Abstract
Free full text

Sphingomonas alaskensis Strain AFO1, an Abundant Oligotrophic Ultramicrobacterium from the North Pacific
Abstract
Numerous studies have established the importance of picoplankton (microorganisms of ≤2 μm in length) in energy flow and nutrient cycling in marine oligotrophic environments, and significant effort has been directed at identifying and isolating heterotrophic picoplankton from the world's oceans. Using a method of diluting natural seawater to extinction followed by monthly subculturing for 12 months, a bacterium was isolated that was able to form colonies on solid medium. The strain was isolated from a 105 dilution of seawater where the standing bacterial count was 3.1 × 105 cells ml−1. This indicated that the isolate was representative of the most abundant bacteria at the sampling site, 1.5 km from Cape Muroto, Japan. The bacterium was characterized and found to be ultramicrosized (less than 0.1 μm3), and the size varied to only a small degree when the cells were starved or grown in rich media. A detailed molecular (16S rRNA sequence, DNA-DNA hybridization, G+C mol%, genome size), chemotaxonomic (lipid analysis, morphology), and physiological (resistance to hydrogen peroxide, heat, and ethanol) characterization of the bacterium revealed that it was a strain of Sphingomonas alaskensis. The type strain, RB2256, was previously isolated from Resurrection Bay, Alaska, and similar isolates have been obtained from the North Sea. The isolation of this species over an extended period, its high abundance at the time of sampling, and its geographical distribution indicate that it has the capacity to proliferate in ocean waters and is therefore likely to be an important contributor in terms of biomass and nutrient cycling in marine environments.
During the last century, a significant effort has been directed at determining the microbial community composition in oligotrophic marine waters. A key issue is the determination of which microbial species are numerically dominant in natural oligotrophic marine environments. An equally important and independent issue is the determination of which species make the greatest contribution to the biological cycling of carbon, nitrogen, and phosphorus. In the last few years these questions have been addressed using a variety of molecular (15, 21, 22, 68, 80), microscropic (3, 16, 30, 38, 48), flow cytometric (1, 11, 20, 27, 28), and cultivation (1, 2, 20, 21, 50, 73) techniques. Many of the conclusions of these studies are incongruent, due in part to the different methods employed by these investigators, variations in the application of similar methods, or inherent limitations of a method. Superimposed on methodological constraints are also sampling variabilities caused by differences in geography, season, diel cycling (13, 21, 37, 50), and predation (11, 13, 31, 53, 58, 59).
Despite difficulties in obtaining quantitative measurements in situ, it is clear from microscopy, flow cytometry and filtration studies that small cells, “ultramicrocells” (volume of less than 0.1 μm3) are prevalent (105 to 106 cells ml−1) in oligotrophic marine waters (reviewed in reference 63). An important question concerning the ultramicrocells is whether they represent a class of microorganism that maintain their ultramicrocell-volume independently of growth status (e.g., growing or starved) or whether they are capable of becoming larger. Related to this is also the question of whether the ultramicrocells are metabolically active and contributing to mineralization or whether they are largely dormant. One approach to answering these questions is to perform physiological studies on isolates which are abundant in oligotrophic waters. A comprehensive understanding of metabolism will enable rationalized testing in situ and environmental modeling (e.g., biological productivity and nutrient flux rates). In addition, the combination of physiology and tangible genetic markers will enable an ecological assessment of cellular markers (e.g., monitoring specific gene expression) to test and augment mathematical models.
The extinction dilution method has proven useful for isolating ultramicrobacteria from oligotrophic marine waters (10, 60). The method relies on the serial dilution of seawater samples until growth no longer occurs. The tube with the highest dilution that supports growth contains the most abundant species that was able to grow in the medium used. Using this method with filtered autoclaved seawater as diluent, a number of Sphingomonas strains were isolated as numerically dominant isolates from Resurrection Bay, Alaska, and the North Sea (10, 60). All the isolates are ultramicrobacteria and members of a single species, recently defined as Sphingomonas alaskensis, with type strain RB2256 (72). Since its isolation, S. alaskensis RB2256 has been the subject of extensive physiological characterization (19, 23–25, 35, 47, 62). These studies have revealed that its physiology is distinctly different from other heterotrophic bacteria such as Escherichia coli or the marine bacterium Vibrio angustum S14. One of the characteristics of this strain is that it retains a relatively unchanged ultramicrosize (0.03 to 0.07 μm3) when grown in a marine medium with organic carbon concentrations varying between 0.8 and 800 mg of C liter−1 (19). Furthermore, cells do not miniaturize when they are starved for extended periods (61). Strain RB2256 is also able to grow over a wide range of temperatures from above 45°C to below 5°C (19), which would theoretically allow it to colonize temperate as well as Arctic or deep-ocean waters.
To increase the number of ultramicrosized microorganisms that are available for laboratory studies, we used an extinction dilution method to obtain isolates that were abundant in the oligotrophic ocean waters near Japan. One isolate formed colonies on plates after 12 months of serial culturing and produced ultramicrosized cells. In this report we describe the taxonomy of the strain and demonstrate that the new isolate, strain AFO1, is a strain of S. alaskensis. The importance of the isolation procedures for obtaining this species and other abundant ultramicrobacteria is discussed. In addition, the implications of the geographical distribution of this species are addressed.
MATERIALS AND METHODS
Isolation and cultivation of bacteria.
Seawater samples were collected from ocean waters near Cape Muroto in Kochi Prefecture, Japan (station 1; 33°17′05′′N, 134°13′89′′E), and the Pacific Ocean (station 2; 32°49′90′′N, 136°09′74′′E). Stations 1 and 2 were located at the continental shelf (water depth, 550 m) and the ocean basin floor (water depth, 3640 m), respectively. The sampling depths and dates were 350 m on 12 June 1998 at station 1 and 500 m on 4 June 1998 at station 2. All seawater samples were collected in acid-washed and thoroughly rinsed Niskin bottles. All samples were stored on board ship at 3°C, and all experimental procedures were started within 5 h after sampling.
The viable heterotrophic bacteria in seawater samples were cultured and isolated by an extinction dilution method (10). Filtered and autoclaved natural seawater (FAS), which contains approximately 0.5 mg of C liter−1, was used as the culture medium. For the preparation of FAS, seawater from each sampling station was filtered through precombusted (550°C) GF/C fiberglass filters (Whatman) and then through 0.22-μm-pore-size nitrocellulose ester membrane filters (Millipore) prior to autoclaving. Seawater samples were diluted up to 107-fold. Culture test tubes contained screw caps with Teflon liners and contained 15 ml of FAS. The test tubes were inoculated with 1 ml of sample that had been diluted to 10−5, 10−6, and 10−7. For each dilution series, 25 culture test tubes were prepared. All the inoculated culture tubes were incubated at 15°C in the dark. After incubation for 1 month, 1-ml samples were withdrawn from each of the 25 culture tubes per dilution series and fixed in 0.5% (wt/vol) glutaraldehyde. The fixed samples were treated with 0.1 % Triton X-100 to improve stain penetration and stained with DAPI (4′, 6-diamidino-2-phenylindole), and bacterial numbers observed with an epifluorescence microscope (Olympus) (52).
When the bacterial count in the culture tube was more than 105 cells ml−1, it was scored as having growth (18). When the bacterial counts were less than 104 cells ml−1, it was recorded as having no growth. To determine whether cells were able to grow on nutrient-rich media, a 0.1-ml sample was taken from the tubes which supported growth, plated onto a complex peptone-based agar, VNSS (19), and plates incubated at 15°C for 14 days. Cells which had grown on VNSS were purified by restreaking on VNSS agar. Cultures from tubes which supported growth of more than 105 cells ml−1 but did not produce colonies on VNSS agar were transferred (1 ml) to freshly prepared FAS (15 ml) and incubated for an additional 1 month at 15°C. Every month (for a total period of 1 year), this procedure was repeated by calculating culture densities with DAPI-stained cells and transferring cultures to VNSS agar or fresh FAS medium. To avoid contamination, subculturing was performed in a laminar-flow hood using a 0.22-μm-pore-size nitrocellulose ester membrane filter.
S. alaskensis RB2256 and strain AFO1 (this work) were maintained on artificial seawater medium supplemented with 3 mM glucose or a peptone-based complex medium, VNSS (19); S. macrogoltabidus ATCC 51380 was maintained on R2A agar (Oxoid); Sphingomonas strain KT-1 (69) was maintained on 3.0 g of yeast extract liter−1–5.0 g of peptone liter−1–0.75% NaCl (YEPS); and Halomonas subglaciescola ACAM 21 was maintained on marine broth 2216 (Difco). For preparation of DNA and fatty acids, S. alaskensis RB2256, strain AFO1, and H. subglaciescola were grown in marine broth 2216, KT-1 was grown in YEPS, and S. marcrogoltabidus was grown in R2A medium at 25°C and cells were harvested at late logarithmic phase (optical density at 610 nm, 0.5).
Morphology.
Atomic force microscopy (AFM) using a SPM-9500 J2 microscope (Shimadzu Co. Ltd.) was used for examining morphology and determining the size parameters of strain AFO1. Since its invention in 1986, AFM has been increasingly used to examine bacterial morphology due to the resolution that may be achieved to the molecular level, the ability to obtain information about three-dimensional structures, and the cost-effectiveness in comparison to electron microscopy (6). AFM images are not affected by grey zones that may surround fluorescing cells in epifluorescence microscopy (39), thereby potentially increasing the accuracy of the size measurements. Cells were grown to logarithmic phase or subjected to 24-h starvation in a complex medium (VNSS) or a modified marine minimal medium (MMMM) consisting of 0.2 % (wt/vol) glucose, 9.52 mM NH4Cl, 1.32 mM K2HPO4, 0.01 mM FeSO4 · 7H2O, 4 mM Tricine, 40 mM morpholinepropanesulfonic acid (MOPS), and 0.05 μg of vitamin B12 per liter of nine-salts solution (NSS [pH 8.2]). Cells were fixed with 0.5% glutaraldehyde and washed twice with distilled water that had been prefiltered through a 0.2-μm-pore-size Isopore filter (Millipore), to remove salt and residual organic matter. The cells were concentrated on a Isopore filter (0.2 μm) before microscopy was performed. The microscope was equipped with microfabricated and oxid-sharpened Si3N4 cantilevers (OMCL-AC160TS-C1; Olympus Co. Ltd.) with a pyramidal tip and using a force of 42 N/m. Scanning images were captured using a dynamic mode. For calibration, a Fluoresbrite calibration size kit (Polysciences, Inc.), containing particles 0.126, 0.5, and 1.0 mm in diameter, was used. The image analysis system supplied by Shimadzu Co. Ltd. was used to measure cell length (l), width (w), and height (h), directly from the image. Cell volume was calculated from length and width measurements using the following formula: volume = (π/4)w2(l − w/3) (45).
Motility.
Strain AFO-1 was cultured at room temperature in one-quarter-strength ZoBell 2216E medium. Exponentially growing cells were harvested and washed with fresh medium. A drop of cell suspension was placed between a microscope slide and coverglass. The swimming behavior of the bacteria was observed using an Olympus BH-2 microscope.
Stress exposure protocols.
Stress experiments were performed essentially as described by Eguchi et al. (19). Stress resistance for strain AFO1 was assessed by recording the number of CFU remaining on VNSS agar following heat stress (shift of culture temperature from 20 to 56°C), ethanol stress (20% [vol/vol] ethanol) or hydrogen peroxide stress (25 mM H2O2; freshly prepared from a 30% commercial stock solution). Ethanol and hydrogen peroxide stress experiments were performed at 20°C. Stress resistance was determined for cells grown in VNSS to logarithmic phase or following starvation. Starved cells were prepared by harvesting logarithmic-phase cells and resuspending them in the mineral salts solution (NSS) (19) for up to 168 h.
Fatty acid analysis.
For fatty acid analysis, Sphingomonas strains were grown at 25°C. Lipids were extracted using the modified one-phase chloroform-methanol Bligh and Dyer extraction method (4, 76). A portion of the total lipid extract was transesterified by reaction at 80°C for 1 h using 3 ml of a methanol-chloroform-hydrochloric acid (10:1:1 [vol/vol/vol]) solution. After the addition of water, the mixture was extracted with hexane-chloroform (4:1 [vol/vol]) to yield fatty acid methyl esters (FAME). Hydroxy functionalities were converted to trimethylsilyl ethers by reaction with bis(trimethylsilyl)trifluoroacetamide (BSTFA) reagent at 80°C for 24 h.
FAME were analyzed using a Hewlett-Packard 5890 II gas chromatograph and 5970A mass-selective detector equipped with a 50-m by 0.22-mm (internal diameter) cross-linked methyl silicone (0.33 μm film thickness) fused-silica capillary column. The operating conditions were similar to those detailed by Nichols et al. (43). Identification of FAME from all samples was achieved by interpretation of component spectra and comparison to those of known standards.
Monounsaturated fatty acid double-bond position and geometry were determined by gas chromatography-mass spectrometry analysis of their dimethyl disulfide adducts (44).
Genome size.
For the preparation of DNA to determine genome size by pulsed-field gel electrophoresis (PFGE), S. alaskensis RB2256 and strain AFO1 were grown in artificial seawater–3 mM glucose, harvested at late logarithmic phase (optical density at 410 nm, 0.7), and washed once with TE buffer (10 mM Tris [pH 8.0], 1 mM EDTA [pH 8.0]). The cells were concentrated by centrifugation to approximately 2 × 109 cells ml−1 in TE buffer and embedded in 1% low-melting-point (LMP) agarose using plug molds (Bio-Rad). The 1% LMP agarose was prepared in 10 mM Tris–10 mM EDTA and kept at 45°C prior to plug assembly. The plugs were made by mixing cell suspensions with the LMP agarose (1:1) to give a final concentration of 109 cells ml−1 in the agarose plug. Cell lysis was performed by incubating the plugs in lysozyme solution (2 mg of lysozyme ml−1, 1% N-lauroylsarcosine, 50 mM Tris [pH 8.0], 50 mM EDTA [pH 8.0]) overnight at 37°C. The lysis buffer was replaced with a proteinase K solution (1 mg of proteinase K [Boehringer Mannheim] ml−1, 1% sodium dodecyl sulfate [SDS], 50 mM Tris [pH 8.0], 50 mM EDTA [pH 8.0]), and the plugs were incubated at 42°C overnight. Overnight incubation was sufficient, although it was noted that incubations for up to 2 days did not result in nonspecific DNA degradation. The proteinase K solution was removed, and the plugs were sequentially washed with 10 ml of sterile water for 5 min, 10 ml of TE buffer for 5 min, and four times with 10 ml TE buffer for 30 min each. Following the washes, the plugs could be stored in TE buffer at 4°C.
Before restriction enzymes were used to digest the DNA, the plugs were sliced in half and sections were washed three times with 200 μl of ice-cold sterile water for 30 min each and then incubated in 100 μl of 1× restriction enzyme buffer on ice for 30 min. The DNA was digested by the addition of 60 U of restriction enzyme prepared in 100 μl of fresh restriction enzyme buffer and incubation overnight at the appropriate temperature for the enzyme.
Electrophoresis was performed on a CHEF-drive II apparatus (Bio-Rad) using 1% pulsed-field-certified agarose (Bio-Rad) with 2 liters of 0.5× Tris-borate-EDTA (TBE) running buffer at 14°C with a pump flow rate of 0.7 liter min−1. Size markers used were yeast DNA (225 to 1,900 kbp), a lambda phage DNA ladder (48.5 to 727.5 kbp), and a low-range lambda PFG marker (2.03 to 194.0 kbp) from New England Biolabs, Inc. Following electrophoresis, the gels were stained for 30 min in 500 ml of sterile distilled water containing 50 μl of ethidium bromide (10 mg ml−1) and destained three times in 1 liter of distilled water for 30 min each. The gels were photographed with a GelDoc instrument (Bio-Rad), and images were analyzed with QuantityOne software (Bio-Rad).
To obtain optimal separation and sizing of bands, switch time, voltage setting (4.5 and 5.5 V cm−1), running time (15 to 28.5 h), and the type of size markers were varied. Switch times were chosen to ensure overlap between runs, with a total of three and six different switch times used to separate SpeI and XbaI digests, respectively. This approach enabled simultaneous fragment sizing, minimized the variations between replicate runs, and increased the precision of fragment sizing.
DNA extraction.
Total genomic DNA was extracted using a modification of the method described by Murray and Thompson (40). A 2-ml aliquot of late-logarithmic-phase cells was pelleted by centrifugation, the medium was decanted, and the pellet was resuspended in 567 μl of TE. Cells were lysed by the addition of 30 μl of 10% (wt/vol) SDS and 3.0 μl of 20 mg of proteinase K per ml to give final concentrations of 100 μg of proteinase K per ml and 0.5% (wt/vol) SDS. The solution was mixed thoroughly and incubated at 60°C for 4 h before the addition of 100 μl of 5M NaCl and 80 μl of 10% (wt/vol) CTAB (hexadecyltrimethylammonium bromide [Sigma]) in 0.7% (wt/vol) NaCl. The CTAB-NaCl solution was prepared by slow addition of CTAB (10 g) to 100 ml of 0.7 M NaCl while heating and stirring.
Samples were mixed thoroughly and incubated at 65°C for 10 min. CTAB complexes were extracted with 1 volume of chloroform-isoamyl alcohol (24:1 [vol/vol]) and centrifugation at 12,000 × g for 5 min, and the supernatant was transferred to a fresh tube. Any CTAB complexes remaining in the supernatant were extracted with 1 volume of phenol-chloroform-isoamyl alcohol (25:24:1 [vol/vol/vol]) and centrifugation at 12,000 × g for 5 min. The supernatant was transferred to a fresh tube, and nucleic acids were precipitated by the addition of 0.6 volume of isopropanol. After the contents of the tubes were mixed by gentle inversion, the nucleic acids were collected by spooling on a glass rod and washed successively in 50, 70, and 100% (vol/vol) ethanol. Spooled and washed DNA was transferred to a fresh tube, dried briefly in vacuo, and resuspended in deionized water. The DNA was subsequently used for 16S rRNA gene amplification, DNA-DNA hybridization, and moles percent G+C content analysis.
Phylogenetic analysis of 16S rRNA sequences.
Amplification of the almost full-length 16S rRNA gene from AFO1 was performed by PCR using the bacterial consensus 16S rRNA primers 27F and 1494R (41). The PCR mixture (20 μl) contained 25 to 50 ng of DNA, 0.2 mM each deoxynucleoside triphosphate, 0.2 pM each primer, and 2.5 mM MgCl2. After an initial denaturation step of 5 min at 95°C, the temperature of the PCR mixture was lowered to 88°C and 1 to 2 U of Taq DNA polymerase (Sigma) was added. Thermal cycling was performed in a PCR Sprint temperature-cycling system (Hybaid Ltd.) for 25 cycles of denaturation for 20 s at 95°C, annealing for 20 s at 50°C, and extension for 1.5 min at 72°C. The final extension step was 5 min at 72°C followed by storage at 4°C.
PCR products were prepared for DNA sequencing by ethanol precipitation. After the addition of 0.1 volume of 3 M sodium acetate and 2 volume of 80% (vol/vol) ethanol, the tubes were vortexed for 20 s and incubated at 22°C for 5 min. The DNA was collected by centrifugation at 12 000 × g for 10 min at 22°C, the supernatant was discarded, and the pellet was resuspended in deionized water. DNA sequencing was performed using the PRISM Big Dye cycle-sequencing system (Applied Biosystems Inc.) and primers 27F, 519R, 530F, 929R, 1114F, 1221R, and 1494R (41) as specified by the manufacturer. Sequencing-reaction products were purified by ethanol precipitation, and sequence data were collected from a model 373 sequencer (Applied Biosystems).
DNA sequences corresponding to E. coli 16S rRNA gene positions 27 to 1433 were aligned using the programs Pileup and ClustalX (71). The nucleotide alignments were edited by hand to resolve positions with ambiguities or gaps. The 16S rDNA distance trees were reconstructed using the neighbor-joining method with Jukes-Cantor corrections (57) as implemented by ClustalX. The bootstrap confidence levels for the interior branches of the trees were estimated from 1,000 resamplings of the data (26).
DNA-DNA hybridisation and moles percent G+C content.
The spectrophotometric renaturation rate kinetic procedure adapted by Bowman et al. (5) from Huss et al. (32) was used to determine DNA-DNA reassociation values between genomic DNA of different strains. Briefly, genomic DNA was sheared to an average size of 1 kb using sonication, dialyzed overnight at 4°C in 2× SSC buffer (0.3 M NaCl, 0.03 M sodium citrate [pH 7.0]), and adjusted in concentration to approximately 60 to 75 μg ml−1. Following denaturation of the DNA samples, hybridization was performed at the optimal temperature for renaturation (TOR), which was 25°C below the DNA melting temperature and was calculated from the following equation: TOR = 48.5°C + (0.41 × %G+C)°C. The decline in absorbance of DNA mixtures and control DNA samples over a 40-min interval was used to calculate DNA hybridization values from the following equation: % DNA hybridization = [(4AB − A − B)/2√(A × B)] × 100%, where A and B represents the change in absorbance for two DNA samples being compared and AB represents the change in absorbance for equimolar mixtures of A and B. DNA hybridization values equal to or below 25% are considered to represent background hybridization and are thus not considered significant.
The DNA base composition of strains was determined using the spectrophotometric thermal denaturation method described by Sly et al. (64).
Nucleic acid accession number.
The AFO1 16S rRNA sequences were deposited in the GenBank database under accession number AF378796. To resolve a number of minor differences and unassigned nucleotides in data entries (Z73631 and AF148812) for the 16S rRNA gene of S. alaskensis RB2256, we sequenced the gene and obtained unambiguous sequence data. This sequence (AF378795) supersedes the older entries and should be used in place of any others in the database.
RESULTS
Bacterial numbers at the sampling sites.
The number of microorganisms present at the sampling sites was estimated from microscopy counts of DAPI-stained cells, CFU on VNSS agar, and most probable numbers MPN (extinction dilution) with VNSS and FAS liquid media (Table (Table1).1). The microbial counts on VNSS agar were 2 to 3 orders of magnitude lower than the direct microscopy counts. Based on the number of cells obtained from direct counts, the standing microbial count at the time of sampling was 3.1 × 105 and 5.5 × 105 cells ml−1 at stations 1 and 2, respectively.
TABLE 1
Number of microorganisms present at the sampling sites determined by direct counts and CFU
Site | No. of cells/ml (103) determined by:
| |||
---|---|---|---|---|
Direct countab | Plate count (VNSS)c | MPN
| ||
VNSSc | FASd | |||
Station 1 | 310![]() ![]() | 0.5![]() ![]() | 3.3 | 24 |
Station 2 | 550![]() ![]() | 9.5![]() ![]() | 92 | 110 |
Isolation of bacteria by extinction dilution.
After a 1-month incubation in FAS at a dilution of 10−5, 24 of 25 samples from station 1 showed positive growth (at least 105 cells ml−1). However, none of the 24 growth-positive cultures from the 10−5 dilution formed colonies on VNSS agar. No growth was observed in any of the tubes diluted 106- or 107-fold. Following reinoculation from the 24 growth-positive cultures into fresh FAS and incubation for a further 1 month, 20 of the cultures supported growth (at least 105 cells ml−1). For a total of 12 months, the samples were recultured each month, and at the end of that period, 14 of the 20 cultures still supported growth in FAS. At the end of the 12-month period, 1 of the 14 cultures produced colonies on the VNSS agar. This isolate was designated AFO1. While the 13 remaining cultures continued to grow in FAS liquid medium, they did not form colonies on VNSS agar.
Following a similar regimen of monthly inoculations in FAS and on VNSS agar (for a total of 12 months), 10 of the 10−5 dilutions and 6 of the 10−6 dilutions of the initial 25 inoculations from station 2, continued to support growth in FAS but not on VNSS agar.
Morphology.
Strain AFO1 formed opaque yellow, low-convex, entire colonies on ASW, VNSS, and marine broth agar. Colonies were visible after 4 days and grew to 1 mm in diameter at 20°C on VNSS agar. The cellular morphology of strain AFO1 was examined by AFM, the cells were oval or rod shaped, and flagella were not detected. Lack of flagella was consistent with the appearance of nonmotile cells in a hanging drop. Cell dimensions and volumes were calculated for strain AFO1 from measurements (Table (Table2)2) taken from AFM images. In a defined medium (MMMM), the volume of growing cells was ~0.05 μm3 and was reduced approximately twofold after 24 h of starvation. Further size reduction was not observed after 168 h of starvation (data not shown). In a complex medium (VNSS), cell volumes were approximately twice those observed in the defined medium.
TABLE 2
Size and volume measurements for strain AFO1
Medium | Growth phase | Length (μm)a | Width (μm)a | Vol (μm3)a |
---|---|---|---|---|
Defined MMMM | Exponential | 0.837 | 0.294 | 0.050 |
Stationary | 0.522 | 0.266 | 0.024 | |
Complex VNSS | Exponential | 1.007 | 0.452 | 0.138 |
Stationary | 0.607 | 0.394 | 0.058 |
S. alaskensis RB2256 and strain AFO1 were grown at various temperatures in a range of growth media in batch and under nutrient-limiting conditions in a chemostat, and cellular morphology was examined by phase-contrast and transmission electron microscopy (data not shown). Under all growth conditions examined, cells were predominantly single regular rods; however, chains or filaments up to 70 μm in length, with septa separating individual cells, were observed.
Phylogeny.
The 16S rRNA sequence from strain AFO1 was compared with sequences on the 16S rRNA database, and the most closely related, full-length sequences were identified and used for constructing phylogenetic trees. Tree topology was similar for parsimony and distance matrix trees (data not shown), and a representative neighbor-joining tree is shown (Fig. (Fig.1).1). From the edited alignments used for tree construction, only one nucleotide difference was present between strain AFO1 and RB2256 (1,406 bp). S. alaskensis strains RB2510 and 2515, which were isolated from Resurrection Bay (73), had 1 and 2 nucleotide differences and four alignment gaps compared with the sequence for strain RB2256, respectively. In view of the errors for 16S rRNA sequences for strain RB2256 (accession numbers Z73631 and AF148812), these differences may also be sequencing artifacts.
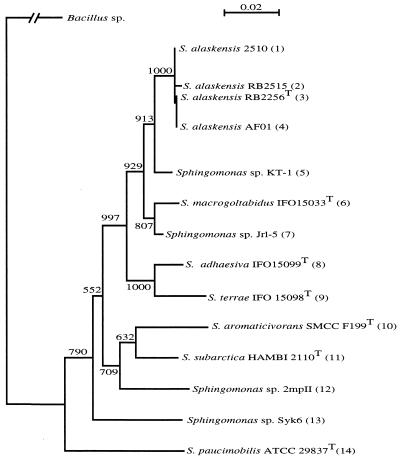
Distance matrix tree of selected Sphingomonas 16S rDNA sequences. DNA sequences corresponding to the E. coli 16S rRNA gene positions 27 to 1433 were aligned using the programs PILEUP and ClustalX (71). Genetic distances were calculated using the method of Jukes and Cantor, and the phylogenetic tree was reconstructed using the neighbor-joining algorithm of Saitou and Nei (57) as implemented within ClustalX. The phylogenetic tree was plotted using the program nj-plot. The root of the tree was determined using the 16S rRNA gene of Bacillus (BCE277907) as an outgroup. Numbers on the branches represent bootstrap values for 1,000 repeats (26). The bar indicates 2 nucleotide changes per 100. Accession numbers: 1, AF145754; 2, AF145753; 3, AF378795; 4, AF378796; 5, AB022601; 6, D13723; 7, AF181572; 8, D17322; 9, D13727; 10, U20756; 11, X94102; 12, U90216; 13, D16149; and 14, U37337.
The most similar sequence to those in the S. alaskensis cluster is from strain KT-1 (Fig. (Fig.1),1), which has 19 nucleotide differences. The sequence of an isolate from a hot spring (AB015049) was also very similar to that of AFO1 (98.1% identity, 1,311 identities in 1,338 residues, no gaps); however, it was not included in the tree because the sequence was short (1,338 bp compared with 1,406 bp).
DNA base composition and DNA-DNA hybridization.
The G+C content for DNA from strains RB2256, AFO1, and KT-1 was 64.8, 65.1, and 65.3 mol% G+C respectively (Table (Table3).3). The DNA-DNA reassociation level between genomic DNA from strains RB2256 and AFO1 averaged 84%, while that between strains RB2256 and KT-1 DNA hybridization levels averaged only 34% (Table (Table3).3). S. macrogoltabidus ATCC 51380 (65 mol% G+C) and H. subglaciescola ACAM 21 (59 mol% G+C) were used as control strains, and both exhibited background renaturation levels of 12 to 23% with strain RB2556. S. macrogoltabidus exhibited significant levels of hybridization (44%) with strain KT-1, however, they were below the level indicative of a bacterial species (65, 75). This indicates that strain KT-1 is closely allied to the species S. macrogoltabidus but appears to represent a novel species.
TABLE 3
DNA base composition and DNA-DNA hybridization values between seawater isolates and S. alaskensis RB2256, strains AFO1 and KT-1, and S. macrogoltabidus
Strain | Mol% G+C (Tm) | % Hybridization for:
| |
---|---|---|---|
RB2556 | KT-1 | ||
S. alaskensis RB22556T | 64.8 | 100 | |
AFO1 | 65.1 | 84 | |
KT-1 | 65.3 | 34 | 100 |
S. macrogoltabidus ATCC 51380 | 65.4 | 23 | 44 |
Genome size.
The restriction enzymes NotI and SfiI (45 to 60 mol% G+C), XbaI and SpeI (50 to 70 mol% G+C), and DraI and SspI (60 to 70 mol% G+C), which have previously been used for PFGE of high-G+C DNA, were tested with genomic DNA from S. alaskensis RB2256 and strain AFO1. Both SpeI (recognition sequence, 5′-ACTAGT-3′) and XbaI (recognition sequence, 5′-TCTAGA-3′) produced suitable profiles for genome sizing, while the other four restriction enzymes produced numerous fragments of less than 100 kb and as a result were not used for DNA digestion. No differences in genome-banding patterns were observed between strain AFO1 and S. alaskensis RB2256, and a summary of band sizes and calculated genome sizes is shown in Table Table4.4. A total of 26 fragments with sizes from 7 to 670 kp were obtained from an XbaI digest of S. alaskensis RB2256 DNA, and 10 fragments ranging in size from 33 to 1,017 kb were obtained for SpeI digests. The number of comigrating fragments was calculated from a linear-regression plot of DNA size versus band intensity (measured as optical density). Four fragments comigrated at 37.3 kb, and two fragments comigrated at 88.7 kb (Table (Table4).4). Based on the sizes of all fragments, the genome size range for strain AFO1 and S. alaskensis RB2256 was estimated as 3,118 kb (SpeI) to 3,250 kb (XbaI).
TABLE 4
Size of restriction fragments generated by XbaI and SpeI cleavage of S. alaskensis RB2256 and strain AFO1 genomic DNAa
XbaIb
| SpeI
| ||
---|---|---|---|
Fragment | Size (kbp) | Fragment | Size (kbp) |
1 | 669.7 | 1 | 1,016.9 |
2 | 403.2 | 2 | 505.8 |
3 | 251.8 | 3 | 345.2 |
4 | 233.8 | 4 | 257.5 |
5 | 204.6 | 5 | 239.4 |
6 | 179.0 | 6 | 231.0 |
7 | 163.0 | 7 | 175.4 |
8 | 142.3 | 8 | 165.7 |
9 | 123.3 | 9 | 148.1 |
10 | 93.7 | 10 | 32.7 |
11 | 88.7![]() ![]() | ||
12 | 78.0 | ||
13 | 74.7 | ||
14 | 63.4 | ||
15 | 55.9 | ||
16 | 37.3![]() ![]() ![]() ![]() | ||
17 | 33.5 | ||
18 | 25.3 | ||
19 | 23.8 | ||
20 | 22.0 | ||
21 | 21.0 | ||
22 | 17.3 | ||
23 | 16.0 | ||
24 | 13.0 | ||
25 | 7.7 | ||
26 | 7.0 |
Fatty acids.
The fatty acid composition of Sphingomonas sp. strains KT-1 and AFO1 compared to S. alaskensis RB2256 is shown in Table Table5.5. Variability between replicate analyses of samples was low. The two marine strains (AFO1 and S. alaskensis RB2256) possessed similar fatty acid profiles (the major components were 17:1ω6c, 18:1ω7c, and 17:1ω8c) and were clearly distinguished from the freshwater species KT-1 (containing 18:1ω7c and 16:1ω7c as the major components). Two 19:1 isomers (19:1ω8c and 19:1ω6c) were identified from AFO1 and S. alaskensis by analysis of dimethyl disulfide adducts. A trace amount of a br18:1ω6c was also detected by single-ion monitoring analysis, which coeluted with 2-OH16:0. The presence of 19:1ω8c and 19:1ω6c together with the series of monounsaturated 2-OH fatty acids detected in this study may act as markers for these species. The fatty acid profiles determined support the phylogenetic relationship between KT-1, AFO1, and S. alaskensis as described by the 16S rRNA sequence (Fig. (Fig.1).1).
TABLE 5
FAME analysis of fatty acids from S. alaskensis RB2256 and Sphingomonas strains AFO1 and KT-1
Fatty acid | Composition (%)a in:
| ||
---|---|---|---|
Sphingomonas sp. KT01 | Sphingomonas sp. AFO1 | Sphingomonas alaskensis RB2256 | |
Saturated | |||
![]() | 0.5![]() ![]() | ND | ND |
![]() | 0.2![]() ![]() | 1.9![]() ![]() | 2.0![]() ![]() |
![]() | 3.4![]() ![]() | 3.6![]() ![]() | 3.2![]() ![]() |
![]() | 3.2![]() ![]() | 4.0![]() ![]() | |
![]() | tr![]() ![]() | 0.4![]() ![]() | 0.9![]() ![]() |
![]() | 4.1![]() ![]() | 9.1![]() ![]() | 10.1![]() ![]() |
Branched | |||
![]() | ND | ND | 0.3![]() ![]() |
![]() | ND | ND | 0.3![]() ![]() |
Unsaturated | |||
![]() | 0.4![]() ![]() | ND | ND |
![]() | 0.2![]() ![]() | 0.2![]() ![]() | |
![]() | 34.2![]() ![]() | 4.3![]() ![]() | 3.8![]() ![]() |
![]() | 3.1![]() ![]() | 0.8![]() ![]() | 0.7![]() ![]() |
![]() | 0.3![]() ![]() | 8.1![]() ![]() | 10.0![]() ![]() |
![]() | 1.9![]() ![]() | 35.8![]() ![]() | 44.7![]() ![]() |
![]() | 42.6![]() ![]() | 24.3![]() ![]() | 20.1![]() ![]() |
![]() | 1.2![]() ![]() | 3.8![]() ![]() | 0.9![]() ![]() |
![]() | ND | 0.9![]() ![]() | 1.0![]() ![]() |
![]() | ND | 1.2![]() ![]() | 1.3![]() ![]() |
![]() | 83.6![]() ![]() | 79.3![]() ![]() | 82.7![]() ![]() |
Hydroxy | |||
![]() | 3.8![]() ![]() | 1.2![]() ![]() | 0.6![]() ![]() |
![]() | ND | 5.1![]() ![]() | 3.5![]() ![]() |
![]() | 0.3![]() ![]() | 0.4![]() ![]() | 0.1![]() ![]() |
![]() | 7.6![]() ![]() | 3.1![]() ![]() | 1.5![]() ![]() |
![]() | 0.3![]() ![]() | 1.8![]() ![]() | 1.2![]() ![]() |
![]() | 0.4![]() ![]() | ND | tr![]() ![]() |
![]() | 12.4![]() ![]() | 11.6![]() ![]() | 6.9![]() ![]() |
Total | 100.0![]() ![]() | 100.0![]() ![]() | 100.0![]() ![]() |
Stress responses.
AFO1 cells were examined for their ability to survive the stresses of heat, hydrogen peroxide, and ethanol. Logarithmic-phase and starved cells were sampled at 0, 15, 30, 45, and 60 min after exposure to 56°C, 25 mM hydrogen peroxide, or 20 % ethanol (Fig. (Fig.2).2). Cells were highly resistant to the stresses tested compared to other gram-negative bacteria (33, 34, 46), and the growth phase had little effect on their resistance. Logarithmic-phase and starved cells showed similar levels of resistance to heat stress (Fig. (Fig.2A).2A). A possible effect of growth phase was observed for hydrogen peroxide resistance, with 24- and 168-h (but not 72-h) starved cells appearing marginally more resistant than logarithmic-phase cells (Fig (Fig2B).2B). In repeat experiments similar patterns were observed (data not shown), indicating that starvation does not provide cross-protection to hydrogen peroxide stress in a similar way to that observed in bacteria including E. coli (33), Pseudomonas putida (29), V. angustum S14 (34, 46), Vibrio sp. strain Ant300 (54) and V. anguillarum (42). With the exception of cells which had just been starved, all cultures exhibited similar levels of stress resistance and remained more than 1% viable after a 60-min exposure to 20% ethanol (Fig (Fig2C).2C). For stress experiments, cells were starved by being centrifuged and resuspended in NSS (see Materials and Methods). It is possible that an extracellular component that was present in growing cells was removed during centrifugation and was resynthesized by 24 h of starvation, in time to reestablish stress resistance. By and large, these data indicate that AFO1 cells are inherently resistant to the three stresses tested and that starvation does not induce cross-protection. It is noteworthy that similar patterns of resistance, including the relative sensitivity to ethanol of newly starved cells, are characteristic of S. alaskensis RB2256 (19).
DISCUSSION
S. alaskensis.
The morphology, stress resistance, fatty acid composition, 16S rRNA sequence, genome size, and DNA composition of strain AFO1 are almost identical to those of S. alaskensis RB2256. Of particular note is the 16S rRNA identity (99.9%) and level of genomic DNA-DNA hybridization (greater than 80%). These similarities indicate that strain AFO1 is a strain of S. alaskensis. From a taxonomic standpoint, the new strain AFO1 is not novel; however, the presence of this single species as a numerically significant proportion of the bacteria at geographically remote sampling sites indicates its importance in ocean ecology.
The type strain, S. alaskensis RB2256, and at least six other strains were isolated from Resurrection Bay in Alaska (60, 72), and morphologically and physiologically similar strains were isolated from the North Sea (60, 63). The presence of this species at these locations was further demonstrated by the hybridization of species-specific probes FP1 and FP4 to eight isolates from Ressurection Bay and one isolate (strain NS1619) from the North Sea (59a). In addition, more than 2 years after the initial isolation of S. alaskensis strains from Resurrection Bay, the cells were again detected using Southern hybridization of extracted community DNA using the FP1 probe (Schut, personal communication). This study demonstrates that S. alaskensis (strain AFO1) was also abundant in ocean waters 2.5 km from Cape Muroto.
Strains AFO1 and RB2256 were isolated from depths of 350 and 10 m (10, 60), respectively. At the sampling site for AFO1, an upwelling current carries water from depths of 500 to 1,000 m towards the surface (71). Deep-ocean, surface water, and intermediate-level currents (e.g., the North Pacific Intermediate Water current) move water through the locations near Alaska and Japan where the two strains were isolated (78). It is conceivable that the North Pacific currents are responsible for the distribution of these strains at locations approximately 10,000 km apart. The isolation of this species over a period spanning about a decade, its abundance at the time of sampling, and its geographical distribution indicate that it has the capacity to proliferate in ocean waters and is therefore likely to be an important contributor in terms of biomass and nutrient cycling in marine environments.
A range of isolates of the Sphingomonas genus have been isolated from oligotrophic and eutrophic marine environments, ranging from polar to temperate waters, including coral pathogens and hydrocarbon degraders (14). A number of strains have also been isolated from costal waters in the Baltic Sea (50, 51), some of which were abundant (e.g., BAL46 [51]), particularly during the low-nutrient summer months (50). Pinhassi and Hagstrom (50) noted that despite the prevalence of Sphingomonas isolates in their studies, there were no previous reports of this genus from the Baltic Sea. This may also reflect the name change for the genus Sphingomonas, which was described as Pseudomonas prior to 1990 (77). Reports describing various levels of abundance of Sphingomonas spp. have also appeared for the North Sea. Schut et al. (60) reported the isolation of sphingomonads, including S. alaskensis, with abundances of 15 to 35%, whereas Eilers et al. (21) identified three sphingomonads from the North Sea, but none were in abundance.
Genome.
Using flow cytometry, the DNA content of S. alaskensis RB2256 was initially estimated to be 1.0 to 1.5 fg cell−1 (60), which equates to less than 2 Mb. Using a modified DAPI-DNA fluorometry method (55), the genome size was recently estimated to be 3.6 Mb (3.96 fg of DNA cell−1) (8) and 3.9 Mb (4.2 fg cell−1) (9). Our analysis using PFGE of restriction enzyme fragments of genomic DNA indicates that the genome sizes of strains RB2256 and AFO1 are 3.1 to 3.2 Mb. It is not clear why the estimations of genome size using flow cytometry differ from those obtained by PFGE. Minor inaccuracies in chromosome copy number or corrections used to account for the AT bias of the DAPI stain could produce errors when flow cytometry is used. The genome size for S. alaskensis is consistent with an estimated 3.8 Mb for S. aromaticivorans (preliminary sequence data were obtained for the DOE Joint Genome Institute at http://www.jgi.doe.gov/tempweb/JGI_microbial/html/index.html) and with those of other free-living alpha proteobacteria, including Rhodobacter capsulatus, Caulobacter crescentus, and Rhizobium meliloti, which are 3.4 to 4.0 Mb (described in reference 67).
A small genome may be advantageous for adaptation to oligotrophic marine environments, as has been suggested for the phototrophic cyanobacterium, Prochlorococcus marinus, which has a genome of 1.8 Mb (66). The somewhat larger genome of S. alaskensis indicates that the genome size per se is not the only cellular characteristic that is subject to evolutionary selection pressures in order for microorganisms to become abundant in oligotrophic environments. The potential burden of a larger genome may be offset by gains in cellular functions. An example of this may be the ability to withstand high levels of stress-inducing agents (e.g., hydrogen peroxide) (Fig. (Fig.2)2) (19, 47), which may enhance survival in the environment and which presumably requires a significant genetic complement.
Fatty acids.
Sphingomonas species are characterized by the presence of 2-OH14:0 (79). In addition to this component, a series of further 2-OH fatty acids were identified from strains KT-1, AFO1, and RB2256 in this study. Of particular interest were a series of monounsaturated 2-OH fatty acids which have not previously been reported from Sphingomonas species, including the recent description of S. alaskensis (7, 36, 72). Busse et al. (7) highlighted the apparent disparity of reported fatty acid compositions for individual Sphingomonas species, attributing this phenomenon to differences in the growth media used in the different studies. There is a noticeable difference in the ratio of 17:1ω6c to 18:1ω7c between our data and those previously reported for S. alaskensis (72), and it is likely that this does relate to differences in culture conditions.
Reports of fatty acid composition by MIDI System software of several Sphingomonas species, including S. alaskensis, also reported the presence of two unresolved “summed features,” in some cases accounting for sizeable proportions of the fatty acid composition (7, 36, 72). This study identified 16:1ω7c as the sole component of “summed feature 4” previously reported for S. alaskensis. In addition, a trace amount of br18:1ω6c was detected by single-ion monitoring and may represent the previously reported “18:1ω7c, 11 methyl” component from S. alaskensis (72). However, the recent description of Sphingomonas (Pseudomonas) echinoides confirmed the presence of 11-Me-18:1ω7c from this species by independent methods (56).
This is the first report of 19:1ω8c, 19:1ω6c, and 2-OH monounsaturated fatty acids from a member of the genus Sphingomonas. These components may represent potential biomarkers for the presence of nonculturable S. alaskensis strains in the pelagic environment.
Ultramicrobacteria.
S. alaskensis AFO1 has an ultramicro size (Table (Table2).2). This characteristic is shared by all members of this species. Growing cells exhibit only minor variations in their size, including growth throughout a 1,000-fold variation in medium richness (19). Even under starvation conditions, the reduction in cell size was limited to about twofold (Table (Table2).2). An apparent increase in cell volume has, however, been reported for some cells when grown in very rich Trypticase soy medium (72). It is possible that the variant cells were mutants which arose during selection under artificial growth conditions. In this study, growth in a complex medium (VNSS) led to an increase in volume of about 2.5-fold (Table (Table2).2). These data indicate that the cell volume of this bacterium may change depending on the growth medium and growth phase; however, the cells retain a volume of less than 0.1 μm3. Furthermore, in contrast to copiotrophic bacteria including V. angustum S14, they do not undergo reductive cell division during starvation.
To date, very few cells as small as S. alaskensis have been isolated from marine environments. Cycloclasticus oligotrophus RB1 (74), which was also isolated from Resurrection Bay (10), has a cell volume of 0.2 μm3 (74), and Prochlorococcus cells range from approximately 0.1 to 0.3 μm3 (calculated from reference 49). Vybiral et al. (73) attempted to isolate ultramicro-sized bacteria from the Bay of Calvi in the Mediterranean Sea by culturing the microorganisms that passed through a 0.2-μm-pore-size filter. Although most of the microorganisms in the filtrate initially had a diameter or width of less than 0.2 μm and a minor portion (less than 10%) had a diameter of 0.2 to 0.3 μm, the majority of cultured cells were rods with widths of 0.4 to 0.7 μm. This filtering approach was useful for obtaining the miniaturized (presumably starved) forms of cells but did not lead to the isolation of cells that maintained their ultramicro-size when growing. It is also possible that if ultramicrobacteria were present in the filtrates, they may not have grown in the medium that was used for culturing.
Extinction dilution.
The extinction dilution method, as used in this study, has proven to be successful for isolating abundant S. alaskensis ultramicrobacteria. A consistent finding has been the initial growth of cells only in liquid oligotrophic medium, with the ability to form colonies on solid media occurring after storage at 5°C for 6 to 12 months (60) or storage and monthly reculturing at 15°C (this study). The storage period enabled cells to form colonies not only on oligotrophic synthetic seawater medium (MPM [60]), but also on rich synthetic seawater medium (ZoBell 2216E [61] and VNSS [this study]). The initial lack of growth but the subsequent ability to grow on rich media indicates that the strains have facultative oligotrophic properties.
A number of other reports of the use of an extinction dilution method appear in the literature (see, e.g., references 2 and 21). Eilers et al. (21) reported that the dilution method and direct plating of samples from the North Sea was highly selective for gamma proteobacteria (not alpha proteobacteria). It is noteworthy, however, that in this study growth did not occur in tubes containing samples more dilute than 10−4 (J. Pernthaler, personal communication). The presence of this proportion of gamma-proteobacteria is consistent with the results of Schut et al. (60), who reported that 1% or less of total cells were comparatively large, rapidly growing cells typical of Vibrio species. In a similar way, Bernard et al. (2) sampled water from the Bay of Banyuls-sur-Mer in the Mediterranean Sea and found that the number of cells able to grow in dilution tubes was similar to the number of actively respiring cells (determined using 5-cyano-2,3-ditolyl tetrazolium chloride). However, these cells represented only 1 to 2% of the total counts (determined by microscopy with SYBR Green II).
It is notable that even in the present study, only 1 of a total of 50 samples diluted to at least 10−5 supported growth on solid media, and the culture developed this ability only after serial passaging for a total of 12 months. During storage and culturing, a prerequisite for survival is therefore that appropriate nutrients have been included and inhibitors excluded and that sufficient time has been allowed for the cells to grow.
The difficulties reported for obtaining cultures growing at 10−5 or 10−6 dilutions may also reflect the physiological status of the cells at the time of sampling. This may be manifested through diel periodicity, which has been reported to cause a shift from carbon limitation in the euphotic zone in the morning to nitrogen and phosphorous limitation in the afternoon, with an associated peak in dividing cells at midnight and the highest bacterial counts in the morning (37). Changing conditions of nutrient limitation have also been described for the same location of the Sargasso Sea, where it was reported to be carbon limiting during a period spanning June 1992 to January 1994 (12) and nitrogen or phosphorous limiting during July 1991 (13). In support of the importance of nutrient limitation, Eguchi (17) reported that vitamin B12-supplemented dilutions supported a 10-fold-larger number of cells for samples taken from Toyama Bay, Japan. It has also recently been found that stress survival of S. alaskensis RB2256 may be affected by nutrient-limited growth (47) and the type of agar medium used for recovery (A. Goodchild and R. Cavicchioli, unpublished results).
ACKNOWLEDGMENTS
M.E. thanks K. Fukami of Kochi University, M. Taniguchi of Deep Seawater Laboratory of Kochi, and I. Sugawara of Mie University for their help in collecting samples.
The research performed by R.C., M.O. and F.F. was supported by the Australian Research Council (grants A00103137 and A09700807), and the research performed by M.E. was supported by the Japanese Society for the Promotion of Science (grant 10660191, 1998).
REFERENCES
Articles from Applied and Environmental Microbiology are provided here courtesy of American Society for Microbiology (ASM)
Full text links
Read article at publisher's site: https://doi.org/10.1128/aem.67.11.4945-4954.2001
Read article for free, from open access legal sources, via Unpaywall:
https://aem.asm.org/content/aem/67/11/4945.full.pdf
Citations & impact
Impact metrics
Citations of article over time
Smart citations by scite.ai
Explore citation contexts and check if this article has been
supported or disputed.
https://scite.ai/reports/10.1128/aem.67.11.4945-4954.2001
Article citations
Use of modified ichip for the cultivation of thermo-tolerant microorganisms from the hot spring.
BMC Microbiol, 23(1):56, 03 Mar 2023
Cited by: 3 articles | PMID: 36869305 | PMCID: PMC9983152
Molecular hydrogen in seawater supports growth of diverse marine bacteria.
Nat Microbiol, 8(4):581-595, 06 Feb 2023
Cited by: 14 articles | PMID: 36747116 | PMCID: PMC10305171
From Recharge, to Groundwater, to Discharge Areas in Aquifer Systems in Quebec (Canada): Shaping of Microbial Diversity and Community Structure by Environmental Factors.
Genes (Basel), 14(1):1, 20 Dec 2022
Cited by: 2 articles | PMID: 36672742 | PMCID: PMC9858702
Comparison of bacterial community structure in PM2.5 during hazy and non-hazy periods in Guilin, South China.
Aerobiologia (Bologna), 39(1):87-103, 19 Dec 2022
Cited by: 1 article | PMID: 36568442 | PMCID: PMC9762634
Identification of two fnr genes and characterisation of their role in the anaerobic switch in Sphingopyxis granuli strain TFA.
Sci Rep, 10(1):21019, 03 Dec 2020
Cited by: 2 articles | PMID: 33273546 | PMCID: PMC7713065
Go to all (44) article citations
Data
Data behind the article
This data has been text mined from the article, or deposited into data resources.
BioStudies: supplemental material and supporting data
Nucleotide Sequences (Showing 17 of 17)
- (2 citations) ENA - AF378796
- (2 citations) ENA - Z73631
- (2 citations) ENA - AF378795
- (2 citations) ENA - AF148812
- (1 citation) ENA - AB022601
- (1 citation) ENA - AF181572
- (1 citation) ENA - D13727
- (1 citation) ENA - D13723
- (1 citation) ENA - U20756
- (1 citation) ENA - X94102
- (1 citation) ENA - U37337
- (1 citation) ENA - U90216
- (1 citation) ENA - D16149
- (1 citation) ENA - AB015049
- (1 citation) ENA - AF145754
- (1 citation) ENA - AF145753
- (1 citation) ENA - D17322
Show less
Similar Articles
To arrive at the top five similar articles we use a word-weighted algorithm to compare words from the Title and Abstract of each citation.
Sphingomonas alaskensis sp. nov., a dominant bacterium from a marine oligotrophic environment.
Int J Syst Evol Microbiol, 51(pt 1):73-79, 01 Jan 2001
Cited by: 23 articles | PMID: 11211276
Life under nutrient limitation in oligotrophic marine environments: an eco/physiological perspective of Sphingopyxis alaskensis (formerly Sphingomonas alaskensis).
Microb Ecol, 45(3):203-217, 14 Mar 2003
Cited by: 28 articles | PMID: 12632213
Review
Sphingomonas humi sp. nov., isolated from soil.
J Microbiol, 48(2):165-169, 01 Apr 2010
Cited by: 10 articles | PMID: 20437147
Sphingomonas molluscorum sp. nov., a novel marine isolate with antimicrobial activity.
Int J Syst Evol Microbiol, 57(pt 2):358-363, 01 Feb 2007
Cited by: 28 articles | PMID: 17267979