by neutrophils during Neptosis cell death to trap and lyse extracellular bacteria) [38]. Cleavage and release of neutrophil Surface Formyl peptide receptor 2 (FPR2) by the Chlamydial-protease-like activity factor (CPAF) plays a role in this process as a CPAF target affecting oxidative burst interfering with chemical-mediated activation of neutrophils (Figure 1(b)). Increased secretion of specific defensin types, i.e., Human neutrophil peptides (HNP1-3), are detected in C. trachomatis infected patients with urethritis [39]. However, clinical studies have revealed higher HNP1-3 secretion in the vagina of infected females correlating with a higher risk of endometriosis and bacterial ascension, and pelvic inflammatory disease (PID) pathogenesis [40] supporting a negative rather than protective role for neutrophils.
Neutrophil recruitment to the site of infection is also dependent on the presence of virulence factors, e.g., the 7.5 kb cryptic plasmid. Infection with a plasmid-bearing C. trachomatis strain triggers a more rapid release of soluble factors from oviduct epithelial cells leading to a higher abundance of neutrophils with prolonged survival at the infection site [41], and severe clinical symptoms observed in female patients [22].
4. C. pneumoniae Hides from Phagocytosis
Oxidative stress via NADPH oxidase in human neutrophils or HeLa cells has been shown to be inhibited by C. trachomatis infection [42, 43]. The mechanism utilized by C. trachomatis involves relocation of Ras-related C3 botulinum toxin substrate 1 (Rac1), a regulatory subunit of NADPH oxidase to the inclusion reducing phagocytosis efficiency (Figure 1(b)) [43]. Fluorescence lifetime imaging data suggest NADPH is relocated to the inner side of the chlamydial inclusion membrane [44] promoting bacterial glycolytic function, affecting in a negative fashion host cell energy generation.
When macrophages are infected with C. pneumoniae, reactive oxygen species (ROS) are produced via Ca2+ influx, and membrane associated NADPH oxidase [45]. Interestingly, levels of ROS in human monocytes in response to C. pneumoniae are less intensive than that observed for C. trachomatis. Thus, C. pneumoniae is able to survive longer than C. trachomatis in human monocytes [46]. C. trachomatis infectivity in monocytes can be restored by treatment with NADPH oxidase or Nitric oxide synthase inhibitors implying that phagocytic cells utilize ROS and/or nitric oxide (NO) for bacterial eradication [46]. ROS release during chlamydial infection is Nucleotide-binding oligomerization domain, leucine rich repeat containing X1 (NLRX1) dependent, and is turned on rapidly upon infection, but switched off only a few hours after infection [47]. C. trachomatis selectively stimulates Myeloperoxidase release, but not superoxide production by human neutrophils [48].
5. Chlamydia sp. Inhibits Host Cell Apoptosis
C. pneumoniae is able to infect and inhibit host cell apoptosis defense function by lowering Procaspase 3 processing with concomitant induction of IL-8; thus, maintaining expression of antiapoptotic Induced myeloid leukemia cell differentiation protein (Mcl-1) via activation of PI3K/Akt and ERK1/2 pathways (Figure 1(c)) [49, 50]. This enables the bacterium to reside and hide inside the neutrophil for up to 90 hours compared to 10 hours in noninfected neutrophils [50]. When infected neutrophils undergo apoptosis and are eventually ingested by neighboring macrophages, bacteria are able to replicate and persist longer. Infection of macrophage through apoptotic neutrophils induces Tumor growth factor-β (TGF-β) secretion compared to TGF-α following direct infection of macrophage with bacteria [51] facilitating the hiding of bacteria, i.e., remaining protected when taken up by long-lived macrophages. CPAF contributes to chlamydial antiapoptotic activity by degrading the proapoptotic BH3-only B-cell lymphoma-2 (BCL-2) subfamily death effector members such as BCL-2-like protein 11 (BIM), p53 upregulated modulator of apoptosis (PUMA), and BCL-2-associated death promoter (BAD) [52]. BIM protein has been observed to disappear during chlamydial infection, and this disappearance could be inhibited by proteasome inhibitors [53]. These proapoptotic molecules transmit death signals to mitochondria inhibiting both BCL-2 pro/antiapoptotic molecules which activate proapoptotic BCL-2-associated X protein (BAX), and BCL-2 homologous antagonist killer (BAK) [54]. Thus, degradation of proapoptotic molecules confers resistance to apoptosis during cellular chlamydial infection.
6. C. trachomatis Suppresses Class I/II MHC to Avoid Immune Detection by T Cells
Intravaginal inoculation with C. trachomatis in the mouse chlamydial model causes recruitment of uterine infiltrate composed of a large number of CD45+ mononuclear cells that express surface Class II major histocompatibility complex (MHC), and the co-stimulatory CD86 molecule to induce T cell activation [55]. Class II MHC is required for immunity to C. trachomatis as evidenced by class II deficiency derived from inactivation of the I-Aβ gene which exhibited a lower concentration of all anti-chlamydia antibody isotypes resulting in failure to resolve the infection compared to wild-type mice after 3 weeks [56]. Likewise, athymic nude or CD4+ T cell depleted mice also exhibit a profound delay in infection resolution [55, 56] suggesting that engagement of both antigen presentation and helper T cells is required in resolving C. trachomatis infection. Involvement of Class II MHC antigen presentation has also been shown through identification of several chlamydial peptides retrieved from Class II MHC-bound peptides eluted from dendritic cells (DCs) pulsed with live or dead C. muridarum elementary bodies (EBs) [57].
Many intracellular pathogens especially viruses have been shown to suppress MHC expression or surface presentation to avoid detection by the adaptive immune system. For example, human cytomegalovirus (HCMV) is able to suppress both Class I and II MHC molecules through the Unique short-2 (US2) and -11 (US11) proteins which target newly synthesized MHC molecules causing ubiquitination, and relocation to the cytosol for proteasome degradation [58, 59]. In contrast, HIV negative regulatory factor (Nef) protein diverts transport of Class I MHC to organelles rather than to the cell surface causing accumulation in cells [60, 61]. Nef also induces immature Class II MHC with invariant chain and accelerates endocytic removal of surface class II MHC molecules [62, 63].
As an intracellular bacterial pathogen, it is likely that C. trachomatis avoids immune detection by hiding from or interfering with MHC presentation. During the developmental cycle, C. trachomatis remains confined within a protective inclusion-like vacuole avoiding Class I MHC presentation (Figure 1(d)). In 1999, Zhong et al. reported C. trachomatis to inhibit Class II MHC expression [64]. It has been shown in several cell types (MRC-5 human lung fibroblast, 2C4 mouse B cells, and Hela cervical epithelial cells) that C. trachomatis infection blocks interferon-γ (IFN-γ) inducible class II MHC (HLA-DR) expression [64]. Further investigation has demonstrated Class II MHC expression is inhibited through indirect degradation of the Upstream Stimulatory Factor-1 (USF-1). USF-1 is a constitutive, ubiquitously expressed transcription factor required for expression of IFN-γ induction of Class II Transactivator (CIITA) which mediates MHC class II expression. Additional studies have revealed other potential targets of CPAF such as proapoptotic BIM and PUMA [52], Nuclear Factor-kB (NF-kB) p65 [65], MHC-like Cd1d [66], and Nectin cell adhesion molecule 1 (NECTIN1) [67].
Subsequent to demonstrating C. trachomatis-mediated inhibition of Class II MHC expression, Zhong and colleagues reported in C. trachomatis that CPAF inhibits Class I MHC by targeting USF-1 [68]. Both constitutive and IFN-γ-induced Class I MHC are inhibited in Chlamydia infected cells. CPAF, residing in the host cell cytoplasm during infection is responsible for degrading USF-1 and Regulatory Factor X5 (RFX5) proteins. As mentioned above, USF-1 regulates class II MHC through CIITA; whereas, RFX5 is a member of the RFX transcription factor complex that is required for binding to the X1 regulatory element upstream of MHC Class I heavy chain, and β2-microglobulin (β2M) genes [69]. Importantly, CPAF is homologous across species, and recombinant CPAF from C. pneumoniae has also been shown to degrade RFX5 impairing Class I MHC expression [70].
Cluster of differentiation d protein (CD1d) is a MHC-like molecule expressed by epithelial cells, and binds to and presents glycolipid antigens to natural killer T cells [71, 72]. Interestingly, Kawana et al. demonstrated CD1d is downregulated by C. trachomatis in human penile urethral epithelial cells [66]. This process also involves CPAF-mediated ubiquitination and degradation of CD1d heavy chain. In chlamydial infected cells, CD1d heavy chains have been shown to relocate to the cytosol and chlamydial inclusion vacuole rather than being transported to the cell surface.
In addition to protease-mediated Class I MHC degradation, Caspar-Bauguil et al. reported IL-10 secretion by infected cells could play a role in Class I MHC inhibition [73]. C. pneumoniae infection of U937 human monocytic cells causes suppression of Class I MHC expression, a reaction that could be reversed by addition of anti-IL-10 neutralizing antibody. Furthermore, addition of recombinant IL-10 alone is able to reduce Class I MHC expression in these cells suppressing bacterial epitope presentation and attenuation of T cell mediated elimination of bacteria.
7. Induction of PD-L1 in Chlamydia Infected Cells Causes T Cell Exhaustion
Increased expression of Programmed cell death protein-1 (PD-1) is indicative of T cell exhaustion as evidenced in many types of chronic viral infections [74]. PD-1 binding to its ligands (PD-L1 and PD-L2) on antigen presenting cells suppresses T cell receptor signaling-mediated activation conferring T cell persistence in the ‘exhausted state' which is characterized by unresponsiveness to antigen exposure, loss of cytotoxicity, and cytokine, i.e., IL-2, TNFα, and IFNγ production [75]. In recent years, antibody and cell immunotherapeutic approaches used to interfere with PD-1 or its ligands have proven to be clinically affective as evidenced by the conferring of the 2018 Nobel Prize in Medicine/Physiology. By targeting PD-1 signaling, T cell exhaustion during chronic infection can be reversed reinvigorating T cell activity for active pathogen clearance. This therapeutic approach initially used in cancer immunotherapy has also been applied in clinical intervention of viral pathogens such as HIV [76]. Most T cell exhaustion studies are conducted using CD8+ cytotoxic T cells, and chronic viral-mediated infection models. However, the focus of a few studies has been on characterization of the involvement of PD-1 signaling in bacterial infection. Given that Chlamydia is an obligate intracellular parasite phenotypically analogous to that of the viral life cycle with its long-term host persistence, some studies have begun to elucidate the potential role of PD-1 signaling in the host response to this pathogen (Figure 1(d)).
Although the principal function of cell-mediated immunity is interdiction and eradication of intracellular pathogens, the focus of most chlamydial studies to date is not on CD8+ T cell response because CD8+ T cell involvement has been shown to play a minimal role in C. trachomatis immunity in genital tract infection in the murine model [77]. Fankhauser et al. have attributed poor CD8+ T response during genital C. trachomatis infection to PD-1 signaling [78]. Having measured the number of immune-dominant antigen Cysteine-rich membrane protein- (CrpA-) Class I tetramer specific CD8+ T cells in the genital mucosa, a high number of infiltrating CD8+T cells during primary intracervical C. trachomatis infection was observed with clearing, i.e., resolving after 4 weeks. However, CD8+ T cells were greatly diminished at the genital mucosa upon secondary reinfection after 5 weeks reminiscent of chronic viral pathogen infection [74, 79]. Administration of anti-CD8 depleting antibody shows no difference in the ability to clear bacteria suggesting that memory CD8+ T cells have an impaired ability to expand; thus, not contributing to control of C. trachomatis during secondary infection. This defective response during secondary infection is attributed to a 10-fold higher expression of PD-L1 in the uterus of infected mice that contributes to impaired bacterial clearance from the host [78]. PD-L1 engagement results in lower IFN-γ secretion from CD8+ T cells while inhibition of PD-L1 restores the CD8+ response. After primary transcervical infection, PD-L1 deficient mice exhibit lower bacterial load. Thus, deletion or inhibition of the PD1/PD-L1 pathway improves the CD8+ T cell response resulting in enhanced bacterial clearance.
In a recent study using a C. muridarum mouse lung infection model, Shekhar et al. demonstrated PD-L1 expression in two different subsets of pulmonary dendritic cells, i.e., CD103−CD11bhigh and CD103+CD11blow [80]. CD11bhigh dendritic cells are associated with effector response and inflammation; whereas, CD103+ dendritic cells are linked to T Helper 2 and regulatory T cells [81]. Both populations exhibit equal levels of PD-L1 expression in response to infection. Interestingly, when the PD1/PD-L1 signaling is blocked by anti-PD1 antibodies in an in vitro coculture experiment, the ability of dendritic cells to promote IFN-γ and IL-17 production and release from CD4+ T cells is greatly enhanced [80]. Thus, these observations suggest that antibody treatment to block PD1/PD-L1 signaling could be employed to enhance dendritic cell promotion of the TH1/TH17 response boosting protective immunity to C. trachomatis infection.
Conversely, Peng et al. demonstrated a contradictory role for PD-1 in C. muridarum genital infection [82]. Administration of neutralizing antibodies against PD-L1 and co-inhibitory T-cell immunoglobulin and mucin-domain containing-3 (TIM3) has no effect on bacteria shedding during early stage infection [82]. However, when mice were harvested at 60 days after infection, increased hydrosalpinx scores and severe inflammatory response in the uterine horn and oviduct of the upper genital tract are observed suggesting that PD-1/PD-L1 and Tim3 may negatively regulate pathology attenuation in chronic chlamydial infection. Most likely, the different results observed in genital chlamydial infection following PD-1/PD-L1 signaling interference are attributed to CD8+ T cell involvement in either protection or pathology [83].
As previously indicated, a 7.5 kb cryptic C. trachomatis plasmid has been implicated as one of several virulence factors associated with more severe pathology in both human and mouse studies. In a transcriptional profiling analysis, Porcella et al. report that plasmid-bearing C. trachomatis strains enhance expression of PD-L1 two-fold compared to plasmid-deficient strains in human epithelial cells [84]. In addition to PD-L1, other immune suppression-related molecules, e.g., NF-κB inhibitor β protein (NF-κBIβ), and Tumor necrosis factor-α inducing protein 3 (TNFαIP3) are also expressed at higher levels in epithelial cells infected with plasmid-bearing strains suggesting that one of eight genes encoded by the plasmid may act to switch off specific immune functions underscoring the need to further investigate and better understand the plasmid immune suppression mechanism.
8. Conclusion
Despite studies to improve diagnosis, treatment, and vaccine development, the rate of Chlamydia infection has steadily increased worldwide in recent years. This review is a summary of various molecules used by Chlamydia sp. that facilitate long-term survival and replication in the host cell. It is important to note that the existence of various nonimmune evasion strategies of the bacteria, i.e., the ability of Chlamydia sp. to modify the host transcription or proteome profiles [85, 86] is not included in the current review. A better understanding of interactions between Chlamydia sp. and host immune cells is essential for development of better and more effective therapeutic strategies for interdiction of chlamydial infection.
Acknowledgments
This work was supported by the National Institutes of Health (NIH), Grant IR03AI11771401A1, and the Army Research Office (Department of Defense Contract no. W911NF-11-1-0136). Travel support from the Fulbright Visiting Scholar Program is gratefully acknowledged.
References
Articles from Journal of Pathogens are provided here courtesy of Wiley
Citations & impact
Impact metrics
Article citations
A review of the roles of pathogens in Alzheimer's disease.
Front Neurosci, 18:1439055, 19 Aug 2024
Cited by: 0 articles | PMID: 39224577 | PMCID: PMC11366636
Review Free full text in Europe PMC
Recognition of Chlamydia trachomatis by Toll-like receptor 9 is altered during persistence.
Infect Immun, 92(7):e0006324, 20 Jun 2024
Cited by: 0 articles | PMID: 38899879
Intranasal delivery of Salmonella OMVs decorated with Chlamydia trachomatis antigens induces specific local and systemic immune responses.
Hum Vaccin Immunother, 20(1):2330768, 22 Mar 2024
Cited by: 1 article | PMID: 38517203 | PMCID: PMC10962599
Chlamydiae as symbionts of photosynthetic dinoflagellates.
ISME J, 18(1):wrae139, 01 Jan 2024
Cited by: 0 articles | PMID: 39046276 | PMCID: PMC11317633
IgG exacerbates genital chlamydial pathology in females by enhancing pathogenic CD8<sup>+</sup> T cell responses.
Scand J Immunol, 99(1):e13331, 13 Oct 2023
Cited by: 2 articles | PMID: 38441219
Go to all (16) article citations
Similar Articles
To arrive at the top five similar articles we use a word-weighted algorithm to compare words from the Title and Abstract of each citation.
Chlamydia psittaci: update on an underestimated zoonotic agent.
Pathog Dis, 73(1):1-15, 04 Dec 2014
Cited by: 116 articles | PMID: 25853998
Review
Interaction of pathogenic yeasts with phagocytes: survival, persistence and escape.
Curr Opin Microbiol, 13(4):392-400, 04 Jun 2010
Cited by: 90 articles | PMID: 20627672
Review
Immunometabolic Phenotype Alterations Associated with the Induction of Disease Tolerance and Persistent Asymptomatic Infection of Salmonella in the Chicken Intestine.
Front Immunol, 8:372, 04 Apr 2017
Cited by: 44 articles | PMID: 28421074 | PMCID: PMC5378774
Review Free full text in Europe PMC
Evasion of Human Neutrophil-Mediated Host Defense during Toxoplasma gondii Infection.
mBio, 9(1):e02027-17, 13 Feb 2018
Cited by: 25 articles | PMID: 29440572 | PMCID: PMC5821086
Funding
Funders who supported this work.
NIAID NIH HHS (1)
Grant ID: R03 AI117714
National Institutes of Health (NIH) (2)
Grant ID: IR03AI11771401A1
Grant ID: W911NF-11-1-0136