Abstract
Free full text

Immunogenicity of DNA Vaccines Expressing Tuberculosis Proteins Fused to Tissue Plasminogen Activator Signal Sequences
Abstract
Novel tuberculosis DNA vaccines encoding native ESAT-6, MPT-64, KatG, or HBHA mycobacterial proteins or the same proteins fused to tissue plasminogen activator (TPA) signal sequences were evaluated for their capacity to elicit humoral, cell-mediated, and protective immune responses in vaccinated mice. While all eight plasmids induced specific humoral responses, the constructs expressing the TPA fusions generally evoked higher antibody responses in vaccinated hosts. Although most of the DNA vaccines tested induced a substantial gamma interferon response in the spleen, the antigen-specific lung responses were 2- to 10-fold lower than the splenic responses at the time of challenge. DNA vaccines encoding the ESAT-6, MPT-64, and KatG antigens fused to TPA signal sequences evoked significant protective responses in mice aerogenically challenged with low doses of Mycobacterium tuberculosis Erdman 17 to 21 days after the final immunization. However, the protective response induced by live Mycobacterium bovis BCG vaccine was greater than the response induced by any of the DNA vaccines tested. These results suggest that the tuberculosis DNA vaccines were able to elicit substantial immune responses in suitably vaccinated mice, but further refinements to the constructs or the use of alternative immunization strategies will be needed to improve the efficacy of these vaccine candidates.
More than 100 years after its discovery, Mycobacterium tuberculosis remains a devastating microbial pathogen, responsible for substantial worldwide morbidity and mortality. The World Health Organization has estimated that there are more than eight million new cases of tuberculosis each year and that the annual death toll for tuberculosis exceeds three million (9). This international public health crisis has worsened in the past decade, largely because the global human immunodeficiency virus (HIV) epidemic is spreading rapidly into the regions with the highest rates of M. tuberculosis infection, and an increasing proportion of individuals infected with M. tuberculosis are becoming coinfected with HIV (40). In recent years, the increasing frequency of drug-resistant M. tuberculosis isolates has further complicated the clinical management of this disease (41). Outbreaks of multiple-drug-resistant tuberculosis in the United States, western Europe, and, more recently, in Latvia and Russia emphasize the importance of this public health problem (11, 32).
Clinical trials designed to evaluate the effectiveness of the current tuberculosis vaccine, Mycobacterium bovis BCG, in preventing primary disease have yielded extremely variable results with protective efficacies ranging from 0 to 80% and an estimated overall effectiveness of only 50% (5). Because of the variable effectiveness of BCG and the magnitude of the global public health and economic consequences of this disease, the development of new, improved, tuberculosis vaccines has become an international research priority. Several new types of tuberculosis vaccine preparations, including subunit, live attenuated, recombinant BCG, and DNA vaccines, are currently being investigated experimentally (20). Among these preparations, DNA vaccines appear to be particularly promising because they can induce persistent, cell-mediated immune responses to antigens isolated from a variety of viral, bacterial, and parasitic pathogens. In animal models of human disease, DNA vaccines have been shown to induce protective responses against HIV, influenza, bovine herpesvirus, rabies, leishmaniasis, malaria, herpes simplex virus, and tuberculosis (3, 10, 18). Besides their immunogenicity, DNA vaccines offer several other practical advantages. These include ease of production, the stability of episomal DNA, the capacity to stimulate cell-mediated responses without the need for adjuvants, and the eradication of time-consuming procedures needed for the purification of subunit proteins (10). In addition, safety concerns associated with vaccination of immunodeficient individuals with live organisms such as BCG would be largely eliminated if an effective tuberculosis DNA vaccine became available.
DNA vaccines encoding at least six tuberculosis proteins have recently been shown to induce protective responses to tuberculous challenge in animal models (19, 22, 25, 37, 45). In this study, we have extended these observations by evaluating the humoral, cell-mediated, and protective immune responses of four different pairs of tuberculosis DNA vaccines. We have shown that these DNA vaccines expressing mycobacterial proteins fused to tissue plasminogen activator (TPA) signal sequences elicit substantial protective activity and that the further development and refinement of this technology should be encouraged.
MATERIALS AND METHODS
Animals.
Specific-pathogen-free C57BL/6 female mice were obtained from the National Cancer Institute (Bethesda, Md.). The mice were 8 weeks old at the time of vaccination. The mice were maintained under barrier conditions and fed commercial mouse chow and water ad libitum.
DNA vaccine construction and purification.
The genes encoding the ESAT-6, MPT-64, HBHA, and KatG proteins were amplified from M. tuberculosis H37Rv chromosomal DNA with Vent DNA polymerase (New England BioLabs, Beverly, Mass.) by using primers designed from the M. tuberculosis genome sequence database. All genes encoding an antigen fused to a TPA signal sequence were amplified with a 5′ primer containing an NheI restriction site and a 3′ primer designed with a BamHI site. The genes that did not encode a TPA signal peptide were amplified with a HindIII site containing a 5′ primer and a 3′ primer designed with a BamHI site. Each PCR product was cloned initially into the Zero Blunt cloning vector (Invitrogen, San Diego, Calif.) and then into the eukaryotic expression vector pJW4303 (kindly provided by James Arthos, National Institute of Allergy and Infectious Diseases, National Institutes of Health) (43). The recombinant pJW4303 constructs were transformed into One Shot TOP10 Escherichia coli cells (Invitrogen) and plated on Luria-Bertani agar containing ampicillin (100 μg/ml). Lastly, large amounts of endotoxin-free plasmid DNA were prepared and purified with the Qiagen EndoFree Plasmid Maxi kit (Qiagen, Chatsworth, Calif.). The endotoxin levels in the final vaccine preparations were assayed with the QCL-1000 Limulus Amebocyte Lysate kit (BioWhittaker, Walkersville, Md.). The endotoxin concentrations of these final preparations were usually less than 50 endotoxin units per mg of plasmid DNA.
Mycobacterial protein purification.
The ESAT-6, KatG, MPT-64, and HBHA antigens were purified as polyhistidine-tagged recombinant proteins by using the Xpress System (Invitrogen). The ESAT-6 and KatG proteins were expressed from the pET-24B plasmid, while MPT-64 and HBHA were expressed from the pET-15B vector. The KatG, MPT-64, and HBHA polyhistidine-tagged proteins were purified under native conditions using Ni-affinity chromatography following the manufacturer’s protocol. The ESAT-6 recombinant protein was purified with Ni-affinity chromatography under denaturing conditions as described by the manufacturer. In initial studies, native purified MPT-64 provided by M. Singh from the World Health Organization recombinant protein bank was also utilized.
The evaluation of antigen-specific antibody levels in vaccinated mice by enzyme-linked immunosorbent assay.
Immulon 1 plates (Dynatech, Chantilly, Va.) were coated at 4°C overnight with 0.1 ml of purified protein (5 μg/ml) in coating solution (KPL, Gaithersburg, Md.) and then blocked the next day with 1% bovine serum albumin in phosphate-buffered saline (PBS) for 30 min at 37°C. Serum samples were applied in serial twofold dilutions from 1:25 and were incubated for 2 h at 37°C. Each serum sample represented pooled sera from three vaccinated or control mice. After the plates were washed with PBS-Tween 20 (0.05%), anti-mouse immunoglobulin G (IgG) alkaline phosphatase conjugate (Sigma, St. Louis, Mo.) was added for detection of specific antibodies. For IgG isotype detection, goat anti-mouse IgG1 and IgG2a alkaline phosphatase conjugates (Southern Biotechnology, Birmingham, Ala.) were used. For color development, the p-NPP phosphatase system (KPL) was added; the reaction was stopped at 20 min by the addition of EDTA to a final concentration of 62 mM. The optical density was measured at 405 nm. The end point titer was defined as the highest dilution of serum that gave an absorbance value that exceeded an optical density of 0.050 and was twofold greater than that of the matched dilution of normal mouse sera (29).
Cytokine ELISPOT assay.
Eight C57BL/6 mice per group were vaccinated intramuscularly in the thigh three times at 3-week intervals with 100 μg of plasmid DNA per injection. Three weeks after the final inoculation, three mice were removed for cytokine analyses and five mice were challenged as described below. Cytokine induction was evaluated by using an ELISPOT protocol as previously described (23, 28). Briefly, 96-well Immulon-2 microtiter plates (Dynatech) were coated with 10 μg of either anti-gamma interferon (IFN-γ) (clone R4-6A2; Pharmingen, San Diego, Calif.) or anti-interleukin-4 (IL-4) (clone BVD4-1D11; Endogen, Woburn, Mass.) mouse antibody per ml of PBS buffer (pH 7.3) for 5 h at room temperature. The plates were blocked with 5% bovine serum albumin dissolved in a solution containing 0.025% Tween 20 in PBS for 2 h and were washed with a fresh application of the same solution. Spleen suspensions were prepared in RPMI 1640 supplemented with 5% heat-inactivated fetal calf serum, 5% nonessential amino acid, 10 mM sodium pyruvate, 2-mercaptoethanol, and 100 U of penicillin-streptomycin per ml (complete medium). The lungs were placed into Hanks balanced salt solution (HBSS), aspirated to remove blood, and digested for 1 h with a prescreened lot of 2.5% collagenase (Worthington Biochemical, Cleveland, Ohio). The digested tissue was next passed through a 40-gauge stainless steel sieve, and the resulting cell suspension was washed three times with complete medium. Serial dilutions of the single-cell suspensions, starting with 106 cells/well, were incubated on anticytokine-coated plates in complete medium for 12 to 16 h at 37°C in a humidified 5% CO2 incubator. When appropriate, purified mycobacterial antigens were added to the plates at a concentration of 10 μg/ml during this incubation period. The plates were washed with 0.025% Tween 20 and overlaid with 1 μg of biotinylated anti-IFN-γ (clone XMG1; Pharmingen) or anti-IL-4 (clone BVD6-24G2; Pharmingen) antibodies per ml at room temperature. After 2 h, a 1:5,000 dilution of avidin-conjugated alkaline phosphatase was added for 1 h at room temperature. Individual cytokine-secreting cells were visualized by the addition of the substrate 5-bromo-4-chloro-3 indolylphosphate (BCIP)/nitroblue tetrazolium agarose mixture (Sigma).
In vitro expression of mycobacterial antigens from DNA vaccine constructs.
Rhabdomyosarcoma cells (ATCC CCL 136) were grown in high-glucose Dulbecco’s modified Eagle medium supplemented with 10% heat-inactivated fetal calf serum, 2 mM glutamine, 20 mM HEPES, 100 U of ampicillin per ml, and 100 μg of streptomycin per ml. At 60% confluency in 35-mm-diameter culture plates, cells were transfected with a mixture containing 2 μg of plasmid DNA and 6 μl of Lipofectin (Gibco) in 200 μl of serum-free medium (OPTI-MEM; Gibco) for 5 h. The Lipofectin-containing medium was removed, and 1 ml of complete medium was added. The cells were incubated for an additional 24 h, washed twice in PBS, harvested, and lysed at 4°C in lysis buffer (1% Nonidet P-40, 0.1% sodium dodecyl sulfate, 50 mM Tris-HCl [pH 8.0], 150 mM NaCl, protease inhibitor cocktail). To evaluate extracellular protein concentrations, the cells were washed with HBSS and incubated for an additional 8 h in HBSS, and the supernatants were collected and precipitated with 10% trichloroacetic acid. After a washing with 9 volumes of acetone, the trichloroacetic acid precipitates were suspended in sodium dodecyl sulfate-polyacrylamide gel electrophoresis loading buffer. Protein expression was visualized with the ECL chemiluminescence system (Amersham, Arlington, Ill.).
Evaluation of the protective activity of DNA vaccines against low-dose aerosol challenge.
The C57BL/6 mice (five mice per group) were vaccinated intramuscularly into the thigh three times at 3-week intervals, with 100 μg of plasmid DNA per injection. Control mice were vaccinated with 106 CFU of BCG Pasteur injected subcutaneously. The mice were challenged by the aerosol route about 17 to 21 days after receiving the final DNA vaccine inoculation and 6 weeks after receiving the BCG vaccination. For the challenge, a frozen ampoule of M. tuberculosis Erdman was thawed and subjected to 10 s of vibration on a vortex mixer. The suspension was then diluted in 0.04% Tween 80-saline to a concentration which would introduce 200 to 500 CFUs into the lung over a 30-min exposure period in a Middlebrook chamber (Glas Col, Terre Haute, Ind.). The concentration of M. tuberculosis Erdman needed to produce this inoculum with the nebulizer had been established in earlier dose response studies (6). Five mice were sacrificed during the first 24 h to confirm the size of the challenge dose. After 28 days, the remaining challenged mice were sacrificed, and the lungs and spleens from individual mice were removed aseptically and homogenized separately in 5 ml of cold Tween 80-saline by using a Seward stomacher 80 blender (Tekmar, Cincinnati, Ohio). The homogenates were diluted serially in Tween 80-saline, and 50-μl aliquots were plated on Middlebrook 7H11 agar. Samples from the BCG-vaccinated controls were plated on 7H11 agar containing 2 μg of thiophenecarboxylic acid hydrazide per ml (Sigma) to inhibit the growth of any residual BCG (6). For determination of the number of CFUs in organs, the plates were incubated at 37°C for 14 to 21 days in sealed plastic bags before the colonies were counted. The counting error for five replicate counts was usually less than 20% of the mean.
RESULTS
Selection of antigens and vaccination schedules.
Four immunogenic M. tuberculosis antigens—ESAT-6, MPT-64, KatG, and HBHA—were selected for testing by using genetic immunization technology (2, 24, 26, 39). The genes encoding these antigens were amplified by PCR and cloned into the pJW4303 eukaryotic expression vector. This vector was designed to express antigens with or without a TPA signal sequence. Since the presence of a TPA signal sequence should facilitate the secretion of the antigens from the host cells, the evaluation of secreted and nonsecreted forms of these antigens permitted an assessment of the effect of differential intracellular targeting on the quantity and quality of immune responses.
Since the immunization schedule for tuberculosis DNA vaccines has not yet been systematically optimized, two immunization schedules were initially evaluated. Injections of a standard dose of 100 μg of plasmid DNA were administered intramuscularly at 3- or 6-week intervals. These experiments indicated that three injections of DNA vaccines given at 3-week intervals generated better humoral and protective immune responses than the corresponding 6-week protocol (data not shown). These studies also demonstrated that maximal responses (relative to 1 or 2 injections) were produced after three injections. Therefore, a schedule of three 100-μg injections of plasmid DNA given 3 weeks apart was selected as being optimal for subsequent immunization experiments.
Relative concentrations of mycobacterial proteins expressed in eukaryotic cells.
Montgomery et al. have recently reported that the amount of expression from a DNA vaccine encoding a TPA-positive antigen 85A protein that had been transfected into a rhabdomyosarcoma cell line was much greater than the expression from a native TPA-negative antigen 85A DNA construct (27). To determine whether the concentrations of antigens expressed from the TPA-positive plasmids examined in this study exceeded the levels of antigen expressed from the corresponding TPA-negative constructs, rhabdomyosarcoma cells were first transfected with DNA vaccines expressing the ESAT-6, KatG, HBHA, or MPT-64 proteins. After 48 h, cell lysates were prepared, and specific protein expression was monitored by immunoblot analyses. As shown in Fig. Fig.1A,1A, the concentrations of all TPA-positive antigens tested in this in vitro system exceeded the levels of native proteins. The concentrations of the native KatG, MPT-64, and HBHA antigens were clearly reduced relative to the corresponding TPA-positive proteins (Fig. (Fig.1A).1A). Moreover, while substantial expression of the TPA-positive ESAT-6 protein was observed, the concentration of the native ESAT-6 antigen was significantly decreased and virtually undetectable in some experiments (Fig. (Fig.1A,1A, lane 5). To evaluate the levels of extracellular proteins, the culture supernatants of cell lines transfected with either the MPT-64 or KatG plasmids were evaluated (Fig. (Fig.1B).1B). When these cell culture fluids were analyzed, much higher concentrations of the TPA-positive proteins (compared to the corresponding native antigen) were also detected (Fig. (Fig.1B).1B).
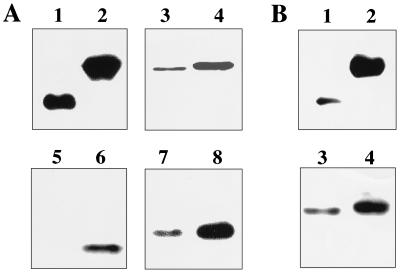
Immunoblot analyses of cell lysates (A) and cell supernatants (B) from rhabdomyosarcoma cells transfected with DNA vaccines expressing either TPA fusion or native proteins. The cell lysates are shown in the following order: MPT-64 negative (lane 1), MPT-64 positive (lane 2), KatG negative (lane 3), KatG positive (lane 4), ESAT-6 negative (lane 5), ESAT-6 positive (lane 6), HBHA negative (lane 7), and HBHA positive (lane 8). In panel B, the rhabdomyosarcoma cell supernatant precipitates from transfections with MPT-64-negative (lane 1), MPT-64-positive (lane 2), KatG-negative (lane 3), and KatG-positive (lane 4) constructs are shown.
Humoral responses to DNA vaccination.
The antibody titers against the four mycobacterial antigens examined in this study were determined in sera harvested from immunized mice about 2 weeks after the third injection. While no antigen-specific antibodies were detected in mice immunized with vector control DNA, all the DNA constructs tested generated a detectable antibody response. As seen in Table Table1,1, vaccination of the mice with most of the TPA-positive constructs consistently induced higher total IgG antibody levels than those induced by the corresponding TPA-negative constructs. Substantial differences in antibody titers were noted between the pairs of ESAT-6, MPT-64, and HBHA constructs. In general, the antibody concentrations induced in response to DNA vaccination reflect the levels of antigenic expression seen in vitro.
TABLE 1
Humoral responses to tuberculosis DNAvaccines
Vaccine | End point titer
| ||
---|---|---|---|
IgG | IgG1 | IgG2a | |
ESAT-6 positive | 12,600 | 1,600 | 3,200 |
ESAT-6 negative | 800 | 50 | 200 |
KatG positive | 25,600 | 12,800 | 6,400 |
KatG negative | 25,600 | 3,200 | 12,800 |
MPT-64 positive | 51,200 | 25,600 | 12,800 |
MPT-64 negative | 400 | 100 | 400 |
HBHA positive | 25,600 | 6,400 | 12,800 |
HBHA negative | 3,200 | 800 | 800 |
Since the relative IgG2a and IgG1 antibody levels are a marker of the overall T-cell phenotype, the IgG2a and IgG1 titers were also determined for sera collected from these vaccinated animals (Table (Table1).1). A Th2-type response favors the production of IgG1 antibodies, while IgG2a antibodies are associated with a Th1 response (35). For the TPA-positive DNA vaccines, the postimmunization IgG1 and IgG2a titers were generally similar. Although antigen-specific differences in the IgG2a/IgG1 ratio were noted, no consistent pattern was apparent. These results suggest that the TPA-positive DNA vaccines induced a mixed T-cell phenotype. In contrast, the IgG antibody titer and isotype analyses indicate that the MPT-64 TPA-negative vaccine generates a Th1-biased response. This construct induced relatively low levels of IgG antibody with a predominant IgG2a isotype (Table (Table11).
Cytokine responses in vaccinated animals.
Protective immunity against mycobacterial disease is believed to largely result from the induction of a Th1 cellular immune response, with secretion of Th1-type cytokines playing an important role in preventing active disease (31, 44). In order to assess the effect of DNA vaccination on the level of cell-mediated immunity, cytokine responses to the four purified antigens were assessed in immunized and naive animals with ELISPOT assays. Since IFN-γ is a critical cytokine associated with the induction of an antimycobacterial protective response (8, 12, 30), we initially focused on this cytokine response in the vaccinated animals. As seen in Fig. Fig.2,2, the ELISPOT data indicate that robust antigen-specific IFN-γ responses were detected in the splenocytes of the vaccinated mice. Immunizations with the ESAT-6, KatG, and MPT-64 TPA-positive and -negative constructs all induced spleen cells which responded to specific antigens by producing IFN-γ. However, more modest numbers of antigen-induced IFN-γ SFUs were detected in the lungs of the vaccinated mice. For each construct, the number of lung IFN-γ SFUs was reduced relative to the splenic IFN-γ SFU values. In fact, no IFN-γ SFUs were detected when specific antigen was used to stimulate the lung cells from mice vaccinated with the HBHA constructs. Among the DNA vaccines, immunization with the two KatG constructs consistently induced the best IFN-γ responses in the lung.
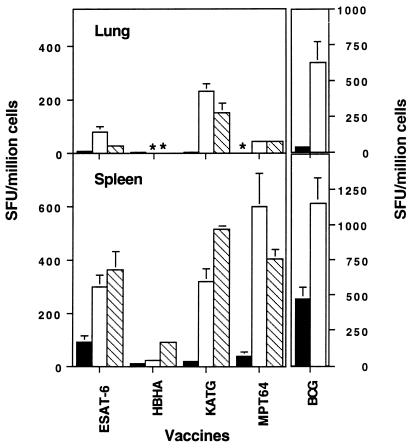
IFN-γ antigen-specific responses in the lungs and spleens of vaccinated mice. After immunization with DNA vaccines or BCG, the lungs and spleens were removed and the numbers of antigen-specific SFUs were determined by ELISPOT analysis as described in the Materials and Methods section. Data are reported for naive mice (closed boxes), TPA-negative vaccines (open boxes), and TPA-positive vaccines (hatched boxes). Nondetectable responses are represented by stars. The BCG response is reported in the adjacent graph. Error bars represent standard errors.
To facilitate assessment of the T-cell phenotype induced by immunization, the numbers of splenic and lung IL-4 SFUs were also determined by ELISPOT analysis (Fig. (Fig.3).3). Vaccination with both TPA-positive and -negative DNA vaccines induced splenic and lung antigen-specific IL-4 responses. Consistent with the IFN-γ results, the antigen-specific IL-4 responses were higher in the splenocytes than in the lung cells of animals vaccinated with TPA-negative DNA constructs. The Th1 bias suggested by the antibody profile for the native MPT-64 construct is also reflected in the relative numbers of IL-4 and IFN-γ SFUs detected in the spleen. For this vaccine, the number of IFN-γ SFUs (518 ± 125) induced by vaccination exceeded the level of vaccine-induced IL-4 SFUs by threefold (170 ± 49).

IL-4 antigen-specific responses in the lungs and spleens of vaccinated mice. After immunization with DNA vaccines or BCG, the lungs and spleens were removed and the numbers of antigen-specific SFUs were determined by ELISPOT analysis. Data are reported for naive mice (closed boxes), TPA-negative vaccines (open boxes), and TPA-positive vaccines (hatched boxes). Error bars represent standard errors.
Control mice were immunized with a single dose of the live BCG vaccine, and the cytokine response in the lungs and spleen was assessed 6 weeks later. As seen in Fig. Fig.22 and and3,3, BCG evoked a predominantly Th1 response, with considerable IFN-γ SFUs detected compared to a relatively limited number of IL-4 SFUs (above the naive background). Immunization with live BCG induced six times more IFN-γ SFUs than IL-4 SFUs in both splenocytes and lung cells.
Genetic immunization induces protective immune responses.
The capacity of these DNA vaccines to elicit protective responses was evaluated 28 days after a low-dose aerosol challenge (31). Each vaccine was tested in two or three independent experiments. Protection data from a representative experiment are shown in Table Table2.2. Since the bacterial DNA can activate immunity nonspecifically, an important control was an assessment of the protective response seen in mice vaccinated with only the vector DNA. In the experiment whose results are shown in Table Table22 and in three subsequent studies, 100 μg of vector alone did not significantly reduce the number of CFUs seen 28 days after challenge.
TABLE 2
Protective activity of tuberculosis DNAvaccinesa
Vaccine | No. of lung CFUs | Lung protectionb | No. of spleen CFUs | Spleen protection |
---|---|---|---|---|
Naive | 7.05![]() ![]() | 5.60![]() ![]() | ||
Vector | 6.97![]() ![]() | 5.43![]() ![]() | ||
ESAT-6 positive | 5.97![]() ![]() | 1.08 | 4.99![]() ![]() | 0.61 |
ESAT-6 negative | 6.44![]() ![]() | 0.61 | 5.04![]() ![]() | 0.56 |
MPT-64 positive | 6.23![]() ![]() | 0.82 | 5.19![]() ![]() | 0.41 |
MPT-64 negative | 6.68![]() ![]() | 0.37 | 5.29![]() ![]() | |
KatG positive | 6.40![]() ![]() | 0.65 | 4.90![]() ![]() | 0.70 |
KatG negative | 6.79![]() ![]() | 5.59![]() ![]() | ||
HBHA positive | 6.80![]() ![]() | 5.33![]() ![]() | ||
HBHA negative | 7.11![]() ![]() | 5.52![]() ![]() | ||
BCG | 5.56![]() ![]() | 1.49 | 3.31![]() ![]() | 2.29 |
Vaccination with three of the TPA-positive constructs—ESAT-6, KatG, and MPT-64—consistently reduced the number of day 28 lung CFUs relative to that seen in the naive controls (p < 0.05). Immunization with the ESAT-6 TPA-positive plasmid resulted in a 90% reduction of bacteria in the lung compared to controls, whereas vaccination with the KatG- or MPT-64 TPA-positive constructs resulted in a 75 to 85% drop in number of lung bacteria. Moreover, mice immunized with the ESAT-6 or KatG TPA-positive constructs showed significantly decreased dissemination of the lung infection to the spleen. Interestingly, the TPA-negative vaccines consistently elicited less protective activity than their TPA-positive counterparts. Although vaccination with the ESAT-6 or MPT-64 TPA-negative plasmids significantly reduced the number of CFUs in the lung after 28 days, the level of protective activity induced was consistently less than that conferred by the corresponding TPA-positive constructs. This difference was especially noticeable for the splenic CFU counts. Of the four TPA-negative constructs, only the ESAT-6 TPA-negative plasmid reduced the dissemination of the infection to the spleen relative to that seen in the controls. Finally, neither HBHA construct significantly reduced the lung or spleen counts. This result supports our vector control data and further suggests that the protective activity of the DNA vaccine preparations tested was not due to nonspecific immune activation by the bacterial DNA.
Control mice were immunized with live BCG and challenged with M. tuberculosis Erdman. Dramatic reductions compared to the naive controls were observed for both lung (1.49 log10) and spleen (2.29 log10) CFUs. Clearly, the reductions in lung and spleen CFUs in the BCG-vaccinated mice exceeded those seen in the lung and spleen of DNA-vaccinated animals. While several of these DNA vaccine preparations were able to induce a significant immune response, the level of protection elicited by these DNA plasmids was lower than the protective immunity induced by an optimal dose of BCG vaccine in this mouse model of human tuberculosis.
DISCUSSION
Genetic vaccination with plasmids encoding mycobacterial genes is clearly an effective means of generating cell-mediated, humoral, and protective immune responses against M. tuberculosis antigens. Using intramuscular immunization protocols, we have identified three proteins expressed from DNA vaccines—ESAT-6, KatG, and MPT-64—which elicit substantial protective activity when evaluated 28 days after a low-dose aerosol challenge. Because these studies were designed primarily as a screen to identify tuberculosis DNA vaccines with protective activity, it should be emphasized that our aerogenic challenges were carried out 3 weeks postimmunization, when vaccination should have evoked optimal antituberculous immunity. Obviously much more preclinical testing of the most active plasmids, including an assessment of their long-term effects on mouse immune memory and an evaluation of their effectiveness in other animal models, will be needed prior to their consideration as candidate vaccines for human vaccine trials.
Our comparison of four different pairs of plasmid constructs demonstrated that DNA vaccines expressing mycobacterial antigens fused to TPA signal sequences are generally more protective against tuberculous challenge in this model system than are DNA vaccines expressing the same native protein without a signal sequence. Moreover, our in vitro data suggests that the difference in protective effectiveness may be the result of elevated concentrations of TPA fusion proteins relative to native antigens in transfected cells. The higher levels of TPA fusion proteins in host cells should lead to increased secretion of these proteins with elevated uptake by antigen-presenting cells, and thus, a more generalized activation of the immune system. The higher antibody titers detected in animals vaccinated with TPA-positive constructs are a reflection of this enhanced immune stimulation. Whether the increased protein concentrations of TPA-fused antigens compared to native proteins are the result of increased expression, enhanced stability, or both is currently being investigated. The correlation between antigenic expression levels and the extent of immune activation has been reported for other experimental DNA vaccines. Haddad et al. demonstrated that DNA constructs expressing chimeric malaria antigens which generated comparable concentrations of antigen in vitro elicited similar humoral responses in vivo, despite different intracellular targeting of the expressed antigens (14). Moreover, Hariharan et al. showed that protein expression and immune induction were enhanced in mice inoculated with Sindbis virus-based vectors relative to those in mice that had been immunized with conventional vaccines (16).
Despite these apparent differences in the protein concentrations generated from the TPA-positive and -negative constructs, both types of constructs induced similar levels of IFN-γ, a critical cytokine for antimycobacterial protection (8, 12, 30). There are at least two possible explanations for this observation. First, the relationship between the dose of antigen expressed from DNA vaccines and cytokine production is often extremely complex. For many purified antigens, the extent of cytokine production does not directly correlate with the antigenic dose (17). In the context of DNA vaccination, where antigen expression can persist for months, this complex relationship between antigen dose and cytokine production becomes even more difficult to assess. Alternatively, TPA-negative vaccines may induce substantial cytokine synthesis despite low concentrations of recombinant protein in the transfected cells, because fragments of antigens expressed from these plasmid constructs rather than intact proteins could act as immunomodulators. This hypothesis has been supported by recent studies showing that ubiquinated proteins expressed from DNA vaccines can induce immune responses despite being essentially completely degraded by the host cell and also by the observation that heat shock proteins can transfer peptides to antigen-presenting cells for the induction of cytotoxic T lymphocyte (CTL) responses (33, 36, 42).
It is also of interest that the protective responses do not directly correlate with the levels of in vitro IFN-γ synthesis. Certainly, the failure of two nonprotective vaccines (HBHA positive and HBHA negative) to evoke significant IFN-γ responses in the spleen and the lung seems consistent with reports suggesting an important role for IFN-γ in antimycobacterial protection. However, the correlation between in vitro IFN-γ synthesis and level of protection fails when data from the ESAT-6 constructs are compared with the results for other vaccines. The ESAT-6 TPA-positive vaccine generated a larger protective response while inducing less IFN-γ in vitro than the KatG TPA-positive plasmid, and the native ESAT-6 construct was more protective than the native MPT-64 vaccine despite a smaller splenic IFN-γ response. One explanation for these discordant results may relate to the level of expression of M. tuberculosis antigens in vivo after challenge. For instance, the considerable protective activity conferred by the ESAT-6-positive plasmid could be the result of elevated expression of ESAT-6, relative to the other antigens, by M. tuberculosis following aerosol challenge. This scenario, however, cannot explain why IFN-γ production and protection do not correlate for the corresponding TPA-positive and -negative constructs. For example, although the ESAT-6 TPA-positive vaccine is more protective than the corresponding ESAT-6 construct expressing native antigen, the TPA-negative plasmid induced similar levels of IFN-γ in the spleen and more IFN-γ in the lung. Since the degree of protection does not directly correlate with the vaccine-induced IFN-γ concentrations in this circumstance, other immune responses must be differentially elicited by TPA-positive plasmids to generate greater protective immunity. Consequently, the enhanced protection seen for these TPA-positive plasmids may result from their capacity to induce higher concentrations of other critical cytokines or chemokines (13). Alternatively, the TPA-positive vectors may more effectively induce mycobacterial CTL responses. It has been speculated that the presence of signal sequences on DNA vaccine constructs enhances CTL responses by targeting the expressed antigen directly to the endoplasmic reticulum and thus obviating the need for antigen to be processed and translocated to that structure (4). For intracellular pathogens such as Listeria monocytogenes, CTLs are clearly required for protective immunity (21). While the role of mycobacterial CTLs in protection is less certain, recent evidence suggests that CTLs may also be important for establishing long-term protection against tuberculosis (34).
Although we have demonstrated that these DNA vaccines induce protective activity, none of the vaccines tested were as protective as live BCG in this animal model. Vaccination with ESAT-6, our most active DNA vaccine, decreased bacterial CFUs in the lung by approximately 90% following challenge, whereas the corresponding reduction was greater than 95% in the BCG-immunized controls. This difference was even more apparent in our assessment of the spleen burden at day 28. BCG was highly effective in reducing dissemination of the lung infection to the spleen (2.3 log10 reduction) compared to the ESAT-6 TPA-positive construct (0.6 log10 reduction). The low relative effectiveness of current DNA vaccines compared to BCG has been previously reported for both the mouse and guinea pig models of tuberculosis (1, 22). Our evaluation of the relative immune responses elicited by vaccination with the DNA vaccines and with BCG suggests that the superior protection afforded by BCG in this model may be due to its capacity to induce a substantial cell-mediated response with a predominant Th1 phenotype in both the lungs and spleens of vaccinated mice. The ELISPOT data clearly shows that BCG elicits a Th1-biased immune response, because an elevated IFN-γ response and a relatively low IL-4 response were detected in both the lungs and spleens of BCG-vaccinated mice. In contrast, immunization with the most protective constructs, the TPA-positive DNA vaccines, generated a mixed Th1-Th2 phenotype. The presence of similar levels of IgG2a (Th1) and IgG1 (Th2) antigen-specific antibodies in sera from DNA-vaccinated mice and elevated numbers of both IFN-γ and IL-4 SFUs in splenocytes of mice immunized with the DNA constructs support a mixed T-cell profile. Moreover, most of these DNA vaccine constructs induced only modest IFN-γ lung responses relative to that achieved in the spleen. This result was not unexpected since Cooper et al. have also shown that immunity to mycobacterial infections is often expressed at different levels in the lungs compared to other organs (7). Surprisingly, the MPT-64 TPA-negative construct, which produced our most predominant Th1 response, was only moderately protective. Although this vaccine produced a Th1-biased response, the number of IFN-γ SFUs generated was much lower than that for BCG, especially in the lung. This result suggests that both the phenotype and the intensity of the immune response induced by a DNA vaccine impact the extent of protection observed.
While our data indicate that these single DNA vaccines need further improvement before clinical evaluation can be initiated, the results suggest at least two possibilities for augmenting the effectiveness of tuberculosis DNA vaccines. Coadministration of plasmids expressing IL-12 could shift the immune response induced by these vaccines from a mixed T-helper-cell population toward the desired Th1 phenotype, with a potential increase in protective effectiveness. Additionally, intranasal administration of tuberculosis DNA constructs formulated in novel delivery systems to enhance expression within the lung could elevate antigen-specific immune responses against the virulent tubercle bacilli (38). In this regard, Hamajima et al. have recently demonstrated that intranasal inoculation of an HIV DNA vaccine increased the HIV-specific mucosal and systemic antibody and cell-mediated immune responses (15). These real possibilities for improving and refining DNA vaccination against this important human pathogen strongly suggest that this approach could eventually contribute to the global control of pulmonary tuberculosis.
REFERENCES
Articles from Infection and Immunity are provided here courtesy of American Society for Microbiology (ASM)
Full text links
Read article at publisher's site: https://doi.org/10.1128/iai.67.9.4780-4786.1999
Read article for free, from open access legal sources, via Unpaywall:
https://iai.asm.org/content/iai/67/9/4780.full.pdf
Free after 4 months at iai.asm.org
http://iai.asm.org/cgi/content/full/67/9/4780
Free after 4 months at iai.asm.org
http://iai.asm.org/cgi/reprint/67/9/4780
Free to read at iai.asm.org
http://iai.asm.org/cgi/content/abstract/67/9/4780
Citations & impact
Impact metrics
Article citations
Humoral and Cellular Immune Responses Induced by Bivalent DNA Vaccines Expressing Fusion Capsid Proteins of Porcine Circovirus Genotypes 2a and 2b.
Vaccines (Basel), 12(3):324, 18 Mar 2024
Cited by: 0 articles | PMID: 38543958 | PMCID: PMC10974225
A Genetically Engineered Bivalent Vaccine Coexpressing a Molecular Adjuvant against Classical Swine Fever and Porcine Epidemic Diarrhea.
Int J Mol Sci, 24(15):11954, 26 Jul 2023
Cited by: 0 articles | PMID: 37569329 | PMCID: PMC10419043
Preclinical Development and Assessment of Viral Vectors Expressing a Fusion Antigen of Plasmodium falciparum LSA1 and LSAP2 for Efficacy against Liver-Stage Malaria.
Infect Immun, 88(2):e00573-19, 22 Jan 2020
Cited by: 6 articles | PMID: 31740525 | PMCID: PMC6977128
Dengue Mosaic Vaccines Enhance Cellular Immunity and Expand the Breadth of Neutralizing Antibody Against All Four Serotypes of Dengue Viruses in Mice.
Front Immunol, 10:1429, 20 Jun 2019
Cited by: 5 articles | PMID: 31281322 | PMCID: PMC6596366
Middle East Respiratory Syndrome Vaccine Candidates: Cautious Optimism.
Viruses, 11(1):E74, 17 Jan 2019
Cited by: 47 articles | PMID: 30658390 | PMCID: PMC6356267
Review Free full text in Europe PMC
Go to all (101) article citations
Similar Articles
To arrive at the top five similar articles we use a word-weighted algorithm to compare words from the Title and Abstract of each citation.
DNA vaccine combinations expressing either tissue plasminogen activator signal sequence fusion proteins or ubiquitin-conjugated antigens induce sustained protective immunity in a mouse model of pulmonary tuberculosis.
Infect Immun, 70(1):292-302, 01 Jan 2002
Cited by: 81 articles | PMID: 11748195 | PMCID: PMC127618
The immunogenicity of single and combination DNA vaccines against tuberculosis.
Vaccine, 18(20):2155-2163, 01 Apr 2000
Cited by: 83 articles | PMID: 10715531
Tissue plasminogen activator (tPA) signal sequence enhances immunogenicity of MVA-based vaccine against tuberculosis.
Immunol Lett, 190:51-57, 17 Jul 2017
Cited by: 33 articles | PMID: 28728855
Differential protective efficacy of DNA vaccines expressing secreted proteins of Mycobacterium tuberculosis.
Infect Immun, 67(4):1702-1707, 01 Apr 1999
Cited by: 193 articles | PMID: 10085007 | PMCID: PMC96517