Abstract
Free full text

Peripheral TREM1 responses to brain and intestinal immunogens amplify stroke severity
Associated Data
Abstract
Stroke is a multiphasic process in which initial cerebral ischemia is followed by secondary injury from immune responses to ischemic brain components. Here we demonstrate that peripheral CD11b+CD45+ myeloid cells magnify stroke injury via activation of triggering receptor expressed on myeloid cells 1 (TREM1), an amplifier of proinflammatory innate immune responses. TREM1 was induced within hours after stroke peripherally in CD11b+CD45+ cells trafficking to ischemic brain. TREM1 inhibition genetically or pharmacologically improved outcome via protective antioxidant and anti-inflammatory mechanisms. Positron electron tomography imaging using radiolabeled antibody recognizing TREM1 revealed elevated TREM1 expression in spleen and, unexpectedly, in intestine. In the lamina propria, noradrenergic-dependent increases in gut permeability induced TREM1 on inflammatory Ly6C+MHCII+ macrophages, further increasing epithelial permeability and facilitating bacterial translocation across the gut barrier. Thus, following stroke, peripheral TREM1 induction amplifies proinflammatory responses to both brain-derived and intestinal-derived immunogenic components. Critically, targeting this specific innate immune pathway reduces cerebral injury.
Stroke is the fourth leading cause of death and the leading cause of disability. Thrombolytic therapy and endovascular removal of thrombi are the only approved therapies for acute ischemic stroke and must be instituted early after the ischemic event. Because of this limited window of therapeutic time, only a small percentage of stroke patients have so far benefited from these interventions1. On the other hand, innate and adaptive immune responses initiated after cerebral ischemia unfold over days to weeks after stroke2–6. Cerebral ischemia causes release of highly immunogenic cellular components, or danger/damage-associated molecular patterns (DAMPs), from the brain into the systemic circulation. These DAMPs activate and recruit peripheral innate and adaptive immune cells to ischemic brain regions. Experimental manipulations suggest that both toxic and protective inflammatory processes are activated after stroke7–11, with toxic effects including generation of proinflammatory cytokines, proteases and reactive oxygen species by inflammatory cells, and protective effects consisting of clearance of injured tissue by myeloid cells and the establishment of a regenerative environment12–14. Therefore, identification of molecular and cellular pathways that could either enhance the beneficial effects or dampen the toxic effects of post-stroke inflammation will be critical in developing novel therapies to improve stroke outcome.
In this study, we hypothesized that attenuating the proinflammatory component of the post-stroke immune response might reduce stroke severity. Triggering receptor expressed in myeloid cells 1 (TREM1), an inflammatory type I membrane receptor, is expressed on myeloid lineage cells and is a potent amplifier of innate immune responses15,16. TREM1 magnifies the proinflammatory response by synergizing with classical pattern recognition receptors, such as Toll-like receptors and Nod-like receptors. TREM1 amplifies proinflammatory cytokine and chemokine production, including interleukin-8 (IL-8), monocyte chemoattractant protein-1 (MCP-1), MCP-3 and macrophage inflammatory protein-1α (MIP-1α)15, inhibits IL-1017 and enhances protease production, neutrophil degranulation and oxidative bursts18. Such TREM1-mediated amplification of proinflammatory immune responses worsens outcome in models of infection, inflammatory bowel disease19,20 and sepsis21–24, and in non-infectious models, including rheumatoid arthritis25, pancreatitis26, atherosclerosis27, cancer28 and fibrosis29.
Since brain ischemia leads to the release of cellular proteins, lipids and other immunogenic materials from brain, we hypothesized that early post-stroke innate immune responses may be amplified by TREM1. Here we show that, in a rodent model of transient focal cerebral ischemia, TREM1 is selectively induced in peripheral myeloid cells that traffic to the ischemic brain and that inhibition of TREM1 reduces stroke injury. Peripheral TREM1 induction occurs not only in spleen, but also in intestinal inflammatory macrophage subsets following sympathetic-mediated increases in gut permeability; we find that intestinal TREM1 amplifies gut permeability, the peripheral innate immune response and bacterial translocation to the periphery. These findings provide evidence that peripheral myeloid cells worsen cerebral injury via TREM1 amplification of immune responses to both sterile brain components and gut microbial pathogens.
Results
TREM1+ myeloid cells infiltrate ischemic brain.
The middle cerebral artery occlusion-reperfusion model of transient focal cerebral ischemia (MCAo) is a widely used model for stroke, a condition characterized by temporary occlusion of an arterial vessel followed by reperfusion into ischemic brain tissue (Fig. 1a). We confirmed the dynamics of myeloid cell trafficking from the periphery to ischemic brain 48 h after MCAo in Cx3cr1GFP/+Ccr2RFP/+ double transgenic mice30,31 (Fig. 1b,,c).c). Analysis of Cx3cr1GFP/+Ccr2RFP/+ mice allows for simultaneous tracking of green fluorescent protein (GFP+) microglia and red fluorescent protein (RFP+) peripheral monocytes/macrophages (Mo/MΦ) and polymorphonuclear neutrophils (PMNs) that have trafficked to ischemic brain32. CD11b+ myeloid cells were stained with anti-CD45, to distinguish CD45int-resident microglia from CD45hi peripherally derived Mo/MΦ, anti-Ly6G, to identify PMNs, and were evaluated for GFP and RFP expression; NK cells, which are CD11b+ non-myeloid cells and infiltrate the ischemic hemisphere33, were not evaluated in this study (Fig. 1c).

a, Diagram of the MCAo model (internal carotid artery, ICA; middle cerebral artery, MCA) carried out in 2–3-month-old male C57B6/J mice. b, Cx3cr1GFP/+Ccr2RFP/+ mice were subjected to MCAo and examined for myeloid cell populations present in ischemic hemisphere 2 d later. Plots identify CD11b+CD45hiLy6G− Mo/MΦ, CD11b+CD45int microglia and CD11b+CD45hiLy6Ghi PMN. c, Representative histograms of GFP-positive CD11b+CD45int microglia and RFP-positive CD11b+CD45hiLy6G−Mo/MΦ and CD11b+CD45hiLy6G+ PMNs 2 d after MCAo. See Supplementary Fig. 1a–c. d, Cx3cr1GFP/+Ccr2RFP/+ mice were assayed for TREM1 surface expression in ischemic hemisphere 2 d after MCAo. Peripherally derived RFP-positive CD11b+CD45hi Mo/MΦ and PMNs express TREM1, while CD11b+CD45int microglia do not. e, Percentages of TREM1+ CD11b+CD45+ myeloid cells at 2 d and 6 d after MCAo in ipsilateral (IL) ischemic hemisphere relative to contralateral (CL) non-ischemic and sham IL and CL hemispheres (n = 6 biologically independent samples per group at 2 d and n = 7 per group at 6 d, mean ± s.e.m.; two-tailed Student’s t-test **P < 0.01, *P < 0.05 for comparison of IL MCAo and sham hemispheres at 2 and 6 d, respectively). See Supplementary Fig. 1d,e. f, MFI of TREM1 on CD11b+CD45hiLy6G+ PMNs, CD11b+CD45hiLy6G− Mo/MΦ and CD11b+CD45int microglia at 2 d and 6 d after MCAo; n = 6 biologically independent samples per group at 2 d and n = 7 at 6 d, mean ± s.e.m.; two-way ANOVA for IL MCAo versus IL sham hemispheres; effect of MCAo in Mo/MΦ: P < 0.001, effect of time P = 0.0154; effect of time in microglia P < 0.001; Bonferroni post-hoc comparisons: ***P < 0.001 for Day 2 IL MCAo versus IL sham hemisphere and P < 0.01 or ****P < 0.001 for Day 6 IL MCAo versus Day 6 IL sham hemisphere). See Supplementary Fig. 1f.
We also assessed changes in myeloid cell dynamics over the first 7 d following MCAo (Supplementary Fig. 1a–c) and confirmed an early increase of CD11b+CD45hi Mo/MΦ and CD11+CD45hiLy6Ghi PMNs by 48 h after MCAo, which plateaued and then declined after Day 4. The rise in infiltrating Mo/MΦ and PMNs was associated with a corresponding decline in CD11b+CD45int microglia, presumably from loss of microglia in the ischemic hemisphere.
We then tested whether TREM1 expression was induced in brain myeloid cells after cerebral ischemia. Examination of Cx3cr1GFP/+Ccr2RFP/+ ischemic ipsilateral hemispheres demonstrated high surface expression of TREM1 on CD11b+CD45hi myeloid cells, but not on resident CD11b+CD45int microglia at 48 h after MCAo (Fig. 1d and Supplementary Fig. 1d). TREM1 expression increased in ischemic IL compared to sham IL hemispheres in CD11b+CD45+ myeloid cells at 2 and 6 d after MCAo (Fig. 1e and Supplementary Fig. 1e). High TREM1 expression was observed on both infiltrating CD11b+CD45hi Mo/MΦ and CD11b+CD45hiLy6G+ PMNs 48 h after MCAo (Fig. 1f and Supplementary Fig. 1f), with the largest fold induction in Mo/MΦ that basally express less TREM1 than PMNs. PMNs showed consistently high cell surface expression of TREM1, which was comparable to levels seen on PMNs from sham controls; microglia showed low levels of TREM1 that did not change with cerebral ischemia. Thus, TREM1+ Mo/MΦ and PMNs infiltrate the ischemic hemisphere from the periphery after stroke.
Early induction of peripheral TREM1 after stroke.
As TREM1 was expressed predominantly on infiltrating Ccr2RFP+/− cells, we investigated the time course of TREM1 surface induction in peripheral myeloid cells. Previous studies have highlighted an important role of the spleen as a reservoir for myeloid cells that traffic to injured myocardial tissue34, so we examined changes in splenic myeloid cell populations after MCAo (Fig. 2a,,b).b). Over the initial 7 d after MCAo, there was a significant decline in splenic PMNs beginning at Day 2, with a trend in decline of Mo/MΦ as well, probably reflecting an egress of myeloid cells transiting out of the spleen to the ischemic hemisphere.
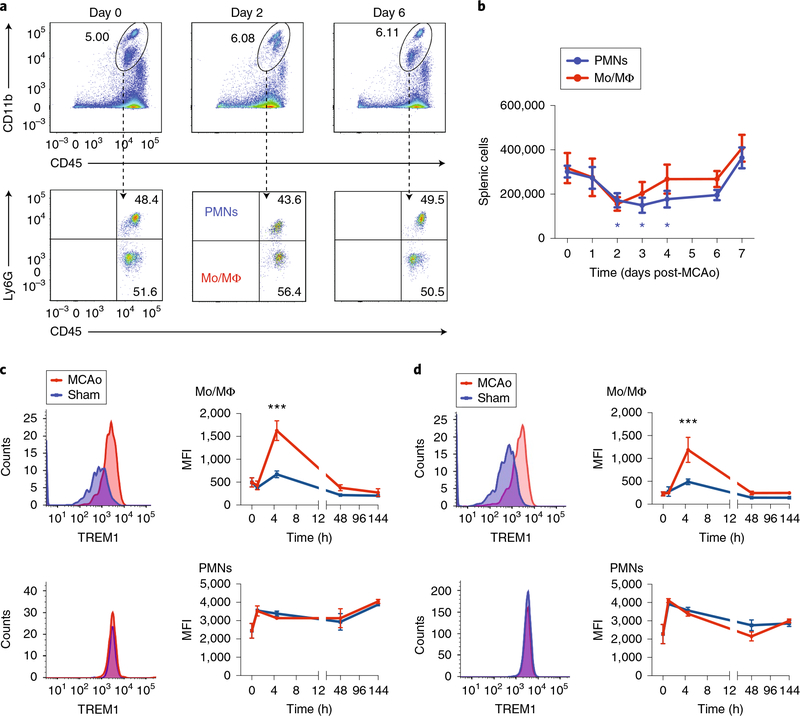
a, Representative plots of CD11b+CD45hiLy6G− Mo/MΦ and CD11b+CD45hiLy6Ghi PMN populations at Days 0, 2 and 6 after MCAo in spleen. b, Time course of peripheral myeloid cell dynamics in spleen for 7 d after MCAo (n = 6–8 biologically independent samples per cell type per time point, mean ± s.e.m.; one-way ANOVA per cell type; effect of PMN, P = 0.0015, effect of Mo/MΦ P = 0.06; Tukey post-hoc *P < 0.05). c, Temporal course of TREM1 expression in blood. Representative histograms of TREM1 expression on Mo/MΦ (top) and PMNs (bottom) at 4.5 h after reperfusion compared to sham surgery (left panels). Time course of TREM1 MFI changes in Mo/MΦ and PMNs at 0 h before sham surgery or MCAo, then 1, 4.5, 48 and 144 h (6 d) after sham surgery or MCAo (right panels) (n = 3–12 biologically independent samples per treatment group per time point, mean ± s.e.m.; two-way ANOVA, effect of MCAo P < 0.01, effect of time P < 0.0001; Bonferroni post-hoc ***P < 0.001 at 4.5 h for MCAo versus sham in blood Mo/MΦ). See Supplementary Fig. 2a–c. d, Temporal course of TREM1 expression in spleen. Representative histograms of TREM1 expression on Mo/MΦ and PMNs at 4.5 h after reperfusion compared to sham surgery (left panels). Time course of TREM1 MFI changes in Mo/MΦ and PMNs before sham surgery or MCAo at 0 h, and after at 1, 4.5, 48 and 144 h (6 d) (right panels) (n = 3–12 biologically independent samples per treatment group per time point, mean ± s.e.m.; two-way ANOVA, effect of MCAo P < 0.01, effect of time P < 0.0001; Bonferroni post-hoc ***P < 0.001 at 4.5 h for MCAo versus sham in splenic Mo/MΦ).
We next examined the time course of the appearance of TREM1-expressing cells in the periphery in blood and spleen at 1 h, 4.5 h, 2 d and 6 d (144 h) after either sham surgery or MCAo (Fig. 2c,,d).d). Basal levels of TREM1 surface expression differed markedly between macrophages and neutrophils; under physiologic conditions, TREM1+ Mo/MΦ and PMNs comprised 10.74% and 90.04% of blood Mo/MΦ and PMNs, respectively, and 5.18% and 65.62% of splenic Mo/MΦ and PMNs, respectively (Supplementary Fig. 2), consistent with previously observed PMN frequencies24. A significant induction of TREM1 expression was evident early, at 4.5 h after MCAo, in peripheral Mo/MΦ in both blood and spleen (Fig. 2c,,d).d). Comparisons of peripheral versus brain-infiltrating Mo/MΦ showed a marked increase in TREM1 mean fluorescence intensity (MFI) in the ischemic hemisphere compared with blood Mo/MΦ at Day 2 after MCAo (Supplementary Fig. 2c). Systemic innate immune responses are thus triggered early, within hours after MCAo, with TREM1+ myeloid cells subsequently appearing in the ischemic hemisphere after MCAo.
TREM1 immune amplification worsens stroke outcome.
We next tested the effect of genetic Trem1 ablation24 and elimination of TREM1-mediated immune amplification following MCAo. Flow cytometry revealed a gene dose-dependent reduction in TREM1 MFI and frequency of TREM1+ cell subsets (Fig. 3a,,bb and Supplementary Fig. 3a), where maximal expression in wild-type (WT) mice was observed on infiltrating PMNs, ~50% lower in Mo/MΦ and negligible in microglia. Trem1 deletion improved neurological scores at 24 h and 48 h and improved survival, with a 2-d survival of 93.02% in Trem1−/− mice compared to 75.67% and 76.19% in Trem1+/+ and Trem1+/− mice, respectively (Fig. 3c,,d).d). Infarct volumes were significantly decreased in Trem1−/− mice at 48 h after MCAo (Fig. 3e,,f).f). These findings suggest that mortality accompanying the MCAo reperfusion model is enhanced by TREM1-mediated amplification of post-ischemic innate immune responses.
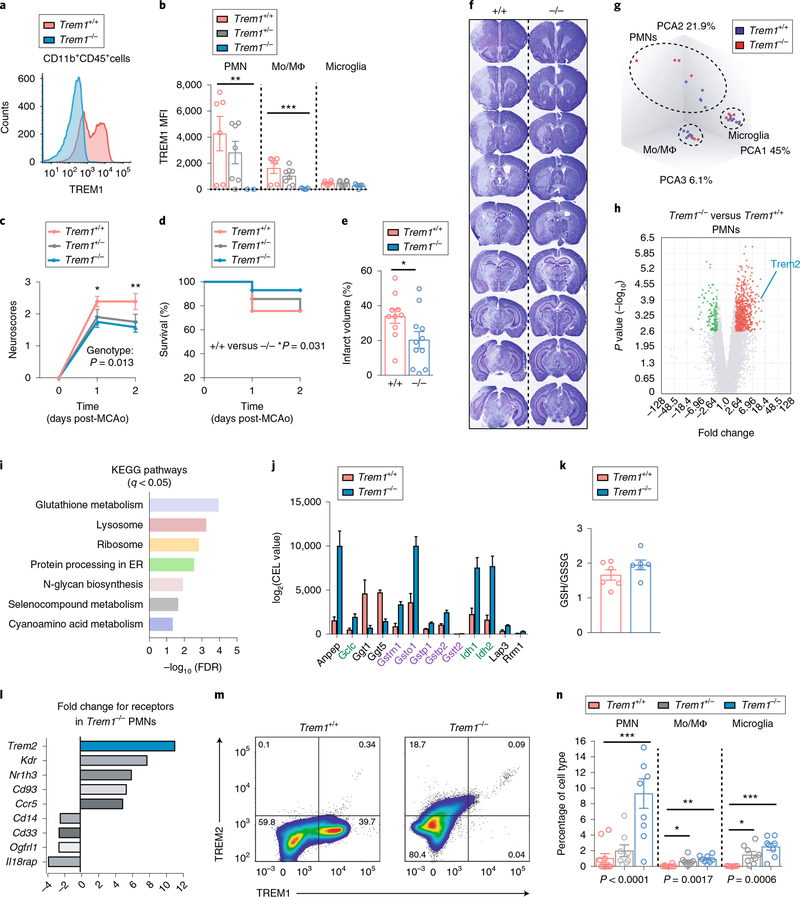
a, Representative histogram of TREM1 expression in Trem1+/+ and Trem1−/− CD11b+CD45+ cells 2 d after MCAo. b, TREM1 expression in CD11b+CD45hiLy6Ghi PMNs, CD11b+CD45hiLy6G− Mo/MΦ and CD11b+CD45int microglia 2 d after MCAo from Trem1+/+, Trem1+/− and Trem1−/− ischemic hemispheres (see Supplementary Fig. 3a). c, Neurological scores after MCAo in Trem1+/+, Trem1+/− and Trem1−/− mice (n = 9 Trem1+/+, n = 10 Trem1+/− and n = 6 Trem1−/−, mean ± s.e.m.; two-way ANOVA, repeated measures, effect of genotype +/+ versus −/−: *P = 0.0132, effect of time ****P < 0.0001, effect of interaction *P = 0.0236; Bonferroni multiple comparisons *P = 0.031 at Day 1 and **P = 0.0046 at Day 2). d, Survival after MCAo in Trem1+/+, Trem1+/−, and Trem1−/− mice (n = 21–23 per genotype; log-rank test *P = 0.031 for Trem1−/− versus Trem1+/+). e, Cerebral infarct volume, measured as a percentage of corrected infarct volume (see Methods), is reduced in Trem1−/− mice 2 d after MCAo (n = 10–11 biologically independent samples per genotype, mean ± s.e.m. group; P < 0.05, two-tailed Student’s t-test). f, Representative sections of Trem1+/+ and Trem1−/− brains stained with cresyl violet 2 d after MCAo. Cresyl violet stain is lost in ischemic regions. g, PCA of sorted CD11b+CD45hiLy6Ghi PMNs, CD11b+CD45hiLy6G− Mo/MΦ and CD11b+CD45int microglia at 2 d after MCAo (n = 9–11 biologically independent samples per cell type; see Supplementary Fig. 3b). h, Volcano plot of differentially regulated genes in Trem1−/− versus Trem1+/+ neutrophils, absolute fold change ≥ 2 and FDR < 0.05. i, KEGG pathways for enriched genes differentially expressed in Trem1−/− compared to Trem1+/+ PMNs (FDR < 0.05; absolute fold change ≥ 2; see Supplementary Fig. 3e). j, KEGG glutathione metabolism gene expression for biosynthetic transcripts (biosynthetic gene labels in green; GSH-conjugating in purple) in Trem1−/− compared to Trem1+/+ PMNs. k, LC–MS measurements of GSH and GSSG in mouse peritoneal macrophages isolated from Trem1+/+ and Trem1−/− mice (n = 6 biologically independent samples per group; two-tailed t-test, P = 0.12). l, TREM2 is the most highly upregulated immune receptor in Trem1−/− PMNs. See Supplementary Fig. 3f–h. m, Representative plot of CD11b+CD45+ brain myeloid cells from Trem1+/+ and Trem1−/− ischemic hemispheres 2 d after MCAo (see Supplementary Fig. 3i,j). n, Percentage change in TREM2 expression with deletion of Trem1 in PMN, Mo/MΦ and microglia in ischemic hemisphere 2 d after MCAo (n = 7–10 biologically independent samples per group, mean ± s.e.m.; Student’s two-tailed t-test, *P < 0.05, **P < 0.01 and ***P < 0.001.
Changes induced by TREM1 deficiency after ischemia.
We next determined the molecular changes associated with deletion of Trem1 using whole-transcriptome analysis of CD11b+CD45hiLy6Ghi PMNs, CD11b+CD45hi Mο/MΦ and CD11b+CD45int microglia 2 d after MCAo (Supplementary Fig. 3b). Principal component analysis (PCA) showed separation of PMNs, Mο/MΦ and microglia, consistent with distinct molecular signatures defining these three myeloid cell types after stroke (Fig. 3g). Changes in gene expression (false discovery rate (FDR) < 0.05, absolute fold change ≥2) were significant in PMNs, where 815 genes were differentially regulated; of these, 678 genes (83.19%) were upregulated and 137 genes (16.8%) were downregulated in Trem1−/− versus Trem1+/+ PMNs (Fig. 3h). In contrast, nine genes were differentially regulated in macrophages (FDR < 0.05, absolute fold change > 2; Supplementary Fig. 3e) and none in microglia (FDR < 0.05, any fold change). These differences are consistent with cell-specific levels of expression of TREM1 (Fig. 3b) which are maximal in PMNs, intermediate in macrophages and very low in microglia.
Differentially expressed genes in PMNs (ConsensusPathDB35, FDR < 0.05, absolute fold change > 2) were highly enriched in Kyoto Encyclopedia of Genes and Genomes (KEGG) pathways for antioxidant defense (KEGG: glutathione metabolism, or GSH), immune clearance and degradation (KEGG: lysosome) and protein synthesis (KEGG: ribosome and protein processing in endoplasmic reticulum; Fig. 3i,,jj and Supplementary Fig. 3c). Separate experiments in primary peritoneal macrophages demonstrated a trend towards increased GSH/GSSG ratios in Trem1−/− macrophages (Fig. 3k). TREM1 deficiency also increased lysosomal gene transcription (Supplementary Fig. 3d), suggesting enhanced lysosomal clearance of ischemic debris.
Trem2 was among the most differentially expressed genes identified in Trem1−/− PMNs versus wild-type PMNs (11.2-fold increase, P < 0.0001; Fig. 3l). Trem2 encodes the related TREM2 immune receptor that functionally opposes TREM1 (ref.16). Recent studies have highlighted a protective role of microglial TREM2 in neurodegenerative diseases36–38 and cerebral ischemia39. TREM2 signaling in peripheral myeloid cells is associated with cell survival and proliferation, chemotaxis, anti-inflammatory polarization and homeostasis38–42. In separate experiments, we observed a reciprocal regulation of Trem1 and Trem2 transcripts in RAW macrophages and BV-2 microglia in response to stimulation with lipopolysaccharide (LPS) (Supplementary Fig. 3g,h), suggesting that following acute immune stimulation, TREM1 and TREM2 expression are inversely regulated. Indeed, at 2 d after MCAo, surface expression of TREM2 increased in Trem1−/− CD11b+CD45hi myeloid cells (Fig. 3m and Supplementary Fig. 3f). The percentages of TREM2+ PMNs, Mο/MΦ and microglia increased by 8.8-, 12.5- and 65.5-fold, respectively, in Trem1−/− compared with Trem1+/+ ischemic hemisphere (Fig. 3n). Myeloid cell infiltration in wild-type, Trem1+/− and Trem1−/− ischemic hemispheres was associated with a gene dose-dependent increase in infiltrating Mο/MΦ (Supplementary Fig. 3i,j), potentially related to TREM2 effects on cell survival or chemotaxis. Thus, TREM1 deficiency in the ischemic hemisphere elicited cerebroprotection through antioxidant glutathione metabolism and effects on anti-inflammatory TREM2 and lysosomal pathways.
TREM1 decoy peptide reduces cerebral injury.
The early peripheral induction of TREM1 suggested that targeting of TREM1 systemically might disrupt amplification of peripheral immune responses and reduce stroke injury. Accordingly, we tested whether the decoy peptide LP17, a 17-mer peptide sequence of TREM1 in the complementarity-determining region 3 (ref.43), might attenuate TREM1 immune amplification. The LP17 sequence was previously identified as a highly conserved region of the extracellular domain of TREM1 across multiple species, including rats, mice and humans44. LP17 suppresses the proinflammatory cascade triggered in sepsis and reduces morbidity and mortality when compared with scrambled peptide23,43,44. LP17 or scrambled peptides administered after MCAo at the time of reperfusion (Fig. 4a,,bb and Supplementary Fig. 4a) significantly improved neurological scores, infarct volume and survival, similarly to effects seen in Trem1−/− mice. Control experiments testing the effect of LP17 in Trem1−/− mice did not show differences in neurological scores or infarct volume (Supplementary Fig. 4c,d).

Mice underwent MCAo and were administered LP17 or scrambled peptides either at reperfusion (t = 0 h) or 4.5 h (t = 4.5 h) after reperfusion. a, Neurological scores from WT mice administered LP17 at reperfusion (biologically independent samples per group, n = 16 mice+ scrambled peptide and n = 18 mice+ LP17, mean ± s.e.m.; two-way ANOVA, repeated measures, effect of treatment P = 0.0168, effect of time P < 0.0001; Bonferroni multiple comparisons *P = 0.039 for LP17 versus scrambled peptide at Day 2). b, Infarct volume of WT mice administered LP17 at reperfusion (biologically independent samples per group, n = 6 mice+ scrambled peptide (scr) and n = 9 mice+ LP17, mean ± s.e.m.; Student’s two-tailed unpaired t-test, **P = 0.0014). See Supplementary Fig. 4a,c,d. c, Neurological scores from WT mice administered LP17 at 4.5 h (biologically independent samples per group, n = 12 mice+ LP17 and n = 14 mice+ scrambled peptide, mean ± s.e.m.; two-way ANOVA, repeated measures, effect of LP17 P = 0.0375, effect of time P < 0.0001, effect of interaction P = 0.024; Bonferroni multiple comparisons **P = 0.0096 scrambled versus LP17 peptides at Day 2). See Supplementary Fig. 4b. d, LP17 administered 4.5 h after reperfusion reduces infarct volume (biologically independent samples per group, n = 12 mice+ scrambled peptide and n = 7 mice+ LP17, mean ± s.e.m.; Student’s two-tailed unpaired t-test, **P = 0.0026). e, Representative plots of CD11b+CD45+ and CD11b+CD45+Ly6G+ myeloid cells in IL ischemic hemisphere, 2 d after MCAo; scrambled peptide (top panels); LP17 (bottom panels) administered at time of reperfusion. f, Percentage of brain-infiltrating CD11b+CD45hi myeloid cells in LP17-treated mice in IL and CL hemispheres (biologically independent samples, n = 8 mice+ scrambled peptide and n = 14 mice+ LP17 at reperfusion, mean ± s.e.m.; two-way ANOVA effect of treatment P = 0.0094; Bonferroni multiple comparisons **P < 0.01 for LP17 versus scrambled peptides). g, Percentages of PMNs and Mo/MΦ in LP17-treated mice in IL and CL hemispheres (n = 8–14 biologically independent samples per group, mean ± s.e.m.; two-way ANOVA, effect of treatment in PMNs and Mo/MΦ P < 0.05; effect of treatment in microglia P < 0.01; Bonferroni multiple comparisons *P < 0.05 and **P < 0.01 for LP17 versus scrambled peptides; ****P < 0.0001 for IL versus CL hemisphere for microglia). h, Representative histogram of TREM1 expression in CD11b+CD45+ myeloid cells 2 d after MCAo following administration of LP17 or scrambled peptide at time of reperfusion. i, Percentage of TREM1+-expressing CD11b+CD45+ myeloid cells in LP17-treated mice in IL and CL hemispheres (n = 8–14 biologically independent samples per group, mean ± s.e.m.; two-way ANOVA effect of LP17 P = 0.0065; Bonferroni multiple comparisons ***P < 0.001 for LP17 versus scrambled peptide). j, Numbers of TREM1+ PMNs and Mo/MΦ in LP17-treated mice in IL and CL hemispheres (n = 7–14 biologically independent samples per group, mean ± s.e.m.; Student’s two-tailed unpaired t-test *P < 0.05). k, TREM2 MFI in PMNs and Mo/MΦ 2 d after MCAo in mice ± LP17 or scrambled control peptide at time of reperfusion (n = 7–14 biologically independent samples per group, mean ± s.e.m.; Student’s two-tailed unpaired t-test *P < 0.05). See Supplementary Fig. 4e. l, Mice were administered LP17 or scrambled peptide at 4.5, 18, 26 and 48 h after reperfusion and neuroscores were assessed for 5 d (n = 14 scrambled peptide and n = 15 LP17, mean ± s.e.m.; two-way ANOVA, repeated measures, effect of LP17 P = 0.0064; Bonferroni post-hoc *P < 0.05 and **P < 0.01). m, Latency of beam crossing for mice treated with either LP17 or scrambled peptide at four time points (4.5, 18, 26 and 48 h after MCAo; biologically independent samples, n = 5 sham, n = 8 scrambled peptide, n = 11 LP17, mean ± s.e.m.; repeated measures two-way ANOVA, effect of LP17 biologically independent samples P = 0.006, effect of time P < 0.0001; post-hoc: *P < 0.05 for LP17 versus scrambled peptide on Day 7; #P < 0.0001 for sham versus MCAo+ scrambled peptide; †P < 0.05 for sham versus MCAo+ LP17). n, Number of paw slips in beam-tested mice for sham, LP17 and scrambled peptide-treated mice for right front and hind paws (n = 5 sham, n = 8 scrambled peptide, n = 11 LP17, mean ± s.e.m.; repeated measures two-way ANOVA for LP17 versus scrambled in front paw, effect of LP17 and effect of time, P < 0.05; for rear paw, effect of LP17 P < 0.001 and effect of time P < 0.05; posthoc: **P < 0.01 and ****P < 0.0001 for MCAo+ LP17 versus MCAo+ scrambled peptide; #P < 0.01 for sham versus MCAo+ scrambled peptide). See Supplementary Fig. 4f,g.
Since an increase in peripheral TREM1 surface expression was observed at 4.5 h after MCAo in blood and spleen, we next administered LP17 or scrambled peptide at 4.5 h after MCAo. Neurological scores and infarct volume again improved (Fig. 4c,,d),d), but there was no beneficial effect on survival (Supplementary Fig. 4b), suggesting that a large component of the mortality observed in this model arises from activation of TREM1-dependent innate immune responses initiated hours after MCAo.
We examined the effect of LP17 on trafficking of peripheral myeloid cells to the ischemic hemisphere. Administration of LP17 at the time of reperfusion reduced brain-infiltrating CD11b+CD45hi myeloid cells (Fig. 4e,,f)f) but led to a reciprocal increase in microglia (Fig. 4g). Proportions of CD11b+CD45hi cells expressing TREM1 in the ischemic hemisphere also declined following LP17 treatment (Fig. 4h–j). In line with reduced TREM1 signaling, we observed a reciprocal increase in TREM2 surface expression in both PMNs and Mo/MΦ (Fig. 4k and Supplementary Fig. 4e), similar to the inverse relationship observed in Trem1−/− mice.
Longer-term motor outcomes were assessed in mice administered four doses of LP17 or scrambled peptide at 4.5, 18, 26 and 48 h after MCAo. Neurological scores significantly improved after MCAo in LP17-treated mice (Fig. 4l and Supplementary Fig. 4f). At one week after MCAo, beam-walking tests to assess coordination and integration of motor movements showed better performance in LP17-treated mice versus scrambled peptide, with both LP17- and scrambled peptide-treated mice returning to baseline by 2 weeks (Fig. 4m,,nn and Supplementary Fig. 4g). Thus, suppression of TREM1 activation improved both short- and longer-term function and was associated with a more benign myeloid cell infiltration.
Positron electron tomography (PET) reveals intestinal TREM1 induction after stroke.
To investigate the in vivo extent of TREM1-mediated immune activation, we performed non-invasive molecular imaging of TREM1 using whole-body PET/computed tomography (CT) imaging of MCAo versus sham mice. A TREM1-specific monoclonal antibody (mAb) was radiolabeled with 64Cu for use as a PET tracer. In vitro binding studies confirmed specificity of [64Cu]TREM1-mAb using HEK293 cells transfected with TREM1 complementary DNA (Supplementary Fig. 5a). [64Cu]TREM1-mAb displayed 24-fold higher binding to TREM1-transfected versus untransfected cells. Blocking studies using unlabeled mAb to TREM1 significantly reduced [64Cu]TREM1-mAb binding to transfected cells.
We then examined the distribution of [64Cu]TREM1-mAb in vivo after either sham surgery or MCAo (Fig. 5a–d and Supplementary Video 1). PET tracer was injected 12 h after MCAo, and mice were imaged 24 h later, corresponding to 36 h after MCAo. PET imaging showed significantly higher uptake in the ipsilateral infarcted cortex and striatum compared to the contralateral hemisphere. The ratio of ipsilateral cortex-to-contralateral cortex at 24 h was 1.36 ± 0.12. The ratio of ipsilateral striatum-to-contralateral cortex at 24 h was 1.44 ± 0.16. We also observed significantly increased PET signal in the spleen and also in intestine, compared with sham mice and MCAo mice imaged with [64Cu]isotype-control (Fig. 5c,,d).d). The [64Cu]TREM1-mAb PET signal was not significantly elevated in other peripheral organs, including bone marrow, liver, heart and lung (Supplementary Fig. 5b).
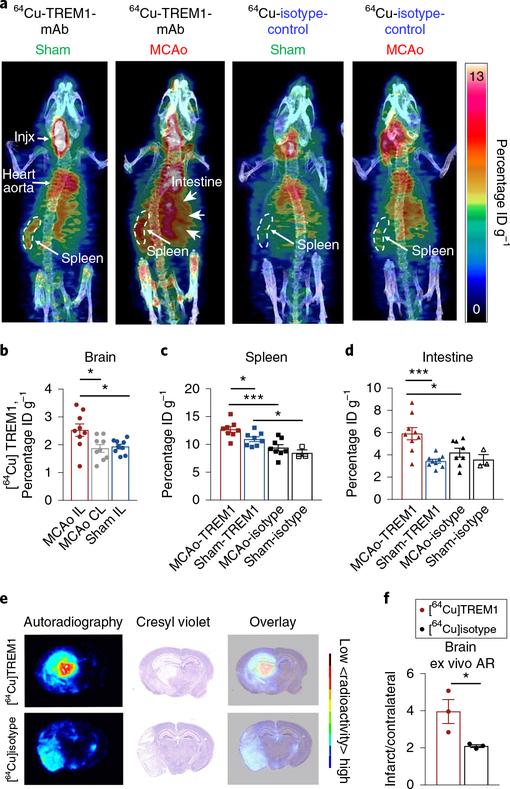
a, Three-dimensional sagittal maximum intensity projection PET/CT images of representative sham and MCAo mice, 36 h after MCAo, injected with either [64Cu]TREM1-mAb or [64Cu]sotype-control-mAb at 12 h after MCAo. b, Quantification of in vivo PET signal in brain 36 h after MCAo (n = 9 biologically independent samples per group, mean ± s.e.m.; Student’s two-tailed t-test, *P < 0.05). c, Quantification of in vivo PET signal in spleen (n = 9 biologically independent samples per group, mean ± s.e.m. except for sham, where n = 3; Student’s two-tailed unpaired t-test, *P < 0.05; ***P < 0.001). d, Quantification of in vivo PET signal in intestine (n = 9 biologically independent samples per group, mean ± s.e.m. except for sham, where n = 3; Student’s two-tailed t-test, *P < 0.05; ***P < 0.001). e, Representative autoradiography images of coronal brain sections, cresyl violet staining and overlay of autoradiography and cresyl staining from mice imaged with [64Cu]TREM1-mAb or [64Cu]isotype-control-mAb 36 h after MCAo. f, Quantification of ex vivo brain autoradiography (AR) (n = 9–10 biologically independent samples per group, mean ± s.e.m.; twotailed Student’s unpaired t-test, *P < 0.05).
In vivo PET findings were then validated with ex vivo gamma counting measurements (Supplementary Fig. 5c–e). MCAo mice injected with [64Cu]TREM1-mAb showed significantly higher tracer uptake within the spleen and brain compared to both sham mice and MCAo mice injected with [64Cu]isotype-control. In addition, ex vivo autoradiography images of brain sections showed higher tracer binding within the infarct area of mice injected with [64Cu]TREM1-mAb compared with those injected with [64Cu]isotype-control (Fig. 5e,,f).f). Taken together, these findings demonstrate a marked increase in the TREM1 PET signal after cerebral ischemia in the brain and in the spleen, in line with flow cytometry findings, but also in the intestine.
Effects of TREM1 induction in intestinal Mo/MΦ subsets.
The lamina propria of the intestine contains a large reservoir of macrophages that maintains an intact gut epithelial barrier and an anti-inflammatory homeostatic microenvironment45. Recent studies have determined that after stroke, sympathetic inputs to the intestine increase gut permeability, leading to translocation of microbial components that contribute to late, post-stroke bacterial infections46. We reasoned that the observed increase in TREM1 PET signal may reflect an induction of TREM1 in response to pathogen-associated molecular pattern molecules (PAMPs) translocating across the intestinal barrier after MCAo. Accordingly, small intestine myeloid cells were examined for TREM1 expression 4.5 h after MCAo, a time point coinciding with increased gut permeability46 and increased TREM1 expression in blood and spleen Mo/MΦ (see Fig. 2). TREM1 MFI increased significantly in CD11b+CD45+ intestinal myeloid cells (Fig. 6a,,bb). CD11b+CD45+ Mo/MΦ cells were gated on the basis of Ly6C and MHCII surface expression to distinguish Mo/MΦ subsets, including Ly6ChiMHCII− extravasated blood monocytes (P1), Ly6CintMHCII+ inflammatory Mo/MΦ (P2) and Ly6CloMHCII+ resident MΦ (P3)45 (Fig. 6c). The inflammatory P2 Mo/MΦ subset selectively expressed TREM1 after MCAo (Fig. 6d), with homeostatic resident P3 MΦs remaining TREM1-negative.

Propranolol or vehicle was administered at reperfusion and 4 h after reperfusion, and Mo/MΦ subsets were examined in small intestine, spleen and blood at 4.5 h after reperfusion. a, Gating strategy to identify CD11b+CD45+ myeloid cells, CD11b+CD45+Ly6G− Mo/MΦ and CD11b+CD45+Ly6G+ neutrophils in small intestine lamina propria. b, TREM1 MFI increases in the CD45+CD11b+ myeloid cell population following MCAo compared to sham control (n = 5 sham and n = 8 biologically independent samples per group, mean ± s.e.m.; Student’s unpaired two-tailed t-test *P < 0.05). c, Representative plot of lamina propria Mo/MΦ subsets after MCAo, demonstrating presence of Ly6C+MHCII− monocytes (P1), Ly6CintMHCII+ inflammatory Mo/MΦ (P2) and Ly6C−MHCII+ resident MΦ (P3). See Supplementary Fig. 6a. d, TREM1 is expressed on the Ly6CintMHCII+ P2 inflammatory Mo/MΦ subset after MCAo. See Supplementary Fig. 6b. e, Effect of ß-adrenergic inhibition with propranolol (ppl) on TREM1 expression in P2 subset of Mo/MΦ after MCAo. See Supplementary Fig. 6c,d. f, Quantification of TREM1 MFI in P3 resident and P2 inflammatory Mo/MΦ subsets in sham, MCAo and MCAo mice ± propranolol (n = 5 sham, n = 8 MCAo and n = 9–10 MCAo+ ppl, mean ± s.e.m.; Student’s two-tailed unpaired t-test; *P < 0.05). g, Representative histogram of TREM1 expression in blood CD11b+CD45+Ly6G− Mo/MΦ from sham mice and MCAo mice ± propranolol. h, Quantification of TREM1 MFI in blood Mo/MΦ from sham mice and MCAo mice ± propranolol (n = 5 sham, n = 9 MCAo and n = 12 MCAo+ ppl, mean ± s.e.m.; Student’s two-tailed unpaired t-test; *P < 0.05). i, Quantification of TREM1 MFI in splenic Mo/MΦ from sham mice and MCAo mice ± propranolol (n = 5 sham, n = 10 MCAo and n = 12 MCAo+ ppl, mean ± s.e.m.; Student’s two-tailed unpaired t-test; *P < 0.05). j, Quantification of plasma IL-6, IL-22, IL-31 and eotaxin from sham mice and MCAo mice ± propranolol (biologically independent samples, sham, n = 3; MCAo+ vehicle, n = 5; MCAo+ ppl, n = 9, mean ± s.e.m.; two-tailed unpaired t-test; *P < 0.05 **P < 0.01).
To examine whether TREM1 was induced in response to sympathetic-driven increases in gut permeability and subsequent translocation of bacterial components, we administered the β-adrenergic inhibitor propranolol at the time of reperfusion and again 4 h later, and assayed TREM1 expression in small intestine Mo/MΦ at 4.5 h after MCAo. β-Adrenergic blockade decreased TREM1 expression in P2 Ly6CintMHCII+ Mo/MΦ but not in P3-resident MΦ or neutrophils (Fig. 6e,,ff and Supplementary Fig. 6a,b). Interestingly, reduction in gut permeability with propranolol also decreased TREM1 induction peripherally in blood and splenic Mo/MΦ (Fig. 6g–i), along with proinflammatory cytokines (Fig. 6j). These findings indicate that TREM1 is induced early after MCAo in intestinal and peripheral Mo/MΦ subsets, in response to sympathetically driven increases in gut permeability and PAMP translocation.
Since TREM1 is an amplifier of innate immune responses via interaction with other innate immune receptors, we asked whether TREM1 activation in the lamina propria might further aggravate the already compromised intestinal barrier. We assessed gut permeability with oral administration of fluorescein isothiocyanate-labeled dextran (FITC-dextran) and measurement of serum FITC-dextran concentrations at 4.5 h after MCAo (Fig. 7a,,b).b). Compared to sham mice, FITC-dextran serum levels increased in wild-type mice administered scrambled peptide after MCAo, but were markedly reduced in LP17-treated mice (Fig. 7a) and Trem1−/− mice (Fig. 7b), indicating that intestinal TREM1 activation further increases gut permeability after MCAo. In addition, the abundance of serum LPS increased in MCAo mice at 4.5 h compared to sham, but was reduced in LP17-treated mice (Fig. 7c) and in Trem1−/− mice (Fig. 7d). Morphometric examination of lamina propria from duodenum, jejunum and ileum at 4.5 h after MCAo revealed marked decreases in the width of the muscularis layer and crypt height in Trem1+/+ mice compared with Trem1−/− mice (Fig. 7e,,f). Expressionf). Expression of the epithelial cell adhesion molecule (EpCAM) was reduced in wild-type mice 4.5 h after MCAo, but preserved in Trem1−/− mice (Fig. 7g,,h). Deletionh). Deletion of TREM1 was also associated with changes in abundance of IL-27 and chemokines MIP-1α and RANTES (Supplementary Fig. 6d). TREM1 deficiency also lowered numbers of colony-forming units in spleen collected at 4.5 h after MCAo (Fig. 7i,,jj and Supplementary Fig. 6e), suggesting that TREM1 activation significantly increases gut permeability and bacterial translocation after stroke. Thus, TREM1 aggravates the post-stroke innate immune response in two temporally and spatially distinct ways: early after stroke, TREM1 amplifies local intestinal myeloid responses to PAMPs and later, with the infiltration of myeloid cells into ischemic brain, TREM1 amplifies responses to sterile brain DAMPs (model in Supplementary Fig. 7).
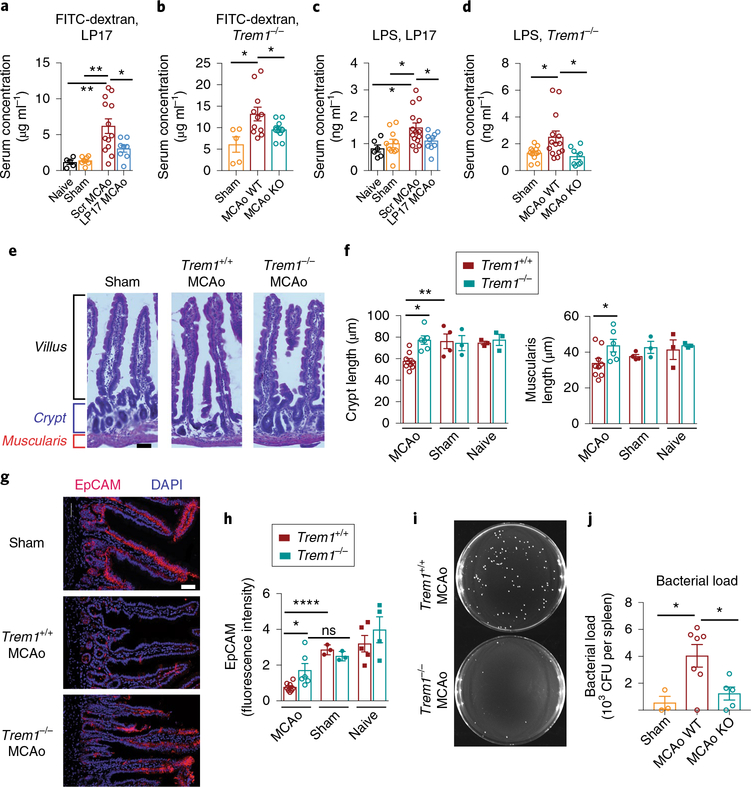
a, Serum concentrations of FITCdextran 4.5 h after MCAo in mice administered scrambled or LP17 peptides at time of reperfusion (n = 5 naïve, n = 7 sham, n = 13 MCAo+ scrambled peptide, n = 8 MCAo+ LP17, mean ± s.e.m.; Student’s two-tailed t-test **P < 0.01). b, Serum concentrations of FITC-dextran 4.5 h after MCAo in Trem1+/+ and Trem1−/− mice (n = 5 sham Trem1+/+, n = 11 Trem1+/+ MCAo and n = 11 Trem1−/− MCAo, mean ± s.e.m.; Student’s two-tailed unpaired t-test *P < 0.05). c, Plasma concentrations of LPS 4.5 h after MCAo in mice ± LP17 or scrambled peptides (n = 6 naïve, n = 10 sham, n = 15 MCAo+ scrambled and n = 10 MCAo+ LP17, mean ± s.e.m.; Student’s two-tailed t-test *P < 0.05). d, Plasma concentrations of LPS 4.5 h after MCAo in Trem1+/+ and Trem1−/− mice (n = 12 sham Trem1+/+, n = 14 Trem1+/+ MCAo and n = 8 Trem1−/− MCAo, mean ± s.e.m.; Student’s two-tailed unpaired t-test *P < 0.05). e, Representative H&E-stained sections of small intestine lamina propria from Trem1+/+ and Trem1−/− mice 4.5 h after MCAo showing villus, crypt and muscularis structures. f, Quantification of muscularis and crypt lengths 4.5 h after MCAo (n = 3–9 biologically independent samples per group, mean ± s.e.m.; Student’s twotailed unpaired t-test *P < 0.05, **P < 0.01). g, Representative confocal images of EpCAM staining in lamina propria 4.5 h after MCAo, scale bar, 50 μm. h, Quantification of EpCAM expression 4.5 h after MCAo (n = 3–9 per group; Student’s two-tailed unpaired t-test; *P < 0.05 and ****P < 0.0001; ns, not significant). i, Representative agar plates from Trem1+/+ and Trem1−/− spleen tissue 4.5 h after MCAo, homogenized (see Methods), plated and incubated for 48 h. j, Quantification of bacterial colonies (CFU, colony-forming units) that grew from splenic lysates from Trem1+/+ and Trem1−/− mice (n = 3 sham, n = 7 MCAo Trem1+/+ and n = 5 MCAo Trem1−/− mice per group, mean ± s.e.m.; Student’s two-tailed unpaired t-test *P < 0.05).
Discussion
In this study, we identify TREM1 amplification of peripheral myeloid immune responses as a major contributor to stroke injury. TREM1 surface expression was induced in Mo/MΦ subsets in the spleen and blood within hours of cerebral ischemia; together with neutrophils expressing high levels of TREM1, these myeloid cells accumulated in ischemic brain and increased cerebral injury and motor deficits. Genetic ablation of TREM1 or blockade with a decoy peptide improved outcome after stroke, providing proof of concept that this unique immune signaling pathway can drive a substantial component of brain injury in this model. Transcriptomics of brain-infiltrating myeloid cells demonstrated that TREM1 suppresses functions essential for brain recovery, including antioxidant glutathione metabolism, anti-inflammatory TREM2 signaling and lysosomal degradative function. Whole-body PET imaging of MCAo mice revealed induction of TREM1 in brain and spleen, but also in intestine. Recent studies have identified a breach of the intestinal barrier with translocation of microbial components occurring in response to sympathetic overactivity after cerebral ischemia46,47. Indeed, we found that, not only was TREM1 induced in lamina propria inflammatory Mo/MΦ subsets, but also activation of TREM1 exacerbated gut permeability and translocation of LPS and bacteria to the periphery. These findings support the concept that after stroke a dual and synergistic innate immune response is initiated early on to intestinal microbial components and later on to brain DAMPs, resulting in an enhanced and toxic innate immune response that worsens cerebral injury.
In Cx3cr1GFPCcr2RFP mice, TREM1 was expressed in the ischemic brain on RFP+ cells, consistent with a peripheral myeloid induction of TREM1. PMNs exhibited robust CCR2-driven RFP expression, consistent with induction of CCR2 in other models of immune activation48,49. Infiltrating PMNs expressed the highest levels of TREM1 under homeostatic and post-stroke conditions, unlike Mo/MΦs, where a marked induction occurred after MCAo. Several endogenous ligands for TREM1 have been proposed, including proteoglycan recognition protein 1, a protein found in neutrophils50, as well as oxidized lipids and bacterial wall-derived proteins and carbohydrates21,51. Genetic ablation of Trem1 induced a widespread induction of gene expression subserving essential cellular functions, including antioxidant defenses, protein translation and processing, lysosomal degradation and other critical metabolic functions. The preservation of these pathways in Trem1−/− neutrophils suggests that activation of this immune amplifier is highly detrimental to overall myeloid cell metabolism and function.
PET imaging of a highly specific radiolabeled TREM1 antibody confirmed the induction of TREM1 in spleen detected by flow cytometry, but also revealed induction of TREM1 in another major lymphoid organ, the intestine. Recent studies demonstrate that disruptions in gut permeability, occurring in response to activation of sympathetic inputs after cerebral ischemia, lead to early systemic dissemination of commensal gut bacteria46,47. In the current study, we cannot exclude the possibility that increased gut permeability may also result from sympathetic vasoconstriction and mesenteric hypoperfusion. In either case, the activation of TREM1 on P2 Ly6CintMHCII+ inflammatory Mo/MΦs51 may represent a host defense to a weakened gut barrier, having the unwelcome effect of worsening gut permeability after MCAo.
The preservation of the lamina propria in Trem1−/− mice may be relevant to the increased survival of Trem1−/− and LP17-treated mice and would argue against a primary role of TREM1 in countering microbial-mediated infection and mortality. It is possible, as in the case of sepsis21, that TREM1 activation increases mortality after MCAo by amplification of immune responses to microbial translocated products, as loss or blockade of TREM1 both improve survival. It remains to be determined what proportion of the innate immune response following cerebral ischemia is driven by translocation of bacterial antigens across the gut barrier versus the immune response to sterile DAMPs released from ischemic brain. The studies using propranolol may be helpful in teasing apart these two contributions. With ß-adrenergic blockade, the reduction of gut permeability significantly lowered TREM1 induction in peripheral blood macrophages. This suggests that induction of peripheral TREM1 occurs in part as a consequence of gut barrier disruption. Moreover, we observed a marked induction in TREM1 MFI as myeloid cells migrated from the periphery into brain, indicating that ischemic brain significantly induces TREM1 expression as well.
The use of PET to detect TREM1 expression highlights the clinical potential of TREM1 PET imaging to detect and track both systemic and brain innate immune activation and to monitor the timing, localization and degree of myeloid-driven inflammation after stroke. The most widely studied PET ligands for tracking inflammation are those that target the translocator protein 18 kDa (TSPO), which is localized on the outer mitochondrial membrane in several cell types, including endothelium, immune cells and astrocytes, where it functions in maintaining mitochondrial homeostasis52. TSPO is upregulated under diverse inflammatory conditions and has been used as a marker of inflammation for PET imaging studies53,54, but no biological connection has emerged between TSPO induction and toxic innate immune responses. Here, the biological significance of TREM1 induction, coupled with its expression on myeloid lineage cells, may represent a new approach to non-invasively detect and follow injurious inflammation in living subjects after cerebral ischemia.
In conclusion, we identify a highly detrimental component of the post-stroke innate immune response, driven by peripheral TREM1+ myeloid cells. From a translational perspective, the post-stroke immune response offers an extended window for therapeutic intervention, and strategies targeting TREM1 could be used alone or in combination with thrombolytic/endovascular interventions, whose time windows are more limited. In addition, the early induction of TREM1 in intestine and its role in promoting bacterial translocation are highly relevant to the occurrence of post-stroke bacterial infections that are associated with high morbidity and mortality in stroke patients. Our findings suggest that inhibition of TREM1 after stroke will blunt injurious immune responses, allowing beneficial myeloid responses—including TREM2 responses—to prevail, accelerating recovery and regeneration. Finally, TREM1 actions poststroke may be relevant to other acute central nervous system insults that are similarly characterized by a post-injury immune response, including traumatic brain injury and spinal cord injury.
Methods
Animals.
This study was conducted in accordance with the National Institutes of Health guidelines for the use of experimental animals and protocols were approved by the Institutional Animal Care and Use Committee. Wild-type C57BL/6J, Cx3cr1-GFP (B6.129P(Cg)-Ptprca Cx3cr1tm1Litt/LittJ) and Ccr2-RFP (B6.129(Cg)-Ccr2tm2.1Ifc/J) mice were purchased from Jackson Laboratories. Trem1−/− mice in the C57BL/6 background have been previously described24. All mice were housed in an environment controlled for lighting (12 h light/dark cycle), temperature and humidity, with food and water available ad libitum.
Transient focal ischemia model.
All middle cerebral artery occlusion-reperfusion (abbreviated MCAo) experiments were performed by an experimenter blinded to genotype or pharmacological treatment as described previously55,56. The 8–12-week-old male C57BL/6J mice were randomized and subjected to either sham surgery or 45 min of MCA occlusion followed by reperfusion, with survival up to 14 d. Neuroscores were assessed as follows: 0, no deficit; 1, forelimb weakness and torso turning to the ipsilateral side when held by the tail; 2, circling to affected side; 3, unable to bear weight on affected side; 4, no spontaneous locomotor activity or barrel rolling, as described in ref.57.
Quantification of infarct volume.
Measurement of infarct volume was carried out by an examiner blinded to genotype or treatment. Mice were lethally anesthetized and brain tissue was collected for infarct quantification using cresyl violet, as previously described56. For each brain, 20-μm sections at 800-μm intervals were stained with cresyl violet and imaged (Keyence microscope, BZ-9000 Series) and the unstained infarct area was quantified using ImageJ (http://imagej.nih.gov/ij). To minimize the contributions of cytotoxic and vasogenic edema, the infarct volume was determined using the indirect method58.
Isolation of immune cells for flow cytometry.
Mice were anesthetized with isoflurane and transcardially perfused with 0.9% saline. Brain hemispheres were homogenized in 10 ml Hank’s balanced salt solution (HBSS, ThermoFisher) filtered through a 70-μm cell strainer and centrifuged at 250g for 5 min at 4 °C. Myelin removal was carried out by incubating with collagenase IV (1 mg ml−1, Worthington) in HBSS for 30 min at 37 °C and 200 r.p.m., centrifugation at 250g for 5 min at 4 °C and addition of 5 ml of 30% Percoll (Sigma) in 0.1 M sodium-potassium PBS and density-gradient centrifugation. Spleens were manually homogenized in 5 ml 0.1 M PBS and filtered through a 70-μm cell strainer. Splenic cells were concentrated with centrifugation at 250g for 5 min at 4 °C and incubated in 3 ml red blood cell lysis (1:10, Biolegend) for 5 min on ice. After incubation, both splenic and blood cells were concentrated by centrifugation at 200g for 5 min at 4 °C. Blood cells were resuspended in 3 ml 0.1 M PBS and splenocytes were suspended in 200 μl 0.1 M PBS. Dead cells were identified using LIVE/DEAD Fixable Dead Cell Stain Kit (ThermoFisher Scientific).
Flow cytometry analysis.
Approximately 106 cells were suspended in 200 μl HBSS buffer. Fc receptor binding was blocked with 100 μl solution of anti-mouse CD16/CD32 (5 ng μl−1, BioLegend, clone 93) in PBS for 10 min at 4 °C followed by staining with 100 μl solution of antibodies for 15 min at 4 °C. The following antibodies were used for surface receptor detection: CD45 (1 ng μl−1, Biolegend, clone 30F11), CD11b (1 ng μl−1, Biolegend, clone M1/70), Ly6G (1 ng μl−1, Biolegend, clone 1A8), TREM1 (2.5 ng μl−1, R&D, clone 174031) and isotype control (rat IgG2A phycoerithrin (PE)-conjugated antibody, R&D, clone 54447). Cells were washed with HBSS buffer, resuspended in 200 μl of HBSS buffer and analyzed with a LSR II cytometer (BD Biosciences) and analyzed using FlowJo software (Tree Star Inc.). For Cx3cr1GFPCcr2RFP flow cytometry, we applied CD11b PE-Cy7 and TREM1 PE: compensation controls for GFP channel consisted of microglia derived from naïve Cx3cr1GFPCcr2RFP brain; for RFP controls were macrophage/monocytes derived from naïve Cx3cr1GFPCcr2RFP blood.
Fluorescence-activated cell sorting.
Brain hemispheres were manually homogenized in 10 ml HBSS through a 70-μm cell strainer, centrifuged at 200g, 4 °C for 5 min and resuspended in 800 μl 0.5% BSA in HBSS. Myelin depletion (Miltenyi Biotec) was carried out according to the manufacturer’s instructions. Cells were incubated with anti-mouse CD16/32 (5 ng μl−1, BioLegend, clone 93) for 10 min at 4 °C to block Fc receptor binding, and dead cells were identified using LIVE/DEAD Fixable Dead Cell Stain Kit (ThermoFisher Scientific). Cells were stained with antibodies in 100 μl PBS solution for 15 min at 4 °C. The following antibodies were used: CD45 (1 ng μl−1, Biolegend, clone 30F-11), CD11b (1 ng μl−1, Biolegend, clone M1/70) and Ly6G (1 ng μl−1, Biolegend, clone 1A8). Cells were sorted using the BD Aria Fusion II (BD Biosciences) and stored in 300 μl RNA later solution (ThermoFisher Scientific) at 4 °C. Between approximately 15,000 and 50,000 macrophages, neutrophils or microglia per ischemic hemisphere were collected.
RNA isolation and Affymetrix Clariom mouse microarray.
RNA purification was performed using the RNeasy Mini Kit (Qiagen). RNA quality was assessed using a BioAnalyzer (Agilent) and yielded an RNA integrity number >8.0 for all samples. In vitro mRNA transcription, labeling and hybridization were performed by the Stanford Protein and Nucleic Acid Facility using the Clariom S Assay HT platform (Affymetrix). Microarray data were analyzed using the Affymetrix Transcription Analysis Console 4 (TAC 4, Affymetrix) to identify statistically significant (FDR < 0.05) differentially expressed genes. Gene enrichment and pathway analyses were conducted using ConsensusPathDB; gene ontology pathway analysis was conducted using DAVID Bioinformatics Resources v.6.8. Gene expression files have been submitted to GEO as submission GSE105132.
Liquid chromatography–mass spectrometry (LC–MS) detection of GSSG/GSH levels.
Peritoneal macrophages were plated in 6-well plates at a confluency of 1.5 × 106 cells per well. Cells were washed with 1× PBS twice by aspirating media and immediately adding 1 ml of −80 °C 80:20 methanol:water. After 20 min of incubation on dry ice, the resulting mixture was scraped, collected into a centrifuge tube and centrifuged at 10,000g for 5 min at 4 °C. Pellets were then extracted again with 500 μl of −80 °C 80:20 methanol:water and incubated for 5 min then, centrifuged at 10,000g for 5 min at 4 °C. Both extracts were combined into a 1.5-ml microcentrifuge tube and dried under N2. For GSH and GSSG measurement, separate samples were prepared, omitting the drying step, which we observed to result in artifactual GSH oxidation. LC–MS was performed as previously described59. In brief, the LC–MS method involved hydrophilic interaction chromatography (HILIC) coupled to the Q Exactive PLUS mass spectrometer (ThermoFisher Scientific). The LC separation was performed on an XBridge BEH Amide column (150 mm × 2.1 mm, 2.5 μm particle size, Waters). Solvent A was 95%:5% H2O:acetonitrile with 20 mM ammonium bicarbonate, and solvent B was acetonitrile. The gradient was 0 min, 85% B; 2 min, 85% B; 3 min, 80% B; 5 min, 80% B; 6 min, 75% B; 7 min, 75% B; 8 min, 70% B; 9 min, 70% B; 10 min, 50% B; 12 min, 50% B; 13 min, 25% B; 16 min, 25% B; 18 min, 0% B; 23 min, 0% B; 24 min, 85% B; 30 min, 85% B. Other LC parameters were: flow rate 150 μl min−1, column temperature 25 °C, injection volume 5 μl.
Cell culture of BV-2 and RAW264.7 cell lines.
BV-2 or RAW264.7 cells lines were cultured in DMEM supplemented with 10% fetal bovine serum and 4.5 g l−1 glucose, l-glutamine and sodium pyruvate (Corning). One day before LPS treatment, cells were transferred to 12-well plates at 2 × 105 cells per well in 500 μl of culture media. The next day, LPS solutions in culture media (10 ng ml−1) were added to the cells for incubation of 0, 4 or 20 h.
Quantitative real-time PCR.
RNA (Qiagen RNA Purification kits) was treated with DNase (Invitrogen) and reverse transcribed to cDNA (High-Capacity cDNA Reverse Transcription Kit, Applied Biosystems). Following cDNA synthesis, samples were amplified for TREM1 and TREM2 (Taqman, TREM1: Mm01278455_m1; TREM2: Mm04209424_g1, Applied Biosystems) and GAPDH (GAPDH: Mm99999915_g1, Applied Biosystems). Fluorescence was measured using QuantStudio 6 Flex and expression levels calculated using the delta-delta CT (ddCT) method. All experiments were conducted in triplicate.
Synthesis of LP17 and scrambled control peptide.
LP17 and scrambled peptides were synthesized as described in ref.44 by the Protein and Nucleic Acid Core Facility at Stanford University. The peptide sequence for LP17 is LQVTDSGLYRCVIYHPP, and for the scrambled control peptide is TDSRCVIGLYHPPLQVY.
Beam-walking test.
A beam-walking test was used to assess the coordination and integrity of motor movements60–62. The time taken to cross the beam and the number of left and right paw slips were recorded with a video camera and analyzed by an experimenter blinded to the treatment groups. The Plexiglas beam was 100-cm long and 0.5-cm wide and was elevated 50 cm off the floor leading to the motivation box (MB) which was 20 cm (length) x 20 cm (width) x 20 cm (height) and made of black plastic. Mice were given five trials of training to learn to traverse the beam. During the first training trial, the animal was placed 25 cm away from the MB; the second trial began at 50 cm away from the MB; on the third, fourth and fifth trials, the animal was placed at the starting point 100 cm away from the MB. On the testing days, mice were given three trials to traverse the beam. The testing days occurred before MCAo and on Day 7 and Day 14 post-surgery. The duration of beam traversal was defined as when the mice started walking across the beam to when the two front paws entered the MB. A maximum trial duration of 300 s was recorded for mice that failed to reach the MB.
1,4,7,10-Tetraazacyclododecane-1,4,7,10-tetraacetic acid (DOTA) conjugation.
Conjugation of anti-mouse TREM1-mAb and isotype-contol-mAb (R&D) with DOTA was performed according to standard procedures using metal-free buffers. In brief, a solution of DOTA-NHS ester (Macrocyclics Inc.) in dimethyl sulfoxide (25 mmol l−1; 9–12 μl) was added to 1 ml of HEPES buffer (0.1 mol l−1, pH 8.8) containing 500 μg of TREM1-mAb or isotype-control-mAb, and the reaction mixture was incubated at 4 °C overnight. The reaction was quenched with Tris pH 7.4 (Sigma), excess DOTA-NHS was removed by Zeba Spin Desalting Columns (0.5 ml, 70K molecular weight cut-off, ThermoFisher Scientific) and the resulting solution was buffer-exchanged into ammonium acetate buffer (0.1 M, pH 5.5) for 64Cu labeling. DOTA-conjugate solutions were concentrated by ultrafiltration (Vivaspin 2 ml, Sartorius) to 1–3 mg ml−1, snap-frozen in liquid nitrogen and stored at −80 °C before radiolabeling. The number of DOTA chelators coupled per antibody molecule was estimated to be between 2 and 4 for both TREM1 and isotype-control, measured via matrix-assisted laser desorption/ionization–time of flight MS, by comparison with unconjugated mAb versus DOTA-conjugated mAb.
Radiolabeling.
Both DOTA-TREM1-mAb and DOTA-isotype-control-mAb were radiolabeled with 64Cu (t½ = 12.7 h) using standard methods and metal-free buffers, with some modifications. DOTA-TREM1-mAb/DOTA-isotype-control-mAb (100 μg) in 30–50 μl of 0.25 mol l−1 ammonium acetate buffer (0.1 M, pH 5.5) was mixed with pH-balanced 64CuCl2 solution (44–74 MBq, pH 4.5–5.0, University of Wisconsin) at 37 °C with gentle shaking at 400 r.p.m. After a 30–60 min incubation period, 0.1 M EDTA (0.5 M, pH 8.0) was added to a final concentration of 0.01 M and incubated at 22 °C for 15 min to scavenge unchelated 64CuCl2 in the reaction mixture. Purification of each radiolabeled antibody was achieved by G25 Sephadex size-exclusion purification (NAP-5 column). Radiochemical purity was determined by instant thin-layer chromatography with TEC-Control Chromatography strips (Biodex Medical Systems), developed in saline, and size-exclusion liquid chromatography with a Phenomenex SEC 3000 column (Torrance) with sodium phosophate buffer (0.1 mol l−1, pH 6.8) at a flow rate of 1.0 ml min−1. 64Cu-labeled anti-TREM1-mAb (that is, [64Cu]TREM1-mAb) and 64Cu-labeled isotype-controlmAb (that is, [64Cu]ISO-mAb) were obtained with high specific radioactivity (>0.400 MBq μg−1), radiochemical purity (>99%) and labeling efficiency (70–95%) and formulated in phosphate-buffered saline (0.1 mol l−1 NaCl, 0.05 mol l−1 sodium phosphate (pH 7.4)).
In vitro cell-binding study.
HEK293 cells transiently expressing murine Trem1 cDNA or control empty vector in 24-well plates were assayed for binding of [64Cu]TREM1-mAb. One day after transfection, media was aspirated from each well and fresh, pre-warmed DMEM containing 0.19 MBq of [64Cu]TREM1-mAb was added (500 μl per well). Cells were incubated with [64Cu]TREM1-mAb at 37 °C and 5% CO2 for 1 h, washed three times with PBS and then lysed in radio-immunoprecipitation assay buffer (ThermoFisher Scientific Inc.; 250 μl). Cell lysates (150 μl) were transferred to counting tubes and decay-corrected radioactivity was determined on a gamma counter (Cobra II Auto-Gamma counter; Packard Biosciences Co.). The remaining lysate was frozen and used following radioactive decay for protein determination using a bicinchoninic acid 96-well plate assay (ThermoFisher Scientific Inc.). In addition, 10 μl standards from the 0.19 MBq per 500 μl solution added to cells were counted to quantitate the percentage radiotracer uptake. For blocking studies, cells were pre-treated with unlabeled unconjugated TREM1-mAb (×100 mass associated with 0.19 MBq of [64Cu]TREM1-mAb) for 30 min, washed three times with phosphate-buffered saline and then tracer ([64Cu]TREM1-mAb) was added for 1 h incubation.
In vivo magnetic resonance (MR) and PET/CT imaging of MCAo and sham mice.
T2-weighted structural MRI images were acquired 1.0–1.5 d post-MCAo surgery to confirm successful stroke and provide anatomical reference for PET image analysis. Images were acquired using a 7 T MRI Varian Magnex Scientific MR scanner system and a millipede quadrature radiofrequency coil.
MCAo and sham mice were injected with [64Cu]TREM1-mAb (1.31–4.38 MBq) or [64Cu]ISO-mAb (1.59–3.63 MBq) intravenously. PET tracer was injected 12 h after MCAo, and mice were imaged 3 h and 24 h later. PET images acquired at 3 h were not as useful as those acquired at 24 h since antibody–PET tracers have a long blood residence and high levels of unbound tracer in blood can obscure visualization of bound tracer in tissues. An antibody–PET tracer must sufficiently clear from blood before imaging to achieve high signal-to-background images. Mice were then imaged at 19–20 h post intravenous injection. Mice were anesthetized using isoflurane gas (2.0–3.0% for induction and 1.5–2.5% for maintenance). A CT image was acquired immediately before each PET scan. CT raw images were acquired at 80 kVp at 500 μA, two-bed position, half-scan 220° of rotation and 120 projections per bed position with a cone beam micro-X-ray source (50 μm focal spot size) and a 2,048 pixel × 3,072 pixel X-ray detector. On the basis of attenuation correction from the CT measurements, each 10-min static PET scan was acquired with default settings of coincidence, a timing window of 3.4 ns and an energy window of 350–650 keV. PET and CT image files were co-registered and analyzed using Inveon Research Workspace software (IRW, v.4.0; Siemens). PET images were reconstructed with the three-dimensional ordered subsets expectation maximization (OSEM3D) algorithm.
The PET system can deliver ~ 1.5–2.0-mm spatial resolution, and a maximum field of view of 10 cm × 30 cm. OSEM3D/maximum a posteriori (MAP) reconstruction yields uniform spatial resolution in all directions, with an average full width at half maximum of 1.656 ± 0.06 mm. All PET images were reconstructed using two iterations of OSEM3D algorithm (12 subsets) and 18 iterations of the accelerated version of 3D-MAP (that is, FASTMAP)—matrix size of 128 × 128 × 159.
Image analysis.
PET, CT and brain MR image files were co-registered and analyzed with VivoQuant (VQ, v.2.0, inviCRO) and IRW software (v.4.0). Regions of interest (ROIs) were drawn around the infarct using the MR image as a guide and then copied to the contralateral hemisphere using VQ software, while peripheral organ ROIs were drawn using IRW. The mean concentration of radioactivity contained within each ROI (Bq cm−3) was used to calculate percentage injected dose (ID) per g (%ID g−1) values, using the decay-corrected dose for each mouse at the time of the PET scan.
Biodistribution.
Following the final PET scan, mice were deeply anesthetized with 2–2.5% isoflurane. Blood samples (100–200 μl) were collected via cardiac puncture immediately before transcardial perfusion using 20–30 ml of PBS. All mice that underwent PET imaging were euthanized after perfusion with saline to remove possible unbound intravascular [64Cu]TREM1-mAb. Blood, heart, liver, lungs, spleen, left brain and right brain hemispheres were extracted/dissected from each mouse, placed in a tube for gamma counting and weighed. Satisfactory perfusions were verified by visual inspection of brain tissue. Dissected tissues were then counted via an automated gamma counter (Cobra II Auto-Gamma counter; Packard Biosciences Co.) and tissue-associated radioactivity was then normalized to tissue weight and to the amount of radioactivity administered to each mouse, and decay-corrected to time of tracer injection using diluted aliquots of the initial administered dose as standards.
Ex vivo autoradiography.
At 20 h post-injection of radiotracer, n = 3 mice injected with [64Cu]TREM1-mAb (3.34–7.88 MBq) and n = 3 mice injected with [64Cu] isotype-control-mAb (2.90–7.97 MBq) were deeply anesthetized using isoflurane gas (2.0–3.0%) and perfused with 30–50 ml of PBS. Brain tissue was quickly embedded in optimal cutting temperature compound (Tissue-Tek) and coronal sections (20 μm) were obtained for ex vivo autoradiography. Autoradiography was conducted and the anatomy of brain sections was confirmed by Nissl staining (cresyl violet acetate; Sigma Aldrich) using standard techniques. In brief, 20-μmthick sections were mounted on microscope slides (Fisherbrand Superfrost Plus Microscope Slides), air-dried for 10 min and then exposed to a high-resolution digital storage phosphor screen (GE Lifesciences) for 72 h at −20 °C. Ex vivo autoradiography images of brain sections were quantified by drawing ROIs around the infarct using Nissl staining to verify. The digital storage phosphor screen was scanned using a Typhoon 9410 Variable Mode Imager (Amersham Biosciences) and images were analyzed using ImageJ (image processing and analysis software in Java, v.1.45s).
Immune cell isolation from murine intestine.
Intestinal immune cell isolation was carried out as previously described28. Mice were terminally anesthetized and perfused with 0.9% saline and the small intestine was opened longitudinally and cut into small pieces. Epithelial cells were removed by shaking at 200 r.p.m. in HBSS containing 5% FCS, 2 mM EDTA for 15 min twice at 37 °C. After washing with EDTA-free HBSS buffer, lamina propria cells were obtained by digestion with 100 U ml−1 collagenase (type IV, Sigma) and 50 U ml −1DNase (type I, grade II, Roche) in Ca2+- and Mg2+-supplemented HBSS with 5% FCS for 15 min at 37 °C. Cell suspensions were passed through a 70-μM cell strainer for flow cytometry. Live/dead cells were identified using LIVE/DEAD Fixable Dead Cell Stain Kit (ThermoFisher Scientific). Cells were then fixed using 1.6% paraformaldehyde in PBS for 10 min at 22 °C and stored at −80 °C for future use.
Flow cytometry of intestinal immune cells.
Cell suspensions were blocked for the Fc receptor (anti-mouse CD16/CD32, BD Biosciences) and stained with the following antibodies (Biolegend, unless indicated otherwise): anti-CD45-APCCY7, anti-CD11b-PE-CY7, anti-Ly6G-FITC, anti-Ly6C-APC, anti-TREM1-PE and anti-MHCII-PerCP-Cy5.5. Dead cells were excluded using Aqua (ThermoFisher) at a final concentration of 0.5 μg ml−1. All samples were acquired on an LSR II (BD Biosciences) and analyzed using FlowJo software (Tree Star).
Inhibition of β-adrenergic receptors with propranolol.
C57BL/6 male mice (8–10-week-old) underwent MCAo, and propranolol (intraperitoneally at 30 mg per kg (body weight)) or vehicle was administered at time of reperfusion, 45 min after occlusion and at 4 h after reperfusion.
Gut permeability assay.
Gut permeability was assayed at 4.5 h after MCAo by measuring serum levels of FITC-dextran (Sigma)46. FITC-dextran (500 mg kg–1 (body weight)) (28 mg ml−1 in saline) was administered by oral gavage 2 h after initiation of MCAo. Mice were terminally anesthetized at 4.5 h after MCAo, and blood was collected via cardiac puncture and centrifuged at 2,500 r.p.m. for 10 min. Serum samples were stored at −80 °C until use. Fluorescence was read (excitation 485 nm and emission 520 nm) on a Spectramax M5 microplate reader (Molecular Devices). Absolute concentrations of FITC-dextran in serum samples were determined relative to that of the standard curve.
Serum LPS detection.
Mice were terminally anesthetized with isoflurane and blood was collected immediately via cardiac puncture and allowed to clot at 22 °C for 15–30 min. Serum was then separated by centrifuging samples at 1,000g for 10 min at 4 °C. Levels of serum LPS diluted 2.5-fold in PBS were assayed using the LPS ELISA Kit (Mybiosource) and absorbance was detected using the Spectromax M5 microplate reader (Molecular Devices).
Intestinal morphology and immunocytochemistry.
Small intestine was collected at 4.5 h after MCAo from terminally anesthetized mice perfused with 0.9% saline, and 1-cm segments of intestine were collected from the duodenum, jejunum and ileum, fixed in 4% phosphate-buffered formaldehyde for 24 h, immersed in 30% sucrose for 24 h and embedded in optimal cutting temperature compound and stored at −80 °C. Each segment was then cut longitudinally into 10-μmthick sections by cryostat. Sections (10–12) were collected from each segment of intestine, and four sections per segment were stained with haematoxylin and eosin (H&E) and visualized at ×400 on a BZ-X700 Keyence microscope. Regions (7–9 each per mouse) from the duodenum, jejunum and ileum were analyzed morphometrically for villus length, crypt depth and muscularis width using ImageJ. For staining with EpCAM, four sections per intestinal segment were blocked with 10% goat serum and 1% BSA in PBS for 1 h at 22 °C, washed in PBS and incubated with anti-EpCAM (1:200, PE-conjugated rat anti-mouse CD326, Biolegend) in PBS containing 5% goat serum and 0.5% BSA overnight at 4 °C. After incubation, the sections were washed with PBST (0.1% Tween-20 in PBS) for 5 min twice and mounted with VECTASHIELD Antifade Mounting Medium with DAPI (Vector Laboratories). Sections were imaged with the BZ-X700 Keyence microscope and 7–9 images from each intestinal segment were analyzed with ImageJ software.
Bacteriological analysis.
All steps were carried out aseptically using sterilized reagents, instruments and equipment. Terminally anesthetized mice were disinfected with 70% ethanol and blood was collected via cardiac puncture immediately into 20 μl of 0.25 M EDTA. Mice were then perfused with 0.9% saline and the spleen was removed, manually homogenized in 10 ml PBS and filtered through a 70-μm cell strainer. For each mouse, 300 μl of spleen homogenate was plated onto brain heart infusion (BHI) agar plates supplemented with 5% sheep blood in replicate; plates were incubated at 37 °C for 48 h, whereupon bacterial colonies were counted for each plate. To quantify bacterial load in blood, 250 μl of blood were diluted 1:1 in DPBS (Life Technologies, catalog no. 14190–144) and transferred gently onto 1 ml RosetteSep DM-L density medium (Stemcell Technologies, catalog no. 15705). Samples were centrifuged at 350g (~ 1,500 r.p.m.) for 20 min. After centrifugation, the separated plasma layer was transferred to a 1.5-ml microcentrifuge tube and an equal volume of 2% saponin (Sigma Aldrich, catalog no. 47036) was added to each sample and mixed by pipetting; samples were then incubated at 22 °C protected from light for 5 min and centrifuged at 4,000 r.p.m. for 20 min at 22 °C. After centrifugation, the supernatant was gently removed by pipetting, leaving a volume of 300 μl covering the pellet. The pellet was then fully resuspended in the remaining 300 μl of supernatant and plated on BHI agar plates containing 5% sheep blood; plates were incubated at 37 °C with 5% CO2 overnight, whereupon bacterial colonies were counted. Anaerobic bacteria were not analyzed in this study.
Statistical analysis.
Statistical analysis was performed using Student’s unpaired t-test, one-way or two-way analysis of variance (ANOVA), followed by Tukey or Bonferonni post-hoc analysis of biologically independent samples. For behavioral analyses, two-way ANOVA with repeated measures was used, with treatment as the main effect. For analysis of differential gene expression as well as gene ontology and KEGG pathway enrichment analyses, FDR < 0.05 was used. All data are reported as mean ± s.e.m. P values ≤ 0.05 were considered significant.
Reporting Summary.
Further information on research design is available in the Nature Research Reporting Summary linked to this article.
Supplementary Material
Supplementary video
2
Acknowledgements
This work was supported by National Institutes of Health (NIH) grant (nos. R01NS045727, R21NS087639, R01NS100180 and RO1AG053001 to K.I.A.), The Paul and Daisy Soros Fellowship for New Americans (to P.S.M.), the Gerald J. Lieberman Fellowship (to P.S.M.) and Stanford Medicine Dean’s Fellowship (to E.N.W.). The authors would like to thank W. Lu for LC–MS expertise, the Stanford Shared FACS Facility, the Stanford Human Immune Monitoring Center, and the Protein and Nucleic Acid Facility.
Data availability
The data that support the findings of this study are available from the corresponding author upon request.
Footnotes
online content
Any methods, additional references, Nature Research reporting summaries, source data, statements of code and data availability and associated accession codes are available at https://doi.org/10.1038/s41590-019-0421-2.
Competing interest
The authors declare no competing interests.
Additional information
Supplementary information is available for this paper at https://doi.org/10.1038/s41590-019-0421-2.
Peer review information: Laurie Dempsey was the primary editor on this article and managed its editorial process and peer review in collaboration with the rest of the editorial team.
Publisher’s note: Springer Nature remains neutral with regard to jurisdictional claims in published maps and institutional affiliations.
References
Full text links
Read article at publisher's site: https://doi.org/10.1038/s41590-019-0421-2
Read article for free, from open access legal sources, via Unpaywall:
https://europepmc.org/articles/pmc6778967?pdf=render
Citations & impact
Impact metrics
Citations of article over time
Alternative metrics
Article citations
PET imaging of microglia in Alzheimer's disease using copper-64 labeled TREM2 antibodies.
Theranostics, 14(16):6319-6336, 30 Sep 2024
Cited by: 0 articles | PMID: 39431020 | PMCID: PMC11488106
A review of the pathogenesis of epilepsy based on the microbiota-gut-brain-axis theory.
Front Mol Neurosci, 17:1454780, 03 Oct 2024
Cited by: 0 articles | PMID: 39421261 | PMCID: PMC11484502
Review Free full text in Europe PMC
Soluble Triggering Receptors Expressed on Myeloid Cells (sTREM) in Acute Ischemic Stroke: A Potential Pathway of sTREM-1 and sTREM-2 Associated with Disease Severity.
Int J Mol Sci, 25(14):7611, 11 Jul 2024
Cited by: 0 articles | PMID: 39062850 | PMCID: PMC11277504
Loss of Epitranscriptomic Modification N6-Methyladenosine (m6A) Reader YTHDF1 Exacerbates Ischemic Brain Injury in a Sexually Dimorphic Manner.
Transl Stroke Res, 13 Jun 2024
Cited by: 0 articles | PMID: 38869772
Mechanisms of inflammation after ischemic stroke in brain-peripheral crosstalk.
Front Mol Neurosci, 17:1400808, 12 Jun 2024
Cited by: 0 articles | PMID: 38932932 | PMCID: PMC11199882
Review Free full text in Europe PMC
Go to all (76) article citations
Data
Data behind the article
This data has been text mined from the article, or deposited into data resources.
BioStudies: supplemental material and supporting data
GEO - Gene Expression Omnibus
- (1 citation) GEO - GSE105132
Similar Articles
To arrive at the top five similar articles we use a word-weighted algorithm to compare words from the Title and Abstract of each citation.
TREM1-ors shake the brain and gut after stroke.
Nat Immunol, 20(8):950-952, 01 Aug 2019
Cited by: 3 articles | PMID: 31285627
Tracking Innate Immune Activation in a Mouse Model of Parkinson's Disease Using TREM1 and TSPO PET Tracers.
J Nucl Med, 63(10):1570-1578, 17 Feb 2022
Cited by: 8 articles | PMID: 35177426
Transcriptome profiling of brain myeloid cells revealed activation of Itgal, Trem1, and Spp1 in western diet-induced obesity.
J Neuroinflammation, 16(1):169, 19 Aug 2019
Cited by: 21 articles | PMID: 31426806 | PMCID: PMC6700800
TREM1: Activation, signaling, cancer and therapy.
Pharmacol Res, 204:107212, 13 May 2024
Cited by: 2 articles | PMID: 38749377
Review
Funding
Funders who supported this work.
NIA NIH HHS (1)
Grant ID: RF1 AG053001
NINDS NIH HHS (3)
Grant ID: R21 NS087639
Grant ID: R01 NS100180
Grant ID: R01 NS045727