Abstract
Free full text

Eosinophils generate brominating oxidants in allergen-induced asthma
Abstract
Eosinophils promote tissue injury and contribute to the pathogenesis of allergen-triggered diseases like asthma, but the chemical basis of damage to eosinophil targets is unknown. We now demonstrate that eosinophil activation in vivo results in oxidative damage of proteins through bromination of tyrosine residues, a heretofore unrecognized pathway for covalent modification of biologic targets in human tissues. Mass spectrometric studies demonstrated that 3-bromotyrosine serves as a specific “molecular fingerprint” for proteins modified through the eosinophil peroxidase-H2O2 system in the presence of plasma levels of halides. We applied a localized allergen challenge to model the effects of eosinophils and brominating oxidants in human lung injury. Endobronchial biopsy specimens from allergen-challenged lung segments of asthmatic, but not healthy control, subjects demonstrated significant enrichments in eosinophils and eosinophil peroxidase. Baseline levels of 3-bromotyrosine in bronchoalveolar lavage (BAL) proteins from mildly allergic asthmatic individuals were modestly but not statistically significantly elevated over those in control subjects. After exposure to segmental allergen challenge, lung segments of asthmatics, but not healthy control subjects, exhibited a >10-fold increase in BAL 3-bromotyrosine content, but only two- to threefold increases in 3-chlorotyrosine, a specific oxidation product formed by neutrophil- and monocyte-derived myeloperoxidase. These results identify reactive brominating species produced by eosinophils as a distinct class of oxidants formed in vivo. They also reveal eosinophil peroxidase as a potential therapeutic target for allergen-triggered inflammatory tissue injury in humans.
Introduction
Eosinophils are a specialized form of white blood cells that play a unique role in host defense mechanisms. In contrast to neutrophils, which primarily ingest and kill relatively small microbes within the harsh environs of a phagolysosomal compartment, eosinophils are recruited to destroy helminthic parasites and other large invading metazoan pathogens. The biochemical mechanisms used by eosinophils in vivo to perform their functions are not known. In vitro studies have focused on their capacity to generate inflammatory mediators, release cytotoxic granule constituents, and generate free radicals and reactive oxidant species (1–6). The reactive species they form, however, also have the potential to harm host tissue and cause tissue injury. Allergic inflammatory diseases such as chronic asthma are characterized by eosinophil recruitment and activation (5–7). Although eosinophils are widely believed to play a central role in promoting inflammatory injury in asthma, the biochemical pathways used by these phagocytes to damage biologic targets in vivo have not yet been established.
Activation of eosinophils in vitro by a variety of stimuli often triggers a respiratory burst in which superoxide (O2•–) and hydrogen peroxide (H2O2) are formed and granule contents are secreted into the extracellular milieu (1). The respiratory burst of eosinophils generates several times as much O2•– and H2O2 as a corresponding number of neutrophils (8–10). Eosinophil peroxidase (EPO), a highly cationic heme protein secreted by activated eosinophils, is believed to play an instrumental role in mediating both the destruction of invading parasites and pathogens and the pathological damage of host tissues (1–6, 11–16). EPO is one of the most abundant proteins in eosinophils (15 μg/106 eosinophils), comprising approximately 25% of the total protein mass of specific granule protein (17). A structurally and functionally distinct enzyme from neutrophil myeloperoxidase (MPO) (17–19), it shares with MPO the unique ability to use halides or pseudohalides (X–) and H2O2 derived from the respiratory burst to generate cytotoxic hypohalous acids (HOX): H2O2 + X– + H+ → HOX + H2O; where X = Cl–, Br–, I–, or SCN–). In cell-free models, halogenating oxidants formed by the EPO-H2O2-X– system are effective cytotoxins for multiple targets such as multicellular worms or parasites, bacteria, viruses, and host cells (1, 11–16, 20).
Nearly two decades ago, Weiss and colleagues first suggested a potential role for bromide (Br–) in mammalian systems (2). Eosinophils were shown to use bromide instead of chloride to generate a halogenating oxidant despite a greater than 1,000-fold excess of chloride. In contrast, neutrophils selectively use chloride (Cl–) at plasma levels of halides to generate chlorinating oxidants (21–23). Studies using chemical trapping agents demonstrated that at least 25% of the oxygen consumed by isolated human eosinophils stimulated in media possessing plasma levels of bromide and chloride could be accounted for by the generation of brominating species (3). However, a role for bromide as a physiological substrate of EPO in vivo has been questioned since the discovery that the peroxidase preferentially uses the pseudohalide thiocyanate (SCN–) (24), an abundant anion in plasma and interstitial fluids, at concentrations of halides normally found in plasma and extracellular fluids (100 mM Cl–, 20–150 μM Br–, 0.1–0.6 μM I–, and 0–70 μM SCN–; ref. 25).
Direct chemical evidence of how eosinophils participate in tissue damage in vivo is lacking. We hypothesized that brominated products might serve as a powerful tool to identify sites of eosinophil-mediated oxidative damage in vivo. We now demonstrate that eosinophil activation in vitro and in vivo results in oxidative damage of proteins through bromination of tyrosine residues, a heretofore unrecognized pathway for covalent modification of biologic targets in human health or disease.
Methods
Eosinophil and neutrophil isolation.
Neutrophils were isolated by buoyant density centrifugation as described previously (26). Low levels of contaminating eosinophils were then removed by fluorescence activated cell sorting (27). Eosinophils were isolated from whole blood obtained from normal healthy volunteers using CD16 microbeads (Miltenyi Biotec, Auburn, California, USA), as described elsewhere (28). Neutrophil and eosinophil preparations were shown cytologically to be at least 99% pure. In addition, SDS-PAGE with in-gel tetramethylbenzidine peroxidase staining (29) was performed on detergent extracts of eosinophil and neutrophil preparations to confirm that no detectable cross contamination of leukocyte peroxidase activity was observed.
Cell experiments.
Studies with activated leukocytes were performed at 37°C in Hanks’ balanced salt solution (pH 7.2; magnesium-, calcium-, phenol red- and bicarbonate-free; GIBCO BRL, Gaithersburg, Maryland, USA) supplemented with 100 μM diethylenetriaminepentaacetic acid (DTPA). Studies using inductively coupled plasma mass spectrometry confirmed that the contaminating level of Br– is this media was approximately 6–8 μM (sum of 79Br and 81Br isotopomers). Cells (1 × 106/mL) were incubated with BSA (1 mg/mL) and the indicated additional concentrations of NaBr. Cells were activated by addition of PMA (200 nM). Superoxide generation by activated human eosinophils was measured as the superoxide dismutase-inhibitable reduction of ferricytochrome c (30).
Sample preparation and mass spectrometry.
The 3-chlorotyrosine content of proteins in bronchoalveolar lavage (BAL) was determined by stable isotope dilution gas chromatography/mass spectrometry (GC/MS) (31). 3-Chloro[13C6]tyrosine standard was prepared and isolated following exposure of L[13C6]tyrosine to HOCl (1:1, mol/mol) in 20 mM phosphoric acid (32). 3-Bromo[13C6]tyrosine and 3,5-dibromo[13C6]tyrosine standards for analyses were similarly synthesized by exposure of L-[13C6]tyrosine to HOBr (1:1, mol/mol), and then isolated by preparative reverse-phase HPLC (33). The content of 3-bromotyrosine, 3,5-dibromotyrosine, and tyrosine in reaction mixtures and BAL samples were determined by GC/MS (33). Briefly, proteins were first precipitated and desalted twice (to remove residual Cl– and Br–) with a single-phase extraction mixture composed of H2O:methanol:H2O-saturated diethyl ether (1:3:7, vol/vol/vol). Samples were supplemented with 50 pmol of each internal standard, and then proteins were hydrolyzed in 4 N methane sulfonic acid (0.5 mL) supplemented with 1% phenol for 24 hours at 110°C under an argon atmosphere. Protein hydrolysates were adjusted to 2 ml volume with 0.1% trifluoroacetic acid (TFA) and passed over a mini solid-phase C18 extraction column (Supelclean LC-18 SPE tubes; 3 mL; Supelco Inc., Bellefonte, Pennsylvania, USA) equilibrated with 0.1% TFA. Columns were washed with 2 mL 0.1% TFA and amino acids were eluted with 2 mL H2O:methanol (1:1, vol/vol) supplemented with 0.1% TFA. The amino acid solution was dried under vacuum and immediately derivatized. GC/MS analyses of L-tyrosine oxidation products were performed after derivatization to their n-propyl per heptafluorylbutyryl or n-propyl per pentafluorylproprionyl derivatives (34) on a Finnigan Voyager GC/MS in the negative ion-chemical ionization mode. Results are normalized to the content of the precursor amino acid, L-tyrosine, which was similarly quantified by GC/MS (31) except for using L[13C915N]tyrosine as internal standard. In all studies, intrapreparative formation of 3-bromo[13C915N]tyrosine, 3,5-dibromo[13C915N]tyrosine, or 3-chloro[13C915N]tyrosine was negligible (i.e., < 5% of the level of the natural abundance product observed).
Subjects.
BAL specimens examined in this study were obtained as part of a separate clinical investigation that was described recently (35). Eight asthmatic and six healthy control subjects were enrolled. Because the amount of lavage obtained from some subjects was limiting, not all measures could be performed on every sample. The number of samples evaluated for each parameter is stated in figure and table legends. To be enrolled, asthmatic individuals had to have shown greater than or equal to both a 200-mL increase and a 12% increase in absolute forced expiratory volume in 1 second (FEV1) either spontaneously or after bronchodilator within the year before enrollment, tested positive on an allergy prick skin test for multiple aeroallergens, and have satisfied the definition of asthma as defined by the National Institutes of Health guidelines (36). Asthma severity and temporal course in volunteers included mild intermittent and mild persistent asthma (36). Asthmatic individuals had not received oral or intravenous corticosteroids within the past 6 months. All asthmatic individuals used short-acting inhaled β2-agonists on an as-needed basis, but did not use β2-agonist medication on the day of bronchoscopic study. Healthy controls were nonsmokers and had a negative skin test and a negative methacholine challenge test.
Segmental allergen challenge model.
Allergic asthmatics first underwent whole lung allergen challenge in which escalating doses of antigen were given to demonstrate antigen responsiveness and to determine the provocative dose that caused a 20% reduction in FEV1 (35). After at least a 4-week “cool off” period, 10% of this dose was then diluted into 10 mL normal saline and used for segmental allergen challenge via fiberoptic bronchoscopy (see later here). Healthy nonallergic controls were challenged with 100 protein nitrogen units (PNUs) of either ragweed or grass allergen (Greer Laboratories, Lenoir, North Carolina, USA). The average age, baseline FEV1 (% predicted), and percent fall in FEV1 at maximum antigen dose during whole lung antigen challenge in BAL 48 hours after allergen challenge in asthmatic versus control subjects were as follows: 36.6 ± 6.7 vs. 42.5 ± 8.6 years old; 86.6 ± 15.1% vs. 98.7 ± 14.3%; and –26.6 ± 6.9% vs. 0.3 ± 4.6%, respectively (35).
Healthy control and allergic asthmatic subjects underwent fiberoptic bronchoscopy, and a specific segment in the left lung (lingula, superior subsegment) was lavaged with normal saline to obtain a baseline sample (t = 0 hours). Normal saline (10 mL) was then instilled into an adjacent subsegment (lingula, inferior subsegment) as a control “normal saline challenge.” Specific segments in the contralateral lung (right middle lobe, medial and lateral subsegments) were then each exposed to a known allergen (Ag) in 10 mL normal saline. The medial subsegment of the right middle lobe was lavaged 10 minutes later with normal saline (10 minutes) to assess the immediate effect of allergen challenge. Forty-eight hours later, fiberoptic bronchoscopy was repeated and both allergen-challenged (right middle lobe, lateral subsegment) and normal saline–challenged (lingula, inferior subsegment) lung segments were lavaged with normal saline. Immediately after BAL was recovered, cells were removed by centrifugation and the supernatant stored in the presence of antioxidants and peroxidase inhibitors at –70°C (100 μM DTPA [pH 7.0], 100 μM butylated hydroxytoluene added from a 100 × ethanolic stock, and 10 mM 3-aminotriazole) (37) until analysis. To calculate epithelial lining fluid (ELF) volume, the concentration of urea in paired blood and BAL samples were determined using a blood urea nitrogen assay (Sigma Diagnostics, St. Louis, Missouri, USA). The study was approved by the Cleveland Clinic Foundation Institutional Review Board, and subjects gave written informed consent.
Analysis of endobronchial biopsy specimens.
Endobronchial biopsy specimens were obtained 48 hours after antigen (and normal saline) challenge. Specific autofluorescence imaging of eosinophil peroxidase in endobronchial biopsies was performed as described elsewhere (38–40). EPO-specific in situ peroxidase staining of tissues was performed after treatment of slides with 0.01 M KCN to inhibit MPO (41, 42).
Statistics.
Data represent the mean ± SD of the indicated number of samples. Statistical analyses were made using a paired two-tailed Student’s t test. For all hypotheses, the significance level was 0.05. When multiple comparisons were made, a Bonferroni correction to the significance criterion for each test was made.
Results
Eosinophils brominate protein tyrosine residues.
Structural identification of stable oxidation products formed by the action of activated eosinophils on target proteins is lacking. Recently, we identified 3-bromotyrosine and 3,5-dibromotyrosine as products formed on proteins exposed to either chemical brominating agents (e.g., HOBr, N-bromo amines, and N,N-dibromo amines) or a cell-free system composed of isolated EPO and the cosubstrates H2O2 and Br– (33). We therefore first investigated whether protein tyrosine residues served as endogenous targets for covalent modification by eosinophils activated in media supplemented with physiological concentrations of serum albumin and halides (Cl– and Br–). GC-MS analysis demonstrated that eosinophils readily modified protein tyrosine residues forming both brominated products, 3-bromotyrosine and 3,5-dibromotyrosine (Figure (Figure1).1). Product identity was confirmed by demonstrating the anticipated mass spectrometry of the derivatized brominated amino acids (Figure (Figure1,1, c and d). The time course of eosinophil-mediated tyrosine bromination paralleled the rate of superoxide (O2•–) production by the phagocytes during a respiratory burst (Figure (Figure1a).1a). Furthermore, formation of protein-bound 3-bromotyrosine demonstrated an absolute requirement for cell activation and Br– and was inhibited by the H2O2 scavenger catalase and EPO inhibitors such as azide and aminotriazole (Figure (Figure1b).1b). These results are consistent with eosinophils using the EPO-H2O2-Br– system to brominate protein tyrosine residues at plasma levels of halides.
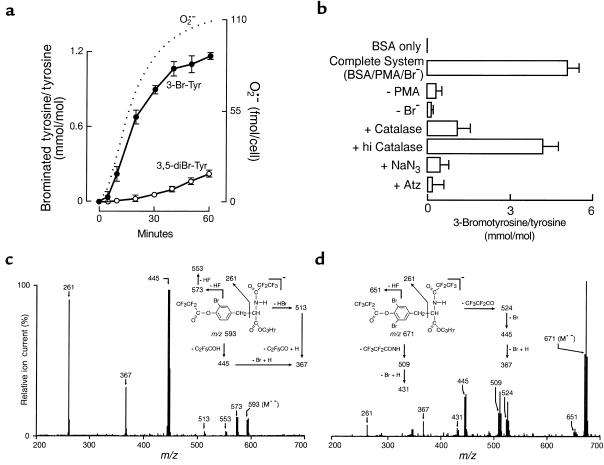
Activated eosinophils utilize plasma levels of bromide to covalently modify protein tyrosine residues. (a) Human eosinophils (1 × 106/mL) were incubated at 37°C in Hanks’ balanced salt solution supplemented with DTPA (100 μM), BSA, 1 mg/mL, and bromide (100 μM NaBr). Eosinophils were activated with PMA (200 nM) and maintained in suspensions by intermittent inversion (Complete System). At the indicated times, eosinophils were removed by centrifugation. Supernatants were delipidated, desalted, and hydrolyzed, and the content of protein-bound 3-bromotyrosine and 3,5-dibromotyrosine was determined by stable isotope dilution GC-MS analysis. In a parallel set of experiments, superoxide production (in the absence of bromide and BSA) was determined as the superoxide dismutase-inhibitable reduction of ferricytochrome c. Data represent the mean ± SD of triplicate determinations (3-bromotyrosine and 3,5-dibromotyrosine) or a representative time course of O2•– production from an experiment performed at least four times. (b) The content of 3-bromotyrosine generated on target proteins after 2-hour incubation in the Complete System was determined by stable isotope dilution GC-MS analysis. Additions or deletions to the Complete System were as indicated. The final concentrations of additions were: catalase and heat inactivated catalase (hi Catalase), 10 μg/mL; NaN3, 1 mM; 3-aminotriazole (Atz), 10 mM. Values are the mean ± SD of triplicate determinations. (c and d) Electron capture negative ion chemical ionization mass spectrum of n-propyl, per pentafluoroproprionyl derivatized (c) 3-bromotyrosine and (d) 3,5-dibromotyrosine recovered in amino acid hydrolysates of BSA exposed to activated eosinophils. (Insets) structures and proposed fragmentation pathways for derivatized (c) 3-bromotyrosine and (d) 3,5-dibromotyrosine.
3-Bromotyrosine serves as a “molecular fingerprint” for eosinophil-dependent oxidative damage of proteins.
To examine the selectivity of 3-bromotyrosine as a marker for protein damage by eosinophils, the capacity of isolated leukocytes (eosinophils and neutrophils) to generate 3-bromotyrosine and 3-chlorotyrosine, a specific marker for MPO-catalyzed protein oxidation (32), was determined. Eosinophils readily formed protein-bound 3-bromotyrosine at plasma levels of Cl– and Br–, whereas little 3-chlorotyrosine was formed (Figure (Figure2a).2a). In contrast, neutrophils formed 3-chlorotyrosine, but failed to generate significant levels of 3-bromotyrosine across the physiological range of Br– concentrations (Figure (Figure2b).2b). These results suggest that 3-bromotyrosine and 3-chlorotyrosine may serve as selective “molecular fingerprints” to identify sites of tissue injury by the leukocyte peroxidases EPO and MPO, respectively.
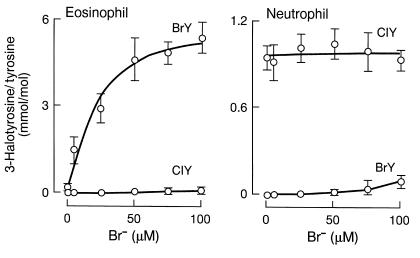
Specificity of 3-bromotyrosine and 3-chlorotyrosine formation on proteins exposed to activated human eosinophils and neutrophils. Human eosinophils and neutrophils (1 × 106/mL) were individually incubated for 2 hours at 37°C in Hanks’ balanced salt solution supplemented with DTPA (100 μM), BSA (1 mg/mL), and the indicated concentrations of bromide (0–100 μM NaBr). Leukocytes were activated with PMA (200 nM) and maintained in suspensions by intermittent inversion. The content of protein-bound 3-bromotyrosine and 3-chlorotyrosine was then determined by stable isotope dilution GC-MS analysis. Data represent the mean ± SD of triplicate determinations.
Brominating oxidants are formed in allergen-induced asthma.
To evaluate whether brominating oxidants play a role in promoting oxidative damage in vivo, we used a provocative model for human asthma exacerbation that elicits a dramatic eosinophilic response, segmental allergen challenge (43–46). In this model, human subjects (healthy control and allergic asthmatic) undergo fiberoptic bronchoscopy, and a specific segment of one lung is challenged by administration of a predetermined allergen at a known dose and for a set time interval. A lung segment in the contralateral lung is similarly challenged with normal saline. Forty-eight hours later, fiberoptic bronchoscopy is repeated and both allergen- and normal saline–challenged lung segments are lavaged with normal saline and biopsied. Specimens examined in this study were obtained as part of a clinical investigation that was described recently (35). Control and allergic asthmatic (non–steroid-dependent) subjects were age- and sex-matched, nonsmokers, and demonstrated similar BAL cell counts and differentials at baseline (Table (Table1).1). After allergen challenge, but not normal saline challenge, a robust infiltration of eosinophils in allergic asthmatic subjects was revealed by histological examination of endobronchial biopsy specimens (Figure (Figure3,3, a and b) and cytological examination of BAL fluids (Table (Table1).1). In contrast, examination of endobronchial biopsies and BAL from healthy control (nonasthmatic) subjects demonstrated no significant eosinophilia (≤ one eosinophil per high power field) and negligible (<0.5%) levels of eosinophils, respectively, after both allergen and normal saline challenge (data not shown).
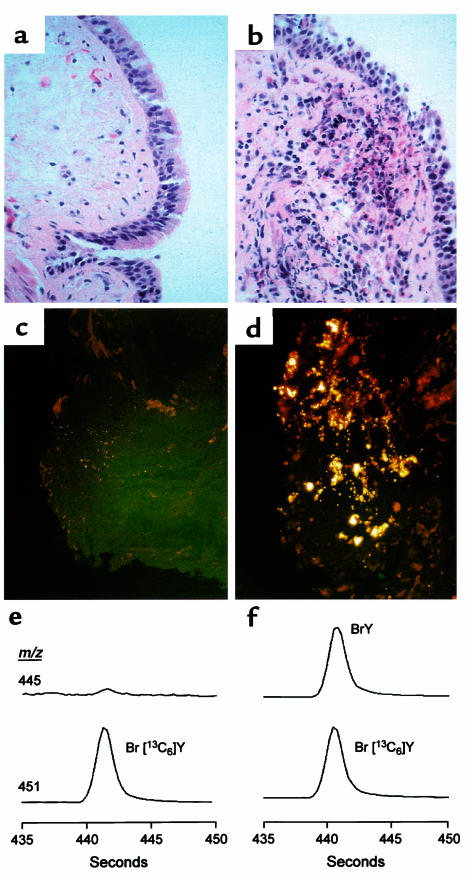
Effect of localized allergen challenge on allergic asthmatic airway and BAL proteins. An allergic asthmatic subject underwent fiberoptic bronchoscopy and ragweed allergen was instilled into a specific segment of one lung. A segment in the contralateral lung was similarly challenged with normal saline. Forty-eight hours later, fiberoptic bronchoscopy was repeated and both allergen- and normal saline–challenged lung segments were lavaged with normal saline and biopsied. (a and b) Hematoxylin and eosin staining of (a) normal saline- and (b) allergen-challenged lung segments reveals intense leukocyte infiltration and red granular debris from eosinophils in the allergen-challenged segment. High-power magnification view (data not shown) demonstrated that the majority of leukocytes recruited to the allergen-challenged airways were eosinophils. (c and d) Histological analysis of (c) normal saline– and (d) allergen-challenged lung segments by in situ fluorescence microscopy under conditions specific for the heme moiety of EPO reveals intense fluorescence signal in the allergen-challenged segment. (e and f) Protein recovered in BAL fluid from (e) normal saline– and (f) allergen-challenged lung segments of an asthmatic subject were analyzed by stable isotope dilution GC-MS for the presence of 3-bromotyrosine (BrY) using selected ion monitoring mode. The chromatograms shown were monitored at m/z 445, the base ion for the n-propyl, per pentafluoroproprionyl derivative of 3-bromotyrosine (Figure (Figure1c),1c), as well as the corresponding isotopically enriched counterpart at m/z 451 derived from the internal standard, 3-bromo[13C6]tyrosine.
Table 1
Nonasthmatics Asthmatics
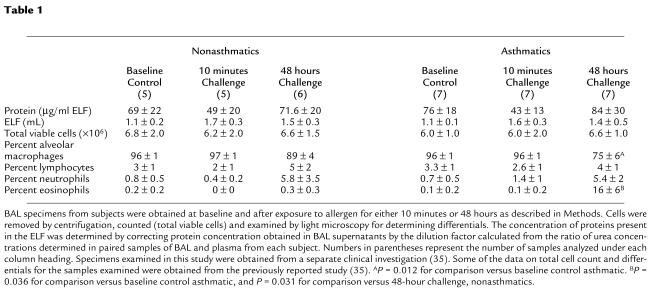
To determine whether EPO is enriched in airways of allergen-challenged, but not normal saline–challenged, allergic asthmatic subjects, several studies were performed. First, endobronchial biopsy specimens were examined by fluorescence microscopy using excitation and emission wavelengths specific for the autofluorescence of the porphyrin group of EPO (38–40). Dramatic increases in fluorescence intensity were observed in allergen-challenged, but not normal saline–challenged, specimens from allergic asthmatic subjects (Figure (Figure3,3, c and d). Endobronchial biopsy specimens were also examined by staining for in situ peroxidase activity under conditions specific for EPO activity (41, 42). Staining was intense only in biopsies recovered from allergen-challenged lung segments (48 hours) of allergic asthmatic subjects and colocalized with eosinophils (data not shown).
Eosinophil infiltration into the airways of asthmatic subjects after provocation with allergen does not indicate whether the phagocytes are active and promote oxidative damage of proteins in vivo. To test whether EPO-generated brominating oxidants are formed in response to allergen-triggered eosinophil recruitment to asthmatic airways, the content of 3-bromotyrosine in proteins present in BAL (after removal of cells) were analyzed by stable isotope dilution GC/MS analysis. Typical GC/MS chromatograms obtained using selected ion monitoring for specific fragment ions derived from derivatized 3-bromotyrosine (mass-to-charge ratio [m/z] 445) and a synthetic heavy isotope-labeled internal standard, 3-Br-[13C6]tyrosine (m/z 451), are illustrated in Figure Figure3,3, e and f. Ions with the appropriate m/z and retention time for 3-bromotyrosine were readily observed in BAL recovered from lung segments of asthmatic subjects 48 hours after allergen challenge, but not normal saline challenge. Thus, brominating oxidants are formed after antigen-exposure in the airways of allergic asthmatic individuals.
The content of 3-bromotyrosine present in BAL proteins recovered at baseline (t = 0 hours) and after segmental allergen challenge (10 minutes and 48 hours) in both nonasthmatic controls and asthmatic subjects are shown in Figure Figure4.4. At baseline, levels of 3-bromotyrosine in BAL proteins from mild allergic asthmatic individuals showed a tendency toward being modestly elevated over those observed in healthy control subjects; however, the difference failed to reach statistical significance (116 ± 84 vs. 47 ± 79 μmol/mol tyrosine, baseline asthmatic versus control, respectively; mean ± SD, P = 0.084; n = six individuals per group). Immediately after allergen exposure (10 minutes), no significant formation of 3-bromotyrosine was observed in BAL proteins recovered from either nonasthmatic or asthmatic subjects (Figure (Figure4).4). In contrast, a dramatic increase in protein modification by brominating oxidants was observed in asthmatic subjects 48 hours after allergen exposure (1,085 ± 365 vs. 32 ± 42 μmol/mol tyrosine; 48 hours after allergen asthmatic versus control, respectively; mean ± SD, P = 0.0003; n = six individuals per group) (Figure (Figure44).
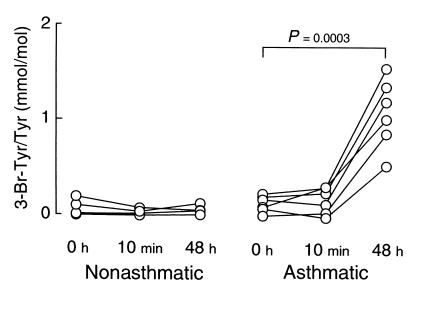
Quantification of 3-bromotyrosine content in proteins recovered from nonasthmatic and asthmatic subjects at baseline and after segmental allergen challenge. Healthy control and allergic asthmatic subjects underwent fiberoptic bronchoscopy, and a specific segment of one lung was lavaged with normal saline to obtain a baseline sample (t = 0 hours). Two specific segments in the contralateral lung were then each exposed to allergen. One of these was lavaged 10 minutes later with normal saline (10 minutes) to assess the immediate effect of allergen challenge. Forty-eight hours later, fiberoptic bronchoscopy was repeated and the other allergen-challenged lung segment was lavaged with normal saline (48 hours). Cells in the BAL were removed by centrifugation, and the content of 3-bromotyrosine on proteins recovered in the supernatant at baseline and after segmental allergen challenge was then determined by stable isotope dilution GC/MS. P values represent the comparison between t = 0 versus 48 hours for allergen-challenged lung segments in asthmatic subjects (n = six per group).
Allergen challenge elicits primarily an eosinophilic response in tissues; however, the content of neutrophils in BAL and endobronchial tissues also rose modestly, though at comparable levels in nonasthmatic and asthmatic subjects (Table (Table1).1). Because the concentration of halides in bronchial tissues is not known, and neutrophils can generate low levels of brominating oxidants at plasma levels of halides (Figure (Figure2),2), we could not exclude the possibility that neutrophils were participating in generating brominating oxidants in vivo. To assess the relative contribution of neutrophils versus eosinophils to protein modification by halogenating species, we compared the content of 3-chlorotyrosine and 3-bromotyrosine in BAL recovered from control and allergic asthmatic subjects 48 hours after challenge with either normal saline or allergen (Figure (Figure5).5). Provocation with allergen (but not normal saline) resulted in a significant two- to threefold increase in the content of 3-chlorotyrosine in BAL recovered from allergic asthmatic (but not healthy control) subjects (276 ± 96 vs. 101 ± 48 μmol/mol tyrosine; asthmatic baseline versus 48 hours after allergen, respectively; mean ± SD, P = 0.024; n = six individuals per group). Thus, MPO-dependent oxidative damage of proteins occurs after allergen exposure in allergic asthmatic individuals. However, the content of 3-bromotyrosine in BAL recovered from allergen-challenged lung segments of asthmatic subjects increased 10-fold over levels observed in BAL recovered from lung segments in the contralateral lung exposed only to normal saline (Figure (Figure5).5). Moreover, after allergen challenge, the content of 3-bromotyrosine in BAL proteins from asthmatic subjects was significantly greater than the content of 3-chlorotyrosine, consistent with eosinophils (Figure (Figure2)2) as a primary source of halogenating oxidants after allergen challenge.
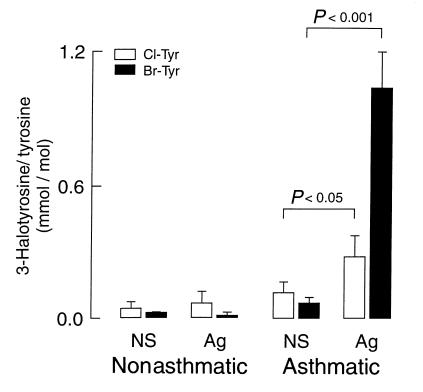
Comparison of protein modification by brominating versus chlorinating oxidants in proteins recovered 48 hours after segmental allergen-challenge. The contents of 3-bromotyrosine and 3-chlorotyrosine generated on proteins in BAL fluid recovered from normal saline (NS) and allergen-challenged (Ag) lung segments (48 hours after challenge) of nonasthmatic and asthmatic subjects were determined by stable isotope dilution GC/MS. Data represent the mean ± SD (n = six for each group). Exposure to allergen only caused significant increases in bromination and chlorination of proteins recovered from asthmatic subjects. n = six per group.
Discussion
Bromide is the fifth most abundant inorganic anion in human plasma and tissues (following chloride, bicarbonate, phosphate, and sulfate) (47). This ubiquitous halide is present at 20–150 μM in plasma, constitutes approximately 0.1% of table salt, and is abundant in sea and ocean waters (48). Brominated halometabolites are common components of many marine organisms and prokaryotes (48–54). However, direct (in vivo) demonstration that the element bromine plays a physiological role in mammals has not yet been established. The results of the present study identify brominating species produced by eosinophils as a distinct class of oxidants formed in vivo. They also demonstrate that bromination of protein tyrosine residues represents a novel form of posttranslational modification of proteins at sites of eosinophil recruitment and activation in humans.
The physiological consequences of EPO-dependent formation of brominating oxidants such as HOBr in vivo are unknown. HOBr reacts rapidly with a variety of nucleophilic targets present in biologic matrices such as thiols, thiol ethers, amines, unsaturated groups, and aromatic compounds (33, 55, 56). Thus, EPO-dependent formation of HOBr from resident eosinophils may directly contribute to both the rapid loss of reduced glutathione and the rapid inactivation of superoxide dismutase activity observed during antigen-induced asthmatic responses in humans (57). In addition, interaction of HOBr with targets such as amino acids and H2O2 can generate secondary reactive species such as N-bromoamines (55, 56), aldehydes (58), and singlet oxygen (59, 60) that may likewise contribute to events in allergic responses. In vitro studies demonstrate that the EPO-H2O2-Br– system possesses potent antibacteriacidal, -fungicidal, -parasitic, and -viricidal activities (1, 11, 12, 14, 15, 20). However, the potential beneficial aspects of these activities within the setting of an allergen-triggered asthma exacerbation are less clear. This is particularly true given the known toxicity of HOBr and the EPO-H2O2-Br– system to eukaryotic cells in culture (4, 16), and their capacity to elicit many of the pathophysiological features of asthma, including epithelial cell damage and sloughing, airway hyperreactivity, bronchoconstriction, β-adrenergic dysfunction, mucus hypersecretion, microvascular leak, and edema (61–66).
Similarly, the consequences of tyrosine bromination in vivo are unknown. One obvious potential function might be the modulation of tyrosine kinase- or phosphatase-dependent signaling. Alternatively, it is tempting to speculate that EPO-dependent bromination of tyrosine residues evolved because it may confer an activity that contributes to host defenses. Multiple distinct brominated halometabolites (including brominated tyrosine analogs) possessing antimicrobial activities have been identified in marine organisms such as algae, sponges, bryozoans, tunicates, worms, and molluscs (48–54, 67). Synthesis of these halometabolites is thought to be catalyzed by distinct bromoperoxidases that, unlike the hemoprotein EPO, utilize vanadium at their catalytic centers. The high concentrations of halides in seawater has thus apparently been exploited during evolution to promote halogenation of organic molecules as a favored biosynthetic process in these organisms. Whether bromination of targets in mammalian systems confers similar benefits remains to be determined.
The present studies identify EPO as a catalytic source of reactive oxidant species during asthma. They thus suggest EPO as a potential therapeutic target for allergen-triggered inflammatory tissue injury in humans. The ability of halogenated oxidation products in tissues to serve as specific “molecular fingerprints” for proteins damaged by mammalian leukocyte peroxidases should serve as valuable tools in identifying the functions of EPO, MPO, and halogenating oxidants in host defenses and inflammation.
Acknowledgments
Mass spectrometry experiments were performed at the Lerner Research Institute Mass Spectrometry Facility. This work was supported in part by the American Heart Association and by the National Institutes of Health (grants HL-61878 and HL-62526).
References
Articles from The Journal of Clinical Investigation are provided here courtesy of American Society for Clinical Investigation
Full text links
Read article at publisher's site: https://doi.org/10.1172/jci9702
Read article for free, from open access legal sources, via Unpaywall:
http://www.jci.org/articles/view/9702/files/pdf
Citations & impact
Impact metrics
Citations of article over time
Alternative metrics
Article citations
Deciphering the Interplay between the Epithelial Barrier, Immune Cells, and Metabolic Mediators in Allergic Disease.
Int J Mol Sci, 25(13):6913, 24 Jun 2024
Cited by: 1 article | PMID: 39000023
Review
Misregulation of bromotyrosine compromises fertility in male <i>Drosophila</i>.
Proc Natl Acad Sci U S A, 121(21):e2322501121, 15 May 2024
Cited by: 1 article | PMID: 38748578 | PMCID: PMC11126969
Diacron-Reactive Oxygen Metabolites Levels Are Initially Elevated in Patients with Bullous Pemphigoid.
JID Innov, 4(4):100282, 16 Apr 2024
Cited by: 0 articles | PMID: 38859975
Eosinophil extracellular traps in respiratory ailment: Pathogenic mechanisms and clinical translation.
World J Otorhinolaryngol Head Neck Surg, 10(3):213-224, 01 Nov 2023
Cited by: 0 articles | PMID: 39233861 | PMCID: PMC11369806
Review Free full text in Europe PMC
Oxidative Stress and Inflammation in Acute and Chronic Lung Injuries.
Antioxidants (Basel), 12(3):548, 21 Feb 2023
Cited by: 28 articles | PMID: 36978796 | PMCID: PMC10045332
Review Free full text in Europe PMC
Go to all (177) article citations
Data
Similar Articles
To arrive at the top five similar articles we use a word-weighted algorithm to compare words from the Title and Abstract of each citation.
3-Bromotyrosine and 3,5-dibromotyrosine are major products of protein oxidation by eosinophil peroxidase: potential markers for eosinophil-dependent tissue injury in vivo.
Biochemistry, 38(12):3538-3548, 01 Mar 1999
Cited by: 99 articles | PMID: 10090740
Eosinophils are a major source of nitric oxide-derived oxidants in severe asthma: characterization of pathways available to eosinophils for generating reactive nitrogen species.
J Immunol, 166(9):5763-5772, 01 May 2001
Cited by: 166 articles | PMID: 11313420
Granulocyte function in the airways of allergen-challenged pigs: effects of inhaled and systemic budesonide.
Clin Exp Allergy, 26(12):1436-1448, 01 Dec 1996
Cited by: 3 articles | PMID: 9027445
Eosinophil-dependent bromination in the pathogenesis of asthma.
J Clin Invest, 105(10):1331-1332, 01 May 2000
Cited by: 15 articles | PMID: 10811837 | PMCID: PMC315472
Review Free full text in Europe PMC
Funding
Funders who supported this work.
NHLBI NIH HHS (2)
Grant ID: HL-62526
Grant ID: R01 HL061878