Abstract
Free full text

Heterogeneity in the Molecular Composition of Excitatory Postsynaptic Sites during Development of Hippocampal Neurons in Culture
Abstract
To determine their roles in the assembly of glutamatergic postsynaptic sites, we studied the distributions of NMDA- and AMPA-type glutamate receptors; the NMDA receptor-interacting proteins α-actinin-2, PSD-95, and chapsyn; and the PSD-95-associated protein GKAP during the development of hippocampal neurons in culture. NMDA receptors first formed nonsynaptic proximal dendrite shaft clusters within 2–5 d. AMPA receptors were diffuse at this stage and began to cluster on spines at 9–10 d. NMDA receptor clusters remained partially nonsynaptic and mainly distinct from AMPA receptor clusters until after 3 weeks in culture, when the two began to colocalize at spiny synaptic sites. Thus, the localization of NMDA and AMPA receptors must be regulated by different mechanisms. α-Actinin-2 colocalized with the NMDA receptor only at spiny synaptic clusters, but not at shaft nonsynaptic or synaptic clusters, suggesting a modulatory role in the anchoring of NMDA receptor at spines. PSD-95, chapsyn, and GKAP were present at some, but not all, nonsynaptic NMDA receptor clusters during the first 2 weeks, indicating that none is essential for NMDA receptor cluster formation. When NMDA receptor clusters became synaptic, PSD-95 and GKAP were always present, consistent with an essential function in synaptic localization of NMDA receptors. Furthermore, PSD-95 and GKAP clustered opposite presynaptic terminals several days before either NMDA or AMPA receptors clustered at these presumptive postsynaptic sites. These results suggest that synapse development proceeds by formation of a postsynaptic scaffold containing PSD-95 and GKAP in concert with presynaptic vesicle clustering, followed by regulated attachment of glutamate receptor subtypes to this scaffold.
Glutamate is the principal excitatory neurotransmitter in the CNS, acting via NMDA receptors, non-NMDA receptors (AMPA and kainate), and metabotropic glutamate receptors. Similar to the clustering of acetylcholine receptors at the neuromuscular junction (for review, see Hall and Sanes, 1993), all three glutamate receptor types can be concentrated at postsynaptic sites (Petralia and Wenthold, 1992; Craig et al., 1993; Aoki et al., 1994; Nomura et al., 1994; Petralia et al., 1994a,b; Siegel et al., 1994). The existence of a large number of glutamate receptor subtypes raises the possibility that receptor subtypes may be targeted differentially among glutamatergic synapses on a single neuron (Rubio and Wenthold, 1997). Although some previous studies suggest that glutamate receptor subtypes can colocalize to function in concert at some individual postsynaptic sites (Bekkers and Stevens, 1989; Jones and Baughman, 1991; Nusser et al., 1994; Siegel et al., 1995; Kharazia et al., 1996; Rubio and Wenthold, 1997), the extent to which these receptors colocalize at single synaptic sites is still under investigation. Particularly for the NMDA- and AMPA-type receptors, the extent of colocalization is of interest, because some models of long-term potentiation (LTP) suggest that LTP occurs by conversion of postsynaptic sites containing only NMDA receptors to sites containing both NMDA and AMPA receptors (Isaac et al., 1995; Liao et al., 1995) (but see also Kullmann et al., 1996). An extension of this model suggests that functional glutamatergic transmission develops by the conversion (by a form of potentiation) of pure NMDA receptor-based synapses into conducting synapses containing both types of receptor (Durand et al., 1996). In this study we determined the relationship between NMDA- and AMPA-type receptors in immunocytochemical “hot spots” or clusters during the development of hippocampal neurons in culture.
Recently, a number of proteins that interact specifically with the C-terminal tails of glutamate receptor subunits have been identified; among these proteins PSD-95/SAP90 (Cho et al., 1992; Kistner et al., 1993; Kornau et al., 1995; Niethammer et al., 1996; Hsueh et al., 1997), chapsyn-110/PSD-93 (Brenman et al., 1996; Kim et al., 1996), SAP102 (Muller et al., 1996), α-actinin-2 (Wyszynski et al., 1997a), and calmodulin (Ehlers et al., 1996) interact with NMDA receptors. PSD-95, chapsyn-110, and SAP102 are closely related proteins that contain PDZ protein interaction domains (for review, see Kornau et al., 1997). Strong genetic evidence exists in other systems for a function of PDZ domain-containing proteins in localizing their receptor/channel ligands to specific membrane domains (Simske et al., 1996; Tejedor et al., 1997; Tsunoda et al., 1997). For the PSD-95 family, multiple lines of evidence in vitro indicate that these proteins interact with NMDA receptors and with each other with high specificity and affinity, making them potential postsynaptic scaffolding proteins. The protein Guanylate kinase domain- associated protein (GKAP) was isolated by its ability to bind to the guanylate kinase domain of the PSD-95/SAP90 family (Kim et al., 1997; Naisbitt et al., 1997; Takeuchi et al., 1997) and thus also may form part of a postsynaptic scaffold. We compared the distributions of PSD-95, chapsyn, GKAP, and α-actinin-2 at different developmental stages with those of the NMDA and AMPA receptors and presynaptic markers to define the potential function of each of these proteins in the development of glutamatergic postsynaptic sites.
MATERIALS AND METHODS
Cell culture. Hippocampal neuronal cultures were prepared from 18-d-old embryonic rats, as in Banker and Cowan (1977)and Goslin and Banker (1991). Briefly, neurons were isolated by trypsin treatment and trituration and plated on poly-l-lysine-coated glass coverslips in minimal essential medium (MEM) with 10% horse serum at a density of 2000 cells/cm2. After attachment of cells the coverslips were transferred, and the neurons were maintained by growing them over a glial monolayer in serum-free MEM with N2 supplements (Bottenstein and Sato, 1979). Cytosine arabinoside was added after 2 d to inhibit glial proliferation. The neurons were fixed 1–42 d after plating and used for immunocytochemical staining. The population of neurons in culture consists mostly of pyramidal cells, with ~7% of the cells being GABAergic interneurons (Benson et al., 1994). The observations described here are of pyramidal cells, unless specified otherwise.
Immunocytochemistry. Coverslips used for NMDA receptor or GKAP immunostaining were fixed with methanol for 10 min at −20°C. For reasons that are not clear, specific NMDA receptor staining was obtained only with methanol fixation and not with paraformaldehyde. This was true with independent antibodies against NR1, NR2A, and NR2B, suggesting that methanol may remove a masking protein or otherwise affect antigen accessibility. Immunocytochemistry not involving NMDA receptors or GKAP was performed by fixing neurons in 4% paraformaldehyde/4% sucrose in PBS for 15 min at 37°C and permeabilizing them for 5 min in 0.25% Triton X-100. Coverslips were blocked with 10% BSA in PBS and exposed to primary antibodies in 3% BSA in PBS. Primary antibodies were visualized with fluorochrome-conjugated secondary antibodies (2.5 μg/ml, Vector Laboratories, Burlingame, CA) or with biotin-conjugated secondary antibody (2.5 μg/ml), followed by fluorochrome-conjugated streptavidin (500 ng/ml). The fluorochromes used were fluorescein, Texas Red, and 7-amino-4-methylcoumarin-3-acetic acid (AMCA). Coverslips were mounted in Tris-HCl, glycerol, and polyvinyl alcohol with 2% 1,4-diazabicyclo[2,2,2]octane. Fluorescent images of cells were captured on a Photometrics cooled CCD camera mounted on a Zeiss Axioskop microscope (Oberkochen, Germany) with a 63×, 1.4 numerical aperture (NA) or a 40×, 1.3 NA lens, using Oncor imaging software. Images were prepared for printing with Adobe Photoshop.
The mouse monoclonal antibody 54.1 to NMDAR1 (PharMingen, San Diego, CA) (Siegel et al., 1994) was used at a concentration of 0.1–3 μg/ml. There was a large amount of variation in staining intensity between different lots of the antibody obtained from the manufacturer. The other antibodies used were as follows: rabbit anti-α-actinin-2 antisera (4B2, 1:500; gift of A. H. Beggs, Harvard University, Cambridge, MA) (Wyszynski et al., 1997a), mouse monoclonal anti-α-actinin antibody (clone EA-53, 1:20,000; Sigma, St. Louis, MO), rabbit anti-NR2A antisera (1:80; Sheng et al., 1994), rabbit anti-NR2B antisera (1:100; Upstate Biotechnology, Lake Placid, NY), rabbit anti-MAP2 (266, 1:20,000; gift of S. Halpain, Scripps Institute, La Jolla, CA) (Halpain and Greengard, 1990), guinea pig anti-GluR1 antiserum (1:1600; gift of R. L. Huganir, Johns Hopkins University, Baltimore, MD) (Blackstone et al., 1992), rabbit anti-synaptophysin (1:8000; gift of P. DeCamilli, Yale University, New Haven, CT) (Navone et al., 1986), mouse anti-GABAA receptor β2/3 subunit (clone bd17, 1:100; Boehringer Mannheim, Indianapolis, IN) (Ewert et al., 1990), guinea pig anti-PSD-95 (1:300; Kim et al., 1995), rabbit anti-chapsyn (1:50; Kim et al., 1996), rabbit anti-GKAP antibodies 1564 and 9589 (1:300; Naisbitt et al., 1997), and mouse anti-SV2 (1:100; gift of K. M. Buckley, Harvard University) (Buckley and Kelly, 1985). The specificity of all of these antibodies has been demonstrated previously. Primary antibodies were incubated together for the double- and triple-labeling experiments; controls showed no cross-reactivity.
RESULTS
The NMDA receptor is always present in a punctate pattern and is restricted to the somatodendritic domain in hippocampal neurons in culture
We double-labeled hippocampal neurons in culture with antibodies to the essential NR1 subunit of the NMDA receptor and to the dendritic protein, microtubule-associated protein 2 (MAP2; Fig.Fig.1).1). At 2–5 d in culture, NMDA receptor staining was already punctate, the puncta restricted to developing dendrites and excluded from axons. At subsequent stages of development the same compartmental restriction was seen, in that NR1 staining was not observed in isolated axons. Diffuse receptor may be present but was not detected reliably above background. Bright spherical clusters of NR1 were apparent at 2–5 d both in cell bodies and radiating into the proximal dendrite shafts (Fig. (Fig.11C). These clusters formed in isolated cells on dendrites with no contact with axons, either from the same cell or from surrounding cells. Between 5 and 14 d in culture, these bright NR1 clusters formed arrays that traversed the length of individual dendrites without any readily apparent relationship to sites of axon contact seen by phase contrast (Fig.(Fig.11D–F). There were no obvious morphological differences between dendrites containing or lacking these NR1 clusters.
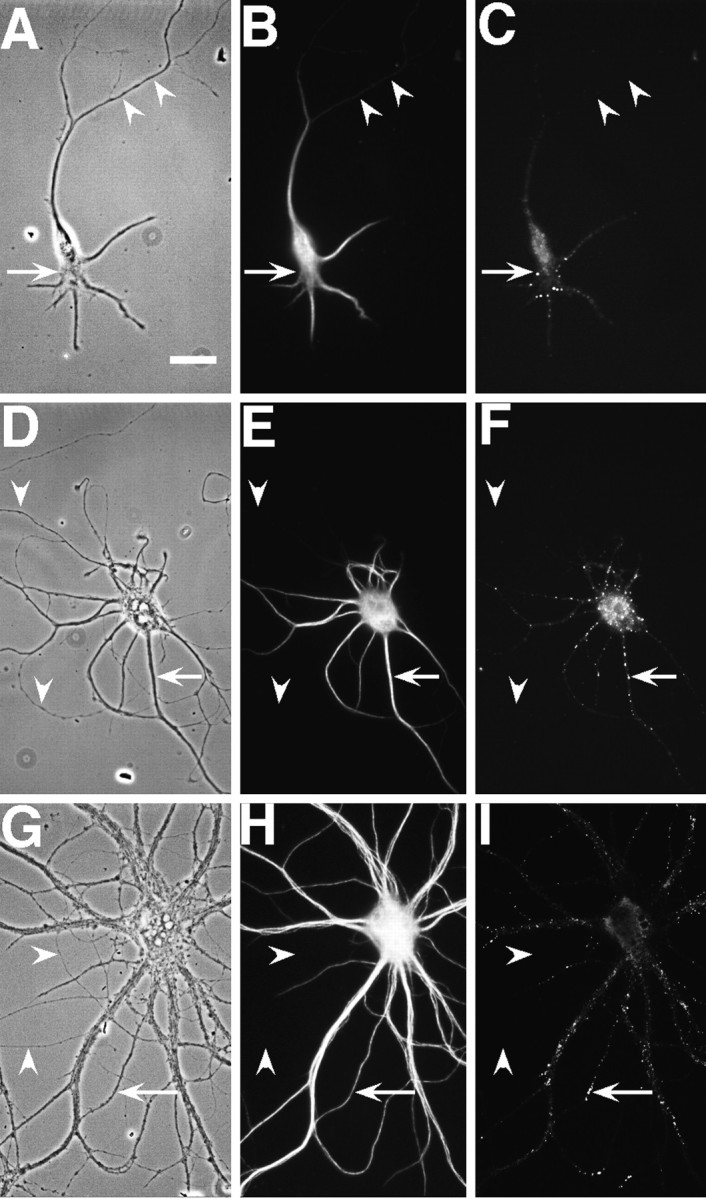
NR1 puncta are restricted to the somatodendritic domain in hippocampal neurons in culture. Hippocampal cultures were fixed at 3 (A–C), 9 (D–F), and 23 (G–I) d and immunostained for the dendritic marker MAP2 (B, E,H) and NR1 (C, F,I). In the phase-contrast images (A, D, G), axons can be seen traversing the substrate (arrowheads), whereas in the paired immunolabeled images no immunostaining can be observed in these processes. Clusters of NR1 (arrows) are evident in the soma and proximal dendrites of the isolated 3-d-old cell in the absence of axonal contacts. At later stages, NR1 clusters move further into the dendrite shafts (F) and by 3 weeks (I) are at the tips of dendrites. Scale bar, 20 μm.
Between 14 and 28 d in culture the majority of neurons in culture showed bright arrays of NR1 clusters only at the distal tips of dendrite branches or on fine-caliber dendrites near the cell body; these clusters were present on the shafts rather than on the spines of dendrites (Fig. (Fig.11I). A few neurons showed the proximal dendrite arrays of clusters that were observed in younger cultures, and an even smaller number showed a spiny distribution of NR1 clusters all along the length of the dendrites (Fig.(Fig.22J). There was some variability among cultures with regard to when the spiny pattern of staining first appeared; in most cultures it was not detectable until 5–6 weeks in culture, whereas in others it was seen in a minority of neurons at 3–4 weeks. There appeared to be a correlation between cell density and development of spiny synaptic NR1 clusters; cultures with very high cell density showed some cells with spiny clusters as early as 2 weeks (data not shown). The high-density cultures were not used for this analysis, because the immunocytochemistry was less informative with the dense overgrowth of processes in these cultures. The NMDA receptor distribution also was markedly affected by neuronal activity (Rao and Craig, 1997); the results reported here were obtained with neurons developing without pharmacological manipulation under conditions that allow spontaneous activity.
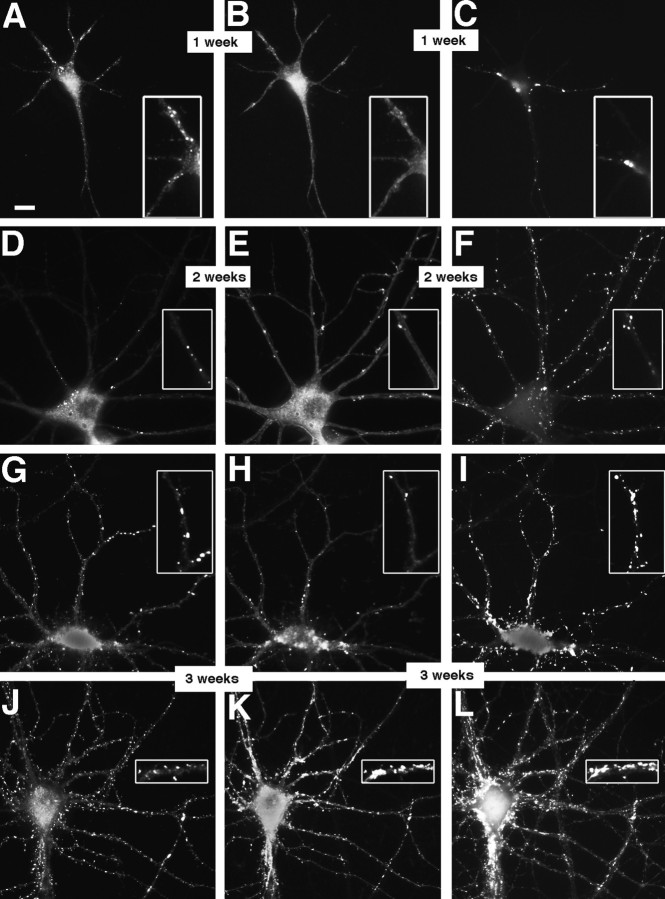
NMDA- and AMPA-type receptors cluster separately until late in development. Cultures fixed at 5 (A–C), 14 (D–F), and 21 (G–L) d in vitro were immunostained for NR1 (A, D,G, J), GluR1 (B,E, H, K), and the presynaptic marker synaptophysin (C, F,I, L). In the 5 d cell, NR1 (A) forms clusters at sites distinct from the synaptic sites indicated by synaptophysin clusters (C). GluR1 staining is detectable but diffuse (B). At 14 d, NR1 clusters have moved out into the proximal dendrite (D) and are still distinct from presynaptic sites (F). GluR1 forms aggregates at spiny sites along the full length of the dendrites (E), always apposed to presynaptic sites (F). By 21 d, two additional patterns of NR1 staining are apparent. Figure legend continues. NR1 can form arrays of large, brightly labeled clusters at the distal dendrite shaft (G) or can cluster at spiny sites throughout the dendritic arbor (J). The distal dendrite shaft NR1 clusters are mostly nonsynaptic but sometimes synaptic (compareG and I) and lack concentrations of GluR1, which is still clustered in spines (H). The spiny NR1 clusters (J) are apposed to presynaptic sites (L) and often colocalize with GluR1 clusters (K). Scale bar, 10 μm.Insets show magnified regions from the full panels.
NMDA and AMPA receptors cluster with different time courses and at different sites during development
We used triple-label immunostaining with antibodies to NR1, GluR1, and synaptophysin to visualize the sites of NMDA receptor clusters, AMPA receptor clusters, and presynaptic specializations simultaneously (Fig. (Fig.2).2). Synaptophysin clusters corresponding to presynaptic specializations form at sites of contact between axons and dendrites or cell bodies beginning at 3 d in culture (Fletcher et al., 1991). GluR1 is present in all AMPA receptor clusters in these neurons and changes from a diffuse dendritic pattern to synaptic clusters beginning at ~9 d in culture (Craig et al., 1993). During the first week in culture, in contrast to the diffuse GluR1 pattern, NR1 staining was present in distinct clusters on the soma and along the dendrites (Fig. (Fig.22A). These NR1 clusters did not colocalize with punctate synaptophysin staining and were observed in the presence or absence of synaptophysin-labeled contacts elsewhere on the dendrites (Fig. (Fig.22C). During the second week in culture GluR1 became clustered at spines all along the length of the dendrites (Fig. (Fig.22E), always apposed to punctate synaptophysin (Fig. (Fig.22F), whereas the NR1 was arrayed in spherical clusters on the proximal dendrite shaft (Fig. (Fig.22D) and not colocalized with synaptophysin or GluR1. During the third week two other patterns of staining developed. Most of the cells showed bright arrays of NR1 clusters on one or two distal dendritic shafts in each cell. Some of these distal NR1 clusters still did not colocalize with GluR1 or with synaptophysin clusters, whereas others colocalized with synaptophysin, but not GluR1 clusters (Fig. (Fig.22G–I). The other pattern, seen only in a few cells during the third week but in more cells in the fourth and fifth week as described above, was of NR1 clusters predominantly at dendritic spines, which were colocalized mostly with synaptophysin clusters and some, but not all, with GluR1 clusters (Fig. (Fig.22J–L).
Thus, we first observed NR1 clusters very early in development in cell bodies and along proximal dendrite shafts, at nonsynaptic sites, and independently of cell–cell contact. This was in contrast to GluR1, which has been shown here and previously (Craig et al., 1993) to form clusters later in development, always at dendritic spines and apposed to presynaptic terminals. The NR1 pattern shifted slowly during development to one of NR1 clusters on distal dendrite shafts, some of which were synaptic, and then finally to one in which NR1 clustered at dendritic spine synapses, colocalized with GluR1.
We compared the pattern of NR1 staining with that of two other NMDA receptor subunits, NR2A and NR2B (Fig.(Fig.3).3). NR2A and NR2B are the most abundant in hippocampal pyramidal cells of the four accessory subunits NR2A–D that can combine with NR1 to produce a functional receptor (Monyer et al., 1994). Both NR2A and NR2B were colocalized with NR1 at all types of NR1 clusters, including proximal and distal nonsynaptic shaft clusters, distal synaptic shaft clusters, and synaptic spiny clusters. NR2A and NR2B were detectable at proximal dendrite shaft clusters as early as NR1, at 2–5 d in culture, and were at most, if not all, NR1 clusters. In short, NR2A and NR2B colocalized with NR1 at all stages of development from 2 d to 5 weeks in culture.
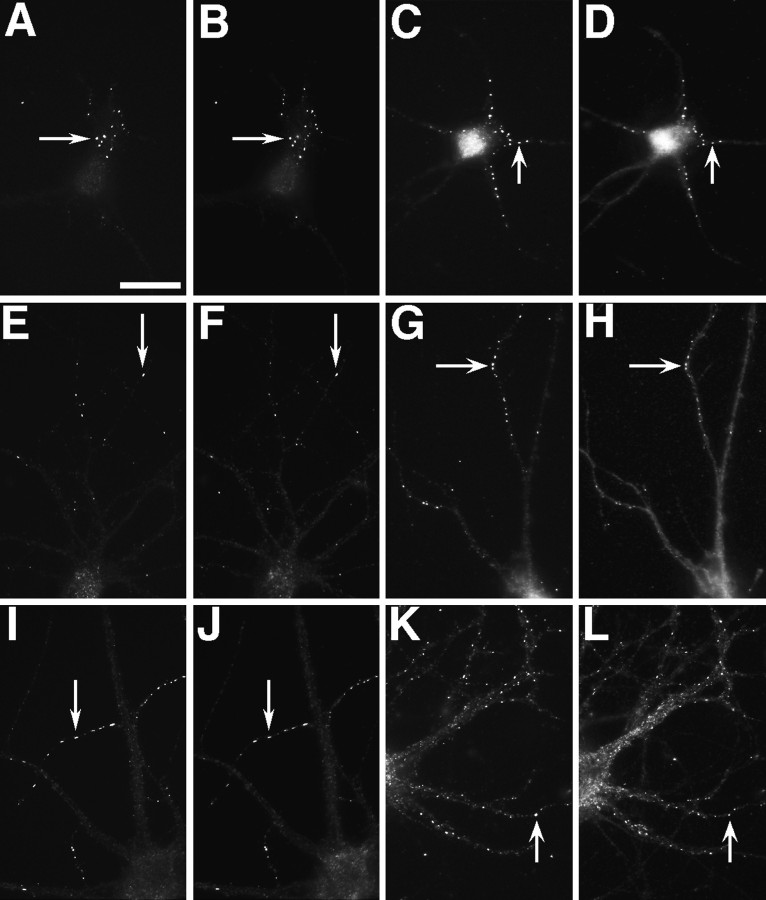
NR2A and NR2B are present at all NR1 clusters, including synaptic and nonsynaptic types. Hippocampal cultures fixed at 5 (A–D), 12 (E–H), 21 (I,J), and 28 d (K,L) were immunostained for NR1 (A,C, E, G, I,K) and NR2A (B, F,J) or NR2B (D, H,L). The two NR2 receptor subunits colocalized with NR1 at all of these stages of development, corresponding to the proximal shaft, distal shaft, and spiny clustering patterns. Arrowsindicate prominent NR1 clusters, which show co-localized NR2 subunits. Scale bar, 10 μm.
NMDA receptor-associated proteins are present at excitatory synaptic sites and absent from inhibitory synapses
We determined whether the NMDA receptor-associated proteins PSD-95, chapsyn, and α-actinin-2 and the associated protein GKAP were present at all synaptic sites or only at excitatory synapses by double-labeling for these proteins along with either GluR1 or NR1 (as markers of excitatory postsynaptic sites) or with the GABAAreceptor β2/3 subunit or glutamic acid decarboxylase (GAD; as markers for GABAergic synapses). Neurons stained for PSD-95 showed very prominent clustered staining with low levels of diffuse staining in the dendrite (Fig. (Fig.4),4), as reported by Kornau et al. (1995). The clusters were present both on spines and on dendritic shafts. Comparing the pattern of PSD-95 staining with that of GluR1, we observed PSD-95 clusters at all GluR1 clusters. In contrast, PSD-95 clusters did not colocalize with GABA receptor clusters; these two proteins were present in distinct and mainly nonoverlapping patterns. Although there were some brightly labeled PSD-95 clusters that did not show concentrations of GluR1 (data not shown), additional experiments suggested that these PSD-95 clusters correspond to a subset of excitatory synapses lacking concentrations of AMPA receptor (i.e., with triple labeling, they stain for PSD-95 and synaptophysin, but not GAD). The same pattern of localization at glutamatergic, but not GABAergic, sites was seen for chapsyn (data not shown), α-actinin-2 (Wyszynski et al., 1997b), and GKAP (Naisbitt et al., 1997)

PSD-95 is present at excitatory synaptic sites, but not at inhibitory synapses. Hippocampal neurons fixed at 21 d in culture were immunostained for PSD-95 (A,C) and either GluR1 (B) or the GABAA receptor β2/3 subunit (D). PSD-95 clusters colocalized with GluR1-labeled excitatory synapses (indicated by arrows in A,B), but not with the GABAA receptor-labeled inhibitory synapses (arrows in C,D). Scale bar, 10 μm.
PSD-95 may be necessary for development of all excitatory synapses, but its presence is not sufficient for synaptic clustering of NMDA receptors
Cells fixed between 3 and 35 d in culture were double-labeled for PSD-95 and NR1 (Fig. (Fig.5).5). PSD-95 clusters were restricted to the somatodendritic domain of hippocampal neurons almost as soon as axons were detectable in these cells, within the first 3 d in culture. At the earliest stages both NR1 and PSD-95 were clustered, but not necessarily at the same sites. Some cells showed NR1 clusters of the typical nonsynaptic pattern (as in Fig. Fig.22A–C) with no concentrations of PSD-95, suggesting that PSD-95 is not necessary for the formation of these nonsynaptic clusters (Fig. (Fig.55A,B). Because NR2A and NR2B colocalize with NR1 throughout development (see above), this result also implies that PSD-95 and NR2 do not always interact within the neuron. Other neurons had nonsynaptic NR1 clusters colocalizing with PSD-95 clusters, suggesting that association of the NMDA receptor with PSD-95 is not sufficient to target either protein to synapses.
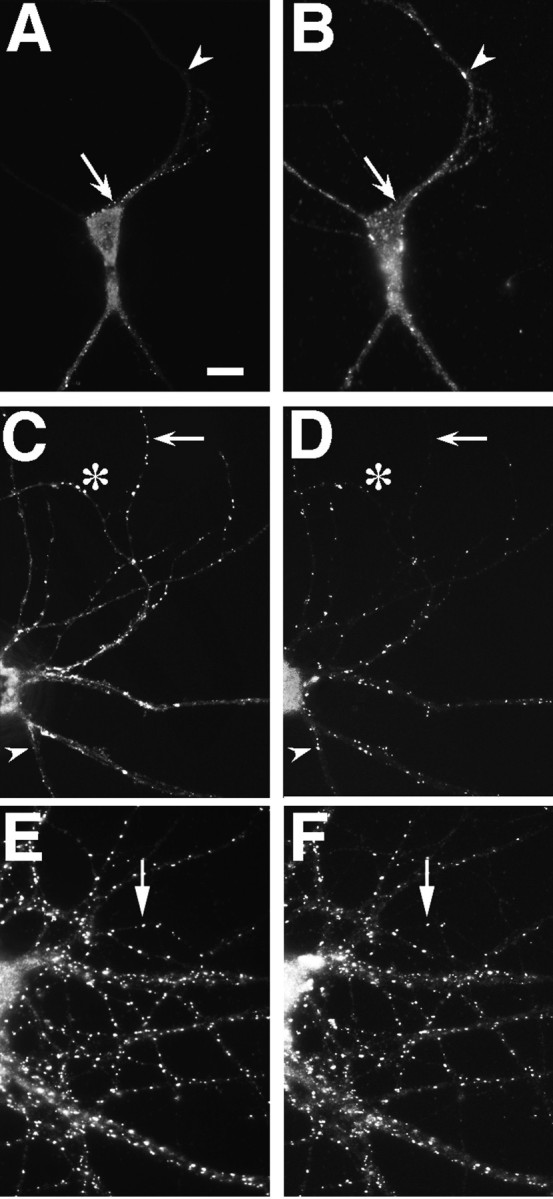
PSD-95 and NR1 can cluster separately early in development but colocalize in older neurons. Neurons fixed at 3 (A, B), 14 (C,D), and 35 (E, F) d in culture were immunostained for NR1 (A,C, E) and PSD-95 (B,D, F). Nonsynaptic-type clusters of NR1 in the 3 and 14 d cells can form in the absence of PSD-95 staining (A–D, arrows), indicating that PSD95 is not necessary for clustering at nonsynaptic sites. Frequently, as in other sites on these cells, NR1 and PSD-95 do colocalize at these clusters. Clustering of PSD-95 without NR1 (A,B, arrowheads) indicates that PSD-95 clustering is not sufficient to induce NR1 clustering at the same site. The asterisk in C and Dshows distal dendrite NR1 clusters that do colocalize with PSD-95 and may be synaptic. In more mature neurons, at a stage when NR1 is spiny and probably synaptic (compare with Fig. Fig.22J,L), NR1 and PSD-95 clusters are colocalized almost completely (E, F, arrows). Scale bar, 10 μm.
At 2 weeks in culture (Fig. (Fig.55C,D), PSD-95 was present at some, but not all, proximal and distal nonsynaptic-type clusters of NR1, at some distal dendrite shaft NR1 clusters that are often synaptic (as in Fig. Fig.22G–I), and at numerous spiny synaptic sites lacking detectable NR1 immunoreactivity. Beginning at approximately the third week in culture, PSD-95 was a more reliable marker of postsynaptic glutamatergic sites than either GluR1 or NR1 alone, because it was present at both kinds of clusters (Fig.(Fig.55E,F for NR1 and PSD-95) (colocalization with GluR1 in Fig.Fig.44A,B). The observation that NR1 is not present at all PSD-95 clusters and specifically not present at most of the synaptic PSD-95 clusters at 2–3 weeks in culture indicates that the presence of a PSD-95 cluster is not sufficient to induce NMDA receptor localization to synapses. At 3 weeks and subsequently, all NR1 clusters also exhibited strong immunoreactivity for PSD-95. Synaptic clusters of NMDA receptor subunits in the absence of a synaptic PSD-95 cluster were never observed, consistent with the idea that PSD-95 may be necessary for the formation of synaptic clusters of the NMDA receptor.
Chapsyn-110 showed a very similar developmental distribution to PSD-95, being clustered at very early stages, sometimes but not always colocalized with the NMDA receptor, and subsequently becoming clustered at all glutamatergic synapses (Fig. (Fig.6).6). In addition to the clusters in the dendrites and soma, chapsyn, but not PSD-95, also became concentrated in the axon initial segment. Chapsyn was not detected along the bulk of the axon, only at the axon initial segment, where it was not clustered but diffusely localized.
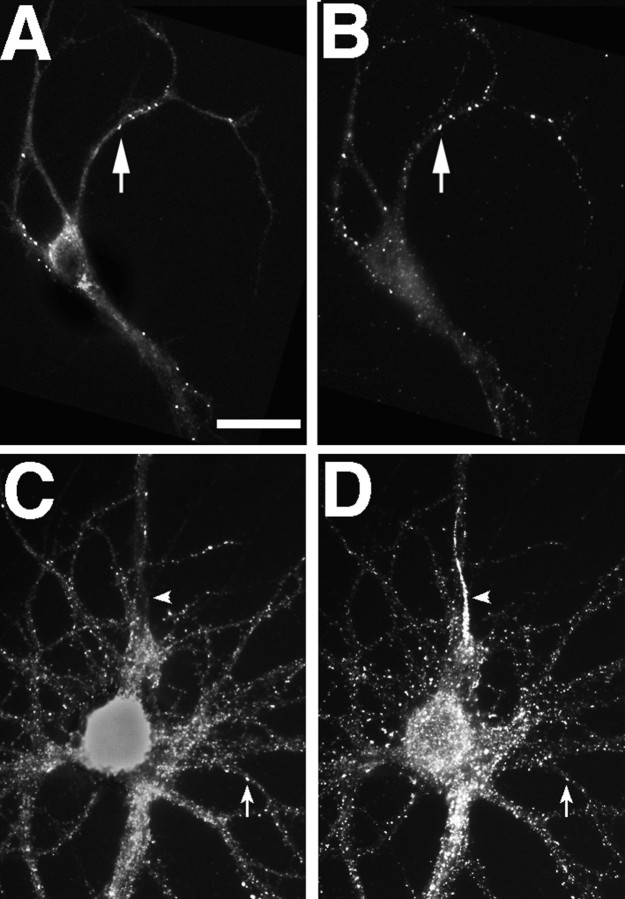
Chapsyn-110 can cocluster with NR1 but also is concentrated at the axon initial segment. Neurons fixed at 5 (A, B) and 35 (C,D) d in culture were immunostained for NR1 (A, C) and chapsyn (B,D). Like PSD-95, chapsyn clustered with NR1 at some sites early in development (A, B,arrow) but also could cluster separately. In mature neurons, chapsyn coclustered with spiny NR1 (C,D, arrow) but also was concentrated at the axon initial segment without concentrations of NR1 (C, D, arrowhead). Scale bar, 20 μm.
GKAP colocalizes closely with PSD-95: both cluster at synaptic sites before NMDA and AMPA receptors
We examined the distribution of GKAP in developing hippocampal cultures with two different antibodies, one (number 1564) directed against the N-terminal 434 amino acid residues and one (number 9589) directed against amino acid residues 446–666 of GKAP2.1 (Naisbitt et al., 1997). There was no difference in the patterns of immunostaining that were obtained with these two antibodies (Fig.(Fig.77A–C vsD–F). Both showed punctate staining, first at nonsynaptic and then at synaptic sites, which completely colocalized with PSD-95. There was some variation in the relative intensities of staining of individual clusters for PSD-95 versus GKAP, but all clusters observed with one antibody also stained with the other throughout development from 3 to 28 d in culture. Compared with NR1 during the first week in culture, GKAP was present at a subset of nonsynaptic-type NR1 clusters; it also formed some additional nonsynaptic and synaptic-type clusters on dendrites (data not shown). As with PSD-95 and chapsyn, formation of NR1 clusters without associated GKAP suggests that GKAP is not necessary fornonsynaptic clustering of NR1.
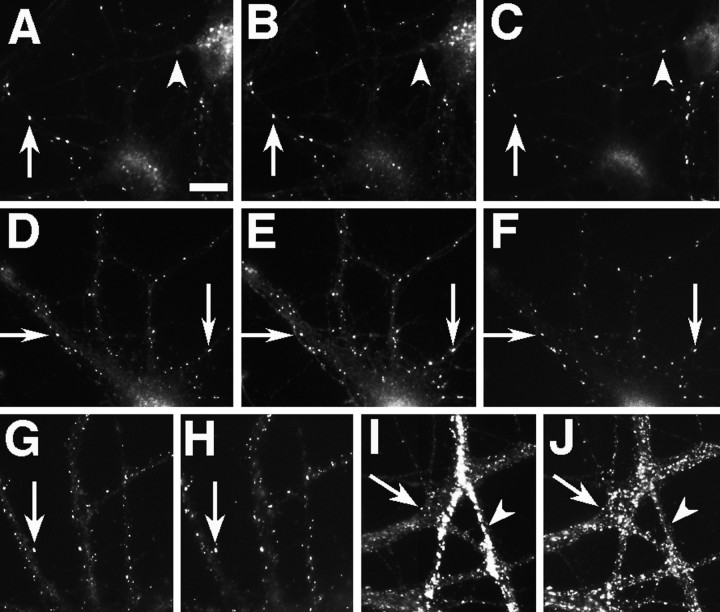
GKAP and PSD-95 are the earliest components of developing glutamatergic postsynaptic sites. Neurons fixed at 5 (A–C), 10 (D–F), and 19 (G–J) d in culture were immunostained for GKAP (A, N-terminal antibody;D, G, I, C-terminal antibody), PSD-95 (B, E,H, J), and the presynaptic marker SV2 (C, F). GKAP and PSD-95 were always colocalized (arrows in A andB, D and E,G and H). At very early stages GKAP and PSD-95 clusters were prominent, apposed to some presynaptic sites (A–C, arrow) but faint at others (A–C,arrowhead). By 10 d, GKAP and PSD-95 appeared at almost all synaptic sites (D–F,arrows) as well as some nonsynaptic sites. At these stages NR1 is completely nonsynaptic, and GluR1 is clustered in very few cells (see Results and Fig. Fig.2),2), so GKAP and PSD-95 must form clusters at synaptic sites before glutamate receptors. In mature neurons GKAP still coclusters with PSD-95 at all sites but is enriched in GABAergic interneurons, when compared with pyramidal neurons (G–J). GKAP and PSD-95 colocalize on the spines of mature pyramidal neurons (G,H, arrow). In I andJ, the dendrites of a GABAergic cell cross the field vertically, whereas the dendrites of a pyramidal cell cross horizontally. GKAP staining in the GABAergic cell dendrite (I, arrowhead) is far brighter than in the pyramidal cell (I, arrow), when compared with the uniform levels of PSD95 staining at the same sites (J). Scale bar, 10 μm.
Both GKAP and PSD-95 formed a large number of clusters at 5 d in culture and thereafter, which were apposed to synaptic vesicle clusters (assessed by staining for the synaptic vesicle protein SV2) and therefore classified as synaptic (Fig. (Fig.77A–F). As described above, NMDA receptor does not cluster at synaptic sites until ~14 d in culture (and usually much later), and GluR1 clusters start to appear in a few cells at ~9 d. There was a marked discrepancy between the large number of synaptic GKAP and PSD-95 clusters during the first 2 weeks in culture, as compared with the small number of synaptic sites with clusters of either NMDA or AMPA receptors, indicating that GKAP and PSD-95 cluster at synaptic sites long before these glutamate receptors. Thus GKAP and PSD-95 were the best early markers of developing excitatory postsynaptic sites, apparently forming part of an initial postsynaptic scaffold shortly after the formation of presynaptic specializations. At this stage the NMDA receptor was present in nonsynaptic clusters, and AMPA receptors were diffusely localized in the membrane, but neither receptor coclustered with GKAP and PSD-95 at synaptic sites, suggesting that an additional regulatory step was necessary to reroute receptors to synaptic sites.
Another interesting feature of the distribution of GKAP in mature neurons was its relative abundance in different cell types. GKAP was present at all excitatory postsynaptic sites but appeared much more abundant in GABAergic inhibitory interneurons than in pyramidal cells (Fig. (Fig.77I,J). This distribution is almost complementary to that of α-actinin-2 (see below).
α-Actinin-2 is concentrated in developing spine synapses and is absent from excitatory shaft synapses
The developmental distribution of α-actinin-2 was very different from that of PSD-95 and chapsyn. Up to 3 weeks in culture, α-actinin was not associated with NR1 clusters (Fig.(Fig.88A,B), although it was present in a spiny clustered pattern from as early as 10 d in culture. After ~3 weeks in culture those cells that developed spiny NR1 clusters displayed clusters of α-actinin at the spiny sites (Fig.(Fig.88C,D), but not at shaft clusters of NR1. The finding that α-actinin does not colocalize with NR1 until NR1 becomes associated with dendritic spines suggests that α-actinin is not sufficient or necessary for the formation of nonsynaptic NR1 clusters or shaft NR1 clusters, but it may be important in the localization of NR1 clusters to dendritic spines.
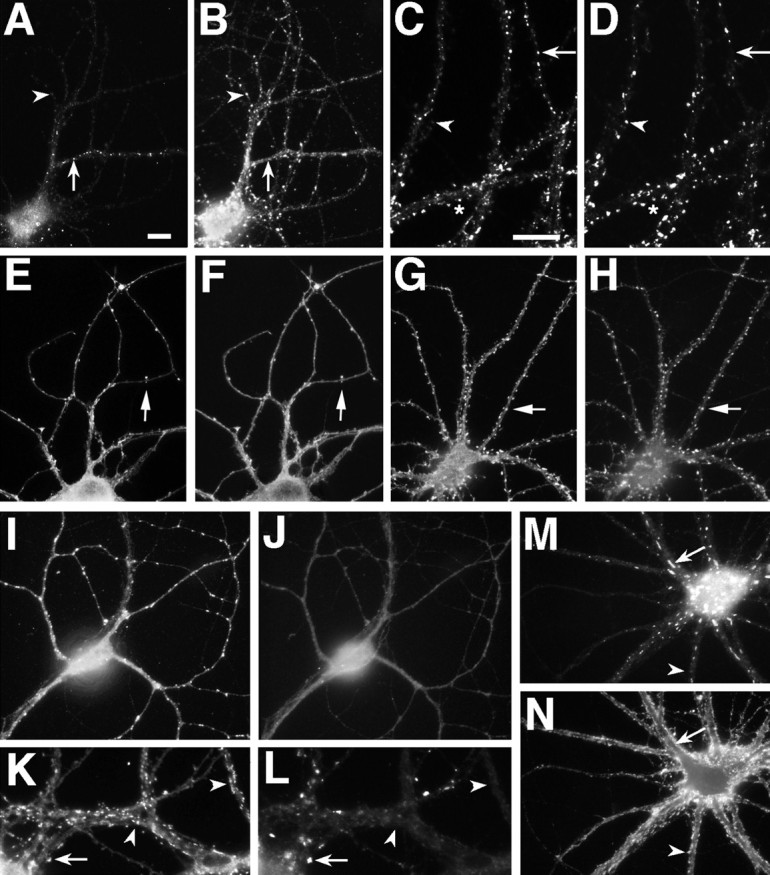
α-Actinin-2 is associated with dendritic spines. NR1 (A, C) and α-actinin-2 (B, D) were not colocalized at shaft clusters of NR1 (arrows) in either 19 (A,B) or 28 (C, D) d cultured hippocampal neurons. α-Actinin-2 formed spiny clusters all over the dendritic tree at 19 d (B) and earlier (F). NR1 was not present at most of these spiny clusters (A–D,arrowheads), but when NR1 clusters became spiny, they colocalized with α-actinin-2 (C, D,asterisk). GluR1 (E, G) clusters colocalized with α-actinin-2 (F,H) at dendritic spines from the earliest time GluR1 clusters were visible (10 d cell in E,F) and thereafter (21 d cell in G,H). In GABAergic cells in the culture (I–L), GluR1 clustered on dendrite shafts (I, K). α-Actinin-2 (J, L) was not present at these shaft GluR1 clusters. In K and L, GABAergic cell dendrites cross from the right(arrowheads) to intersect with pyramidal neuron dendrites coming from the left (arrow). α-Actinin-2 is present at the spiny GluR1 clusters of the pyramidal cell (arrow), but not at the shaft GluR1 clusters of the GABA cell (arrowhead). Frequently, α-actinin-2 also formed elongated clusters in the soma and dendrite core (M, arrow), which were not at synaptic sites (as indicated by synaptophysin staining inN). Arrowheads indicate synaptic sites. Scale bars: in A, 10 μm (for all panels exceptC, D, K,L); in C, 10 μm for C, D, K, L).
This colocalization of α-actinin with NR1 specifically at dendritic spines led us to compare the distribution of α-actinin with that of GluR1, which is at spines from the earliest time a clustered distribution can be observed. α-Actinin clusters were observed at all GluR1-containing spines from the earliest time that such spines were detectable (Fig. (Fig.88E,F). This colocalization persisted later in development (Fig. (Fig.88G,H). We next determined whether α-actinin was associated specifically with the GluR1 clusters or with dendritic spines, by studying its distribution in GABAergic cells in the culture, which form GluR1 clusters at synaptic sites on the dendrite shaft rather than on spines (Craig et al., 1993). There was no detectable α-actinin staining in GABAergic cells at GluR1 clusters, indicating that α-actinin is associated not with glutamatergic synapses as such but with glutamatergic synapses on dendritic spines in pyramidal cells (Fig. (Fig.88I–L). In addition to the pattern of spiny α-actinin staining, α-actinin also was frequently present in large elongated clusters within the dendrite shaft of pyramidal neurons. These shaft clusters were not associated with synaptic sites (Fig. (Fig.88M,N) or with staining for the other synapse-associated proteins studied here, but they were associated with filamentous actin, as shown by fluorescent phalloidin staining (data not shown).
DISCUSSION
The major findings of this study include the following. (1) The NMDA- and AMPA-type glutamate receptors cluster at different dendritic sites in a different temporal sequence in hippocampal neurons and thus must be aggregated and targeted by different mechanisms. (2) The NMDA receptor is capable of forming clusters in the absence of presynaptic input and of the putative postsynaptic clustering/anchoring proteins PSD-95, chapsyn, GKAP, and α-actinin-2. (3) PSD-95, chapsyn, and GKAP cluster at synaptic sites before either NMDA or AMPA receptors can be detected at these sites. (4) Clustering of PSD-95, chapsyn, GKAP, and α-actinin at synapses is not sufficient to induce synaptic clusters of NMDA receptor, but (5) synaptic clustering of PSD-95, chapsyn, and GKAP may be necessary for the formation of synaptic clusters of the NMDA receptor. (6) α-Actinin is associated with clusters of the NMDA receptor only at dendritic spines. The stages observed in the development of excitatory postsynaptic sites in cultured hippocampal neurons are diagrammed in Figure Figure99.
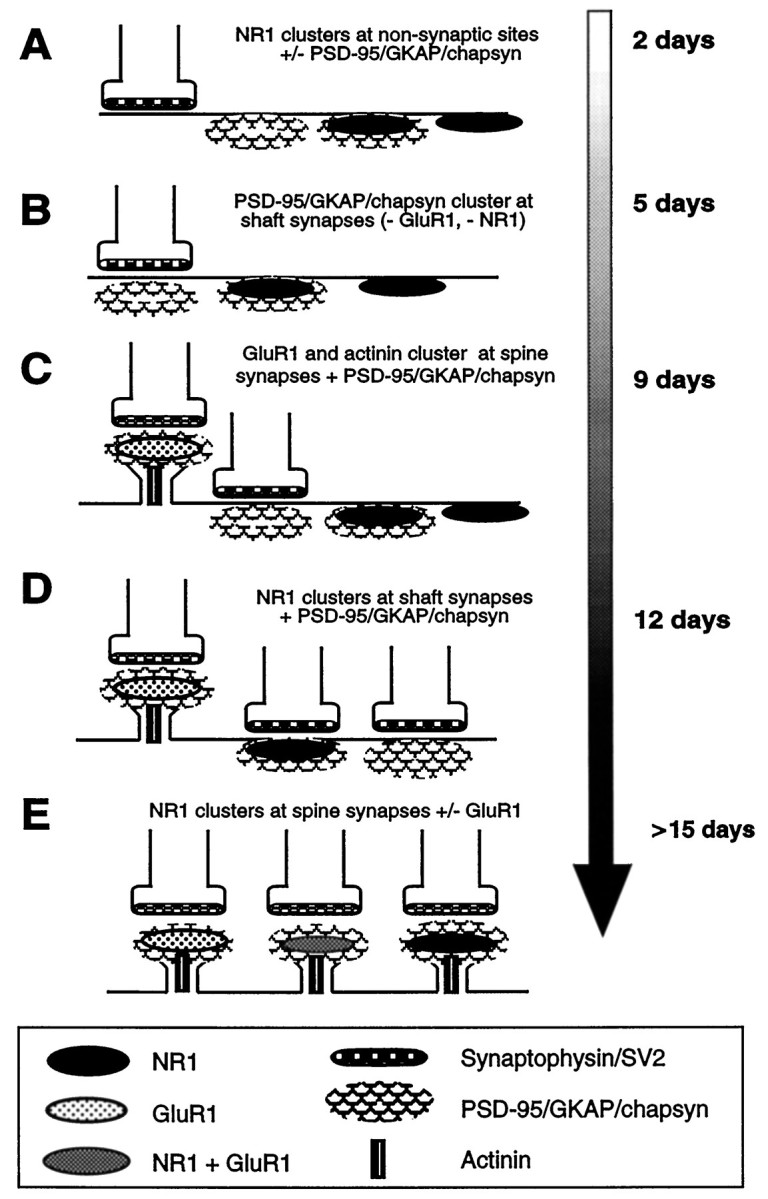
Summary of the stages of development of excitatory postsynaptic sites on hippocampal pyramidal neurons, as indicated by the molecular markers that were used in this study. The time line indicates the earliest time in culture at which each event was observed; many of these events occurred over a span of days to weeks.A, NMDA receptors were clustered from the earliest stages in development at nonsynaptic sites in the soma and proximal dendrite shaft; some clusters colocalized with PSD-95, GKAP, and chapsyn, and some did not. B, PSD-95, GKAP, and chapsyn formed clusters within the first week in culture at dendrite shaft synapses lacking clusters of either NMDA or AMPA receptors.C, α-Actinin-2 formed synaptic clusters in the second week in culture but only at spine synapses colocalizing with AMPA receptor, but not NMDA receptor clusters. D, During the second and third week in culture, NMDA receptor clusters were predominantly at fine terminal branches of the dendritic tree, many of them colocalizing with PSD-95, GKAP, and chapsyn; some of these were synaptic. E, Finally, only beginning at 3 weeks did NMDA receptor clusters become localized primarily at dendritic spines throughout the extent of the dendritic arborization, where they often colocalized with AMPA receptor clusters and always with α-actinin-2, PSD-95, GKAP, and chapsyn.
Nonsynaptic NMDA receptor clusters
Nonsynaptic NMDA receptor clusters have been reported previously as prominent in developing cortical tissue, some intracellular and some associated with the plasma membrane (Aoki et al., 1994; Johnston et al., 1996). Because methanol fixation was necessary for NMDA receptor visualization in our hippocampal cultures, we were not able to determine whether the nonsynaptic NMDA receptor clusters we observed were part of an intracellular pool or cell surface receptors. What could be the developmental function of these nonsynaptic NMDA receptor clusters? The nonsynaptic clusters were prevalent during the period of initial dendrite outgrowth, whereas the synaptic clusters formed very late in development, when dendritic growth presumably is slower. Furthermore, the nonsynaptic clusters appeared to progress distally along the dendrites with dendrite growth, such that in the majority of the 3 week cultured neurons, the clusters were present only at the finest distal dendritic branches. This developmental progression from a proximal to a distal pattern suggests a potential role in local control of dendrite outgrowth or its cessation. Although total NMDA receptor blockade has no apparent effect on dendritic outgrowth or branching (Kossel et al., 1997), locally applied glutamate can regulate the growth of individual dendrite branches (although possibly via non-NMDA receptors; Mattson et al., 1988).
Differences in NMDA and AMPA receptor distribution
We find major differences in the pattern of development of NMDA as compared with AMPA receptor aggregates in cultured hippocampal neurons. These observations suggest that different mechanisms must be involved in both the formation and synaptic localization of aggregates of these two kinds of receptor.
As discussed earlier (see introductory remarks) it has been suggested that glutamatergic synapses develop by the conversion of “silent synapses” (containing only NMDA receptor) to conducting synapses containing both NMDA- and AMPA-type receptors (Durand et al., 1996). The synaptic NMDA receptor clusters we see at distal dendrite shafts do not colocalize with AMPA receptor clusters and may correspond to physiologically silent synapses, which also were observed on dendrite shafts. Many of the spiny synaptic NMDA receptor clusters seen later in development do colocalize with clusters of AMPA receptor and may represent conducting synapses. However, it is important to note that the inability to detect a receptor immunocytochemically does not necessarily imply the absence of functional protein from these sites, because the threshold for immunocytochemical detection may be high. In addition, although shaft NMDA receptor cluster sites lacked AMPA receptor clusters, the level of diffuse AMPA receptor immunoreactivity was fairly high over much of the somatodendritic domain, so that the shaft synapses need not necessarily be physiologically silent. We did not see any sequence of molecular events corresponding to the addition of AMPA receptor clusters to previously existing NMDA receptor-based synapses. The shaft synaptic clusters of NR1 were few in number and on distal dendrites and thus could not be the major precursor for the numerous spiny synapses containing both receptor types that later developed all along the dendrite length. In fact, our data would suggest that many of these spiny synapses developed AMPA receptor clusters before developing NMDA receptor clusters. Although differences in pattern and number of NMDA and AMPA receptor clusters are inconsistent with a direct conversion of silent synapses to conducting ones, they are consistent with a development and then loss of silent synapses when the total population of synapses is considered. In the hippocampus the number of silent synapses decreases during the first postnatal week as the number of conducting synapses increases (Durand et al., 1996), but the time course in vivo(1 week) is much shorter than ours in culture (3–5 weeks). This difference may be attributable to the decrease in cell density and perhaps to an increase in the time course of synaptogenesis in culture.
Role of NMDA receptor-associated proteins in development of glutamatergic synapses
In hippocampal neurons in culture, PSD-95, chapsyn, and GKAP distributions during development are consistent with a role in glutamatergic synapse formation, although none of these proteins appears to be necessary for the formation of initial nonsynaptic NMDA receptor clusters. Nonsynaptic NMDA receptor clusters may form via the action of some other interacting protein (e.g., SAP102) or simply by an ability of the receptor to self-aggregate (Ehlers et al., 1995).
One of the particularly interesting findings of this study is the early postsynaptic clustering of PSD-95, chapsyn, and GKAP, which apparently form a postsynaptic scaffold to which the receptors later attach. Although formation of synaptic clusters of PSD-95, chapsyn, and GKAP is not sufficient to induce localization of NMDA receptors to synapses, we know that NMDA receptors containing NR1 and NR2 subunits are present at this point and are capable of forming clusters. It is well documented that the NMDA receptor and PSD-95/chapsyn are capable of direct and sequence-specific interaction. These observations together suggest either that a novel late-appearing component of the postsynaptic specialization is required for synaptic localization of the NMDA receptor or that there may be some developmental change in either the receptor or in the interacting protein that regulates their association. Protein kinase A-mediated phosphorylation of the K+ channel Kir2.3 can inhibit its association with PSD-95 (Cohen et al., 1996). Although NR2A and NR2B do not have similar C-terminal phosphorylation sites, the principle of a post-translationally regulated interaction may apply. An analogous mechanism is known to occur at the neuromuscular junction (for review, see Glass and Yancopoulos, 1997). Agrin-induced formation of a muscle-specific kinase (MuSK)-containing scaffold is thought to be an early event in neuromuscular junction formation (Apel et al., 1997). MuSK then recruits the intracellular protein rapsyn, and both MuSK kinase activity and rapsyn are required to recruit the acetylcholine receptor to synapses. Tyrosine phosphorylation of the acetylcholine receptor β-subunit precedes its synaptic localization.
Unlike the other postsynaptic components studied here, α-actinin-2 was not a ubiquitous component of excitatory synapses. Its distribution during development and its known actin bundling activity are consistent with a role in the development and maintenance of dendritic spines. The competitive interaction between α-actinin and calmodulin for binding to NR1 (Wyszynski et al., 1997a) and the inhibitory effect of calmodulin on NMDA receptor function (Ehlers et al., 1996) suggest additional modulatory roles for α-actinin in the regulation of NMDA receptor activity and/or localization in spines.
The concentrations of chapsyn, but not PSD-95, at the axon initial segment suggests an additional function for chapsyn. An obvious potential interacting protein is the voltage-gated sodium channel, which is concentrated at the axon initial segment (Wollner and Catterall, 1986). Some of the muscle forms of the α-subunit of this channel end in the sequence ESXV (Kornau et al., 1995), a potential interacting region for chapsyn; perhaps there are similar axonal subunits. The differential localization of chapsyn and PSD-95 in this region of the neuron also was surprising, given their degree of homology and their ability to interact directly by disulfide linkage of their N-terminal regions (Hsueh et al., 1997). Once again, this observation suggests a modulated interaction, perhaps via post-translational modifications or alternative splicing.
Conclusion
These results indicate an unsuspected heterogeneity in the molecular composition of excitatory postsynaptic sites, extending both to receptors and to potential anchoring/scaffolding proteins. The distributions of all of these proteins in overlapping but distinct developmental patterns suggest that there may be separate mechanisms for clustering each component and for localizing each component to synapses, with regulatory links yet to be discovered. This degree of complexity may allow for subtle modulations in molecular composition associated with synaptic plasticity in mature neurons.
Footnotes
This work was supported by the Markey Charitable Trust, the Pew Charitable Trust, and National Institute of Health Grant NS33184 to A.M.C. M.S. is an Assistant Investigator of the Howard Hughes Medical Institute. We thank Anna S. Serpinskaya, Maureen E. McGrath, and Eric M. Cha for excellent technical support.
Correspondence should be addressed to Dr. Ann Marie Craig, Department of Cell and Structural Biology, University of Illinois, B107, Chemical and Life Sciences Laboratory, 601 South Goodwin Avenue, Urbana, IL 61801.
Dr. Kim’s present address: Department of Pharmacology, Pusan National University, Pusan 609-735, Korea.
REFERENCES
Articles from The Journal of Neuroscience are provided here courtesy of Society for Neuroscience
Full text links
Read article at publisher's site: https://doi.org/10.1523/jneurosci.18-04-01217.1998
Read article for free, from open access legal sources, via Unpaywall:
https://www.jneurosci.org/content/jneuro/18/4/1217.full.pdf
Free after 6 months at www.jneurosci.org
http://www.jneurosci.org/cgi/content/full/18/4/1217
Free after 6 months at www.jneurosci.org
http://www.jneurosci.org/cgi/reprint/18/4/1217.pdf
Free to read at www.jneurosci.org
http://www.jneurosci.org/cgi/content/abstract/18/4/1217
Citations & impact
Impact metrics
Citations of article over time
Article citations
Rapid sequential clustering of NMDARs, CaMKII, and AMPARs upon activation of NMDARs at developing synapses.
Front Synaptic Neurosci, 16:1291262, 10 Apr 2024
Cited by: 0 articles | PMID: 38660466 | PMCID: PMC11039796
Coordination of Pickpocket ion channel delivery and dendrite growth in Drosophila sensory neurons.
PLoS Genet, 19(11):e1011025, 09 Nov 2023
Cited by: 1 article | PMID: 37943859 | PMCID: PMC10662761
Prolonged STAT1 activation in neurons drives a pathological transcriptional response.
J Neuroimmunol, 382:578168, 02 Aug 2023
Cited by: 6 articles | PMID: 37556887 | PMCID: PMC10527980
Molecular basis of interactions between CaMKII and α-actinin-2 that underlie dendritic spine enlargement.
Elife, 12:e85008, 25 Jul 2023
Cited by: 1 article | PMID: 37489746 | PMCID: PMC10484527
SAPAP Scaffold Proteins: From Synaptic Function to Neuropsychiatric Disorders.
Cells, 11(23):3815, 28 Nov 2022
Cited by: 6 articles | PMID: 36497075 | PMCID: PMC9740047
Review Free full text in Europe PMC
Go to all (294) article citations
Similar Articles
To arrive at the top five similar articles we use a word-weighted algorithm to compare words from the Title and Abstract of each citation.
Role of actin in anchoring postsynaptic receptors in cultured hippocampal neurons: differential attachment of NMDA versus AMPA receptors.
J Neurosci, 18(7):2423-2436, 01 Apr 1998
Cited by: 400 articles | PMID: 9502803 | PMCID: PMC6793094
Mismatched appositions of presynaptic and postsynaptic components in isolated hippocampal neurons.
J Neurosci, 20(22):8344-8353, 01 Nov 2000
Cited by: 98 articles | PMID: 11069941 | PMCID: PMC6773189
A functional role of postsynaptic density-95-guanylate kinase-associated protein complex in regulating Shank assembly and stability to synapses.
J Neurosci, 24(42):9391-9404, 01 Oct 2004
Cited by: 59 articles | PMID: 15496675 | PMCID: PMC6730104
PSD-95-like membrane associated guanylate kinases (PSD-MAGUKs) and synaptic plasticity.
Curr Opin Neurobiol, 21(2):306-312, 28 Mar 2011
Cited by: 115 articles | PMID: 21450454 | PMCID: PMC3138136
Review Free full text in Europe PMC
Funding
Funders who supported this work.
NINDS NIH HHS (2)
Grant ID: R01 NS033184
Grant ID: NS33184