Abstract
Free full text

The Zebrafish Motility Mutant twitch once Reveals New Roles for Rapsyn in Synaptic Function
Abstract
Upon touch, twitch once zebrafish respond with one or two swimming strokes instead of typical full-blown escapes. This use-dependent fatigue is shown to be a consequence of a mutation in the tetratricopeptide domain of muscle rapsyn, inhibiting formation of subsynaptic acetylcholine receptor clusters. Physiological analysis indicates that reduced synaptic strength, attributable to loss of receptors, is augmented by a potent postsynaptic depression not seen at normal neuromuscular junctions. The synergism between these two physiological processes is causal to the use-dependent muscle fatigue. These findings offer insights into the physiological basis of human myasthenic syndrome and reveal the first demonstration of a role for rapsyn in regulating synaptic function.
The emergence of zebrafish as a vertebrate model for the study of nervous system development is attributable primarily to the readily available supply of genetic mutants (Granato and Nusslein-Volhard, 1996). From a physiological standpoint, a critical advantage offered by this species is the speed at which the nervous system develops. Thus, the functional consequences of many mutations in neuronal and synaptic proteins that are lethal in mammals can be examined in fish before death occurs. Such has been the case for functional knock-outs of voltage-dependent sodium channels (Ribera and Nusslein-Volhard, 1998), nicotinic acetylcholine receptors (AChRs) (Westerfield et al., 1990; Ono et al., 2001a), and acetylcholinesterase (Behra et al., 2002). Despite these proofs of principal, relatively few studies have used physiological approaches to determine the underpinnings of mutant behavior. This seems paradoxical in light of the fact that so many of the mutants were originally identified on the basis of defects in swimming behavior (Granato et al., 1996). Any incertitude about whether the culprit gene could eventually be identified purely on the basis of physiological investigations has been quelled by the success of studies on paralytic mutant fish. Physiological analyses of the paralytic mutant linesnic-1 (Westerfield et al., 1990), sofa potato, and relaxed (Ono et al., 2001a) have identified defects in nicotinic receptors and muscle dihydropyrine receptors. In fact, the successful identification of mutations underlying defects in motility has stemmed, in part, from the large body of knowledge about key proteins involved in nerve–muscle communication. In particular, more is known about the neuromuscular junction than any other synapse, and many of the genes encoding key players have been cloned (Burden, 1998;Sanes and Lichtman, 1999, 2001). However, even more important than identification of the mutation, physiological analyses of the mutant phenotypes have revealed new functional roles played by well characterized postsynaptic proteins (Ono et al., 2001a).
Here we used physiological analysis of twitch once fish neuromuscular synaptic transmission to identify the gene responsible for fatigue in swimming. Our findings identify a mutation in the muscle protein rapsyn that is responsible for subsynaptic clustering of the AChR. Disruption of the rapsyn–receptor interaction leads to a diffuse organization of receptors within the muscle membrane, consistent with the well established role of rapsyn in receptor clustering (Froehner et al., 1990; Phillips et al., 1991). However, disruption of rapsyn–receptor interaction in vivo revealed an additional, unexpected role for rapsyn in synaptic function. In addition to lowering synaptic strength through a reduction in subsynaptic receptor density, interference with rapsyn–receptor interaction led to pronounced frequency-dependent synaptic depression. This important additional role played by rapsyn likely went undetected because rapsyn knock-out mice die before functional synapse formation (Gautam et al., 1995; Nguyen et al., 2000). Thus, the use of mutant zebrafish has disclosed a new role for rapsyn.
MATERIALS AND METHODS
The distribution of muscle rapsyn and AChRs was determined using fluorescence microscopy. Rapsyn distribution was measured using either stable or transient expression. For stable expression of rapsyn fluorescence, a line of fish was used that expressed a murine rapsyn-green fluorescent protein (GFP) fusion protein under control of a muscle α-actin promoter (Ono et al., 2001a). For transient expression, cDNA coding for fish rapsyn-GFP fusion protein was injected into 1–16 cell stage fertilized eggs. Observation of GFP and rhodamine-based fluorescence was done as described previously (Ono et al., 2001a). For imaging of fish swimming, digital images were taken at the rate of 1000 frames/sec.
To clone the gene encoding zebrafish rapsyn, PCR primers corresponding to conserved amino acids were synthesized. The amplified PCR product from 5-d-old zebrafish cDNA was subcloned and sequenced. 5′ rapid amplification of cDNA ends (RACE) and 3′ RACE (Clontech, Palo Alto, CA) were performed to obtain the whole coding region. The GenBank accession number for zebrafish rapsyn is AB070208. This gene was used to genotype individual fish after confocal imaging or video imaging. To do this, decapitated fish were placed in solution containing 10 mm Tris, pH 8.5, 0.2% Triton X-100, and 0.2 mg/ml Proteinase K. After 1 hr incubation at 50°C, the reaction tube was boiled for 1 min and centrifuged at 10,000 × g for 1 min. The extracted genomic DNA was amplified by PCR with rapsyn primers flanking the region of G130E mutation. Amplified products were run on agarose gel, isolated, purified, and sequenced.
To construct plasmids for transient rescue experiments, the zebrafish α-actin promoter (Higashijima et al., 1997), Kozak sequence, zebrafish rapsyn gene, enhanced GFP (EGFP), and simian virus 40 early polyadenylation signal were ligated such that rapsyn and EGFP are in frame. The fish rapsyn sequence with G130E mutation was amplified from twitch once(two−/−) fish cDNA using Pfu DNA polymerase (Stratagene, La Jolla, CA). Wild-type (WT) rapsyn sequence was made by mutating the 130 E back to G. The regions coding for rapsyn were sequenced for both wild-type and G130E constructs. Plasmids were linearized upstream of α-actin promoter sequence. DNA (25 ng/μl) was pressure injected into 1–16 cell stage embryos following methods described previously (Higashijima et al., 1997).
Evoked and spontaneous synaptic currents were recorded from muscle as described previously, with slight modifications (Ono et al., 2001a). Decapitated 7- to 10-d-old fish were fixed on sylgard with tungsten needles in 100% HBSS. Skin was peeled from one side of the fish with tungsten needles. The fish was treated with 2 mformamide for 5 min to stop movement of muscle cells. After treatment, the fish was washed vigorously in HBSS and placed in a recording solution containing the following (in mm): 110 NaCl, 4 CaCl2, 2 KCl, 1 MgCl2, 4 glucose, and 5 HEPES, pH 7.4. The pipettes were filled with an internal solution containing the following (in mm): 120 KCl, 5 HEPES, and 5 BAPTA, pH 7.1. Muscle cells were voltage clamped at −90 mV for miniature endplate current (mEPC) recordings and at −50 mV for EPC recordings. Holding muscle at −50 mV minimizes any possible contamination by muscle sodium current in response to electrical stimulation.
For stimulation, two insulated 0.005-inch-diameter platinum–iridium electrodes were positioned above and below the fish so that the current flow was directed through the spinal cord. Care was taken to ensure that the stimulating and recording electrodes were located in the same muscle segment. A Grass stimulator (model SD5) was used to deliver the current. The pulse duration was set to 0.3 msec, and the amplitude was gradually increased until no additional incremental increase in EPC amplitude was seen. This ensured that all of the motor units innervating that muscle cell were recruited. The stimulus intensity required for complete recruitment of the motor neurons generally corresponded to 30–50 V. All experiments were performed in compliance with institutional guidelines.
RESULTS
Homozygous twitch once(two−/−) mutants failed to exhibit the spontaneous swimming behavior normally acquired at 3 d of age (Granato et al., 1996). These mutant fish were also unable to mount a full-blown escape response to tail touch, which is acquired normally within the first 3 d of age (Fig.(Fig.1).1). High-speed cinematic analyses of swimming patterns showed thattwo−/− fish executed only one or two bilateral flips of the tail before the escape response was abruptly terminated (Fig. (Fig.1).1). An inactive period followed, during which the fish were unresponsive to additional mechanical prodding. The lack of control over voluntary responses results in death when the fish reach ~14 d of age. The truncated escape behavior of the mutant fish differs from wild-type behavior wherein a series of repetitive tail flips in response to tail touch rapidly propels the fish out of the field of view (Fig. (Fig.1).1). Such obvious and consistent differences in behavior between the mutant and wild-type fish provided an easy initial screen for identification oftwo−/− fish for additional experimentation.
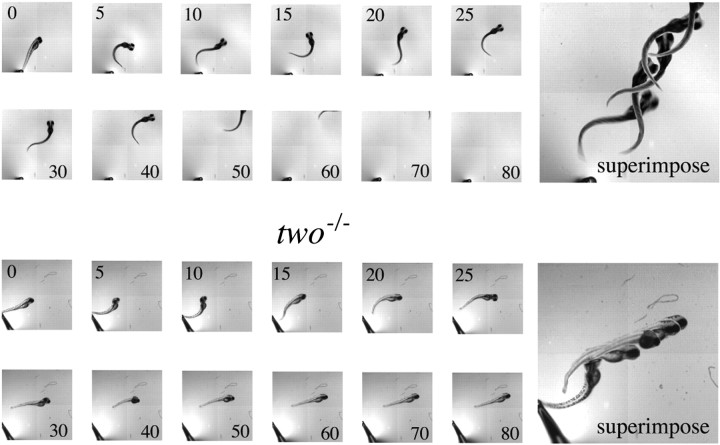
Comparisons of wild-type and two−/− fish escape responses. Five-day-old wild-type fish (top) andtwo−/− mutant fish (bottom) were each mechanically prodded to elicit escape responses. The swimming response by each fish was recorded at the indicated times (in milliseconds) after the stimulus. Superimposed images of selected frames are indicated to theright.
Synaptic currents are reduced intwo−/− fish
Recordings of synaptic currents in myotomal muscle revealed striking differences between wild-type andtwo−/− fish. Using whole-cell recordings from individual muscle cells, both the spontaneous (mEPC) and evoked (EPC) synaptic currents in mutants were compared with those of wild-type fish (Fig. (Fig.2).2). The evoked endplate currents were generated in response to suprathreshold extracellular stimulation of the spinal cord, thus ensuring that all of the primary and secondary motor neuron inputs to the individual muscle cell were activated (Ono et al., 2001a). The mean EPC amplitudes corresponded to 3.7 nA (n = 12) for wild-type and 0.48 nA (n = 13) fortwo−/− fish (Fig. (Fig.2).2). This average difference of 7.7-fold was highly statistically significant.
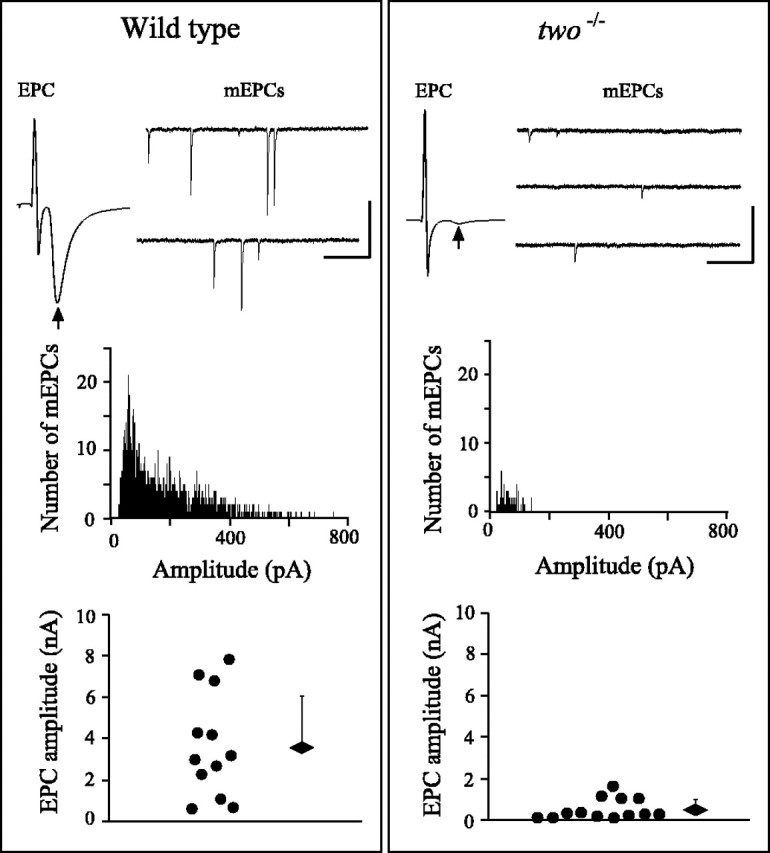
Spontaneous and evoked synaptic currents in wild-type and two−/− muscle. The properties of EPCs and mEPCs are compared for wild-type (left) and two−/−(right) fish. Top, Representative EPCs and mEPCs. The arrow indicates the position of the EPC after the stimulus artifact. Calibration: EPC, 2 msec, 5 nA; mEPC, 100 msec, 100 pA. Middle, Frequency histograms of mEPC amplitude for wild-type and two−/−fish. Bottom, Scatter plot showing the largest EPC amplitude from each recording in 12 wild-type and 13two−/− different fish. The overall average ± SD for wild-type andtwo−/− fish are indicated by thediamonds and bars.
Both the amplitude and frequency of spontaneous mEPCs differed between wild-type and two−/− fish. Specifically, the mEPC frequency was much lower intwo−/− fish (Fig. (Fig.2).2). Furthermore, as seen for the EPCs, the average mEPC event amplitude was greatly reduced (Fig. (Fig.2).2). In wild-type fish, the mEPC amplitudes ranged from 23 to 752 pA, with the smaller-amplitude mEPCs contributing the majority of the events. In two−/− fish, it was difficult to obtain large numbers of mEPCs in a single recording attributable to a low frequency of detectable events. For those mEPCs detected, the amplitudes ranged from 23 to 143 pA, representing an ~2.7-fold reduction in mean amplitude compared with wild-type muscle. It is likely that the apparent reduction in mEPC frequency was a consequence of this decreased amplitude, such that only the largest events were able to be distinguished from the noise.
Postsynaptic ACh receptors are diffusely distributed intwo−/− zebrafish
The postsynaptic distribution of ACh receptors was compared between two−/− and wild-type fish to identify any differences that might account for the reduction in synaptic response amplitude. For this purpose, the AChRs in myotomal muscle were labeled with rhodamine-conjugated α-bungarotoxin (Rh-α-Btx) (Ono et al., 2001a). The muscle was exposed to toxin by selective removal of skin on one side of the fish such that the muscle on the other, intact side of the fish could be viewed using confocal scanning microscopy. In this manner, the fluorescence distribution of AChRs was determined without disturbing the muscle, nerve, or endplates. For the purpose of orientation, a region of tail comprising three to four myotomes and the individual muscle cells are shown in Figure Figure3.3. The labeled receptors in 5-d-old wild-type fish showed well organized clusters on the muscle cell surface. The predominant labeling is observed at the ends of muscle cells, as well as punctate locations along the surface. These regions correspond to the locations of neuromuscular junctions (Ono et al., 2001a). In contrast, the receptors in 5-d-oldtwo−/− myotomal muscle were diffusely distributed along the plasma membrane edge of muscle (Fig. (Fig.3)3) (see Fig. Fig.7).7). The apparent clusters within the muscle cells likely represent optical slices through the invaginating T-tubular membrane rather than true receptor clusters. Unlike the wild-type fish examined, there was no evidence for formation of subsynaptic receptor aggregates in two−/− fish between the ages of 4 and 8 d.
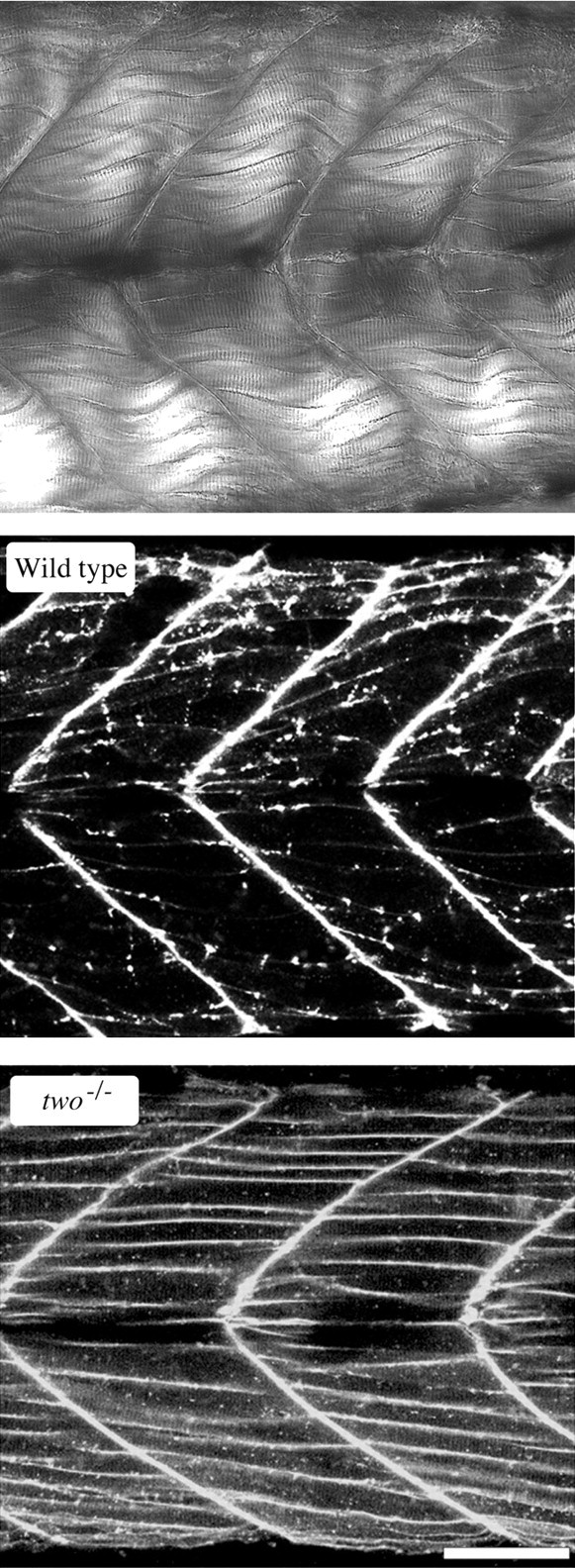
Distribution of ACh receptors in the myotomal muscle of wild-type and two−/−fish. Top, A differential interference contrast image of a section of the tail musculature is shown for orientation of the fluorescence images. This image spans approximately four chevron-shaped segments or myotomes and is are similar to the image area of the accompanying fluorescence photos. Layers of individual muscle cells can be resolved between the myocomata that separate tail segments. Confocal fluorescence image showing the distribution of rhodamine-conjugated α-bungarotoxin labeling in the tail of wild-type (middle) and two−/−mutant (bottom) fish. Scale bar, 50 μm.
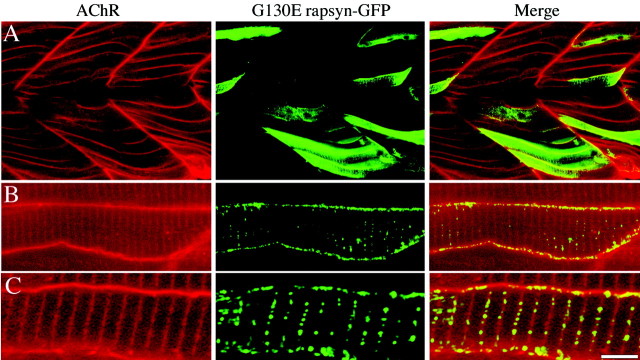
Transient expression of G130E rapsyn intwo−/− fish. 1–16 cell stage fish embryos were microinjected with DNA constructs encoding G130E rapsyn-GFP. Fish embryos were examined by confocal microscopy on day 5 for distribution of rapsyn and receptors. A, Low-power confocal fluorescence images of redrhodamine-conjugated α-bungarotoxin (left),green rapsyn-GFP (middle), and merged images (right). Note the absence of localized rapsyn and receptor in the low-power images. Intermediate (B) and higher (C) -magnification images show small clusters of greenrapsyn, with no associated clusters of red receptor, along the edge of muscle. The presence of green clusters on a diffuse red background leads toyellow clusters on the merge. The coincidentgreen and red fluorescence within the muscle represents an optical slice through invaginating T-tubular membrane. Scale bar: A, 50 μm; B, 10 μm; C, 5 μm.
The lack of synaptic receptor aggregates could be a consequence of reduced innervation by motor neurons, although some innervation would have to be maintained because of the presence of synaptic responses. To examine the innervation pattern, we exploited the availability of a stable line of zebrafish expressing GFP under control of the neuronal-specific Islet-1 gene promoter (Higashijima et al., 2000). In this line, the majority of secondary motor neurons are brightly fluorescent (Ono et al., 2001a). This line was crossed with the twitch once zebrafish line to produce mutant fish with GFP fluorescent motor neurons. No obvious differences were observed in the motor neuron patterns of wild-type andtwo−/− fish expressing GFP (data not shown). Moreover, no clusters of AChRs colocalized beneath the nerve terminals in two−/− fish, further distinguishing this mutant from wild-type fish (Ono et al., 2001a). Rapsyn is a subunit associated with AChRs that is required for subsynaptic clustering of the receptor (Burden et al., 1983; Froehner et al., 1990; Phillips et al., 1991; Gautam et al., 1995). The unusual AChR distribution in two−/− muscle offered a unique opportunity to test, in normally innervated muscle, whether extrasynaptic AChRs have the ability to associate with rapsyn. To test this idea, we once again exploited a preexisting stable line of zebrafish. This line expresses exogenous murine muscle rapsyn fused to GFP. The rapsyn-GFP fusion protein expresses to very high levels specifically in skeletal muscle because it is under control of the muscle actin promoter (Ono et al., 2001a). In wild-type fish background, the rapsyn-GFP faithfully colocalized with the high-density subsynaptic AChRs, and expression of either nonsynaptic AChR or rapsyn-GFP was minimal (Ono et al., 2001a). In progeny from matings oftwo+/−/ rapsyn-GFP fish with two+/− fish, 50% of the fish with two−/− swimming patterns were expected to also express the rapsyn-GFP gene. This assumes that the genes sort independently. In fact, of 314 offspring tested, 16% of the embryos showed a swimming pattern typical oftwo−/− fish, but in none of these fish was rapsyn-GFP fluorescence detected. All fish showing rapsyn-GFP-positive fluorescence in myotomal muscle were able to mount escape responses. More careful measurements of swimming patterns indicated that ~25% of the GFP-positive fish exhibited escape responses that were more sluggish than typical wild-type responses (Fig.(Fig.44A). These collective findings suggested that the rapsyn-GFP transgene rescued thetwitch once phenotype. Subsequent genotypic analysis of the sluggish zebrafish (see below) confirmed that these rescued fish weretwo−/−. Additionally, Rh-α-Btx labeling in these sluggish fish indicated a wild-type-like distribution of AChRs. Specifically, the receptors were effectively clustered at synaptic locations in the muscle (Fig. (Fig.44B).
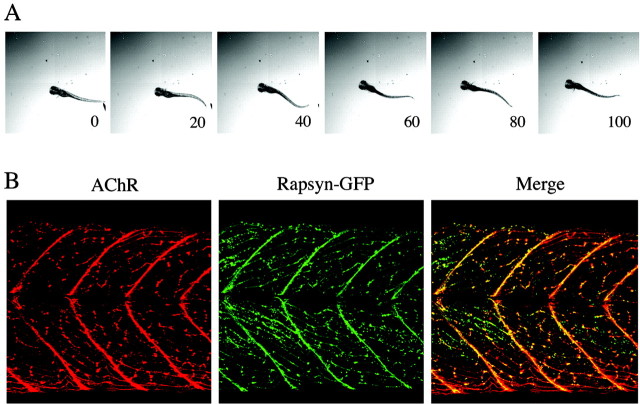
Stable expression of a murine rapsyn-GFP fusion protein rescues two−/−.A, The escape response of atwo−/− fish expressing murine rapsyn-GFP is recorded at day 5. Sequential images taken at 20 msec intervals are shown after the start of movement. B, Confocal fluorescence images showing the redfluorescence associated with AChRs (left) and thegreen fluorescence associated with rapsyn-GFP (middle) in the fish shown in A. The merged images (right) reveal the striking colocalization of the receptor–rapsyn distribution in thistwo−/−/rapsyn-GFP fish.
Mutant rapsyn self-aggregates but fails to aggregate AChRs
To further test the idea that a rapsyn defect was causal to thetwitch once phenotype, rapsyn cDNA from wild-type zebrafish was cloned on the basis of homology with other species. Translated amino acid sequence of cloned zebrafish rapsyn showed 74% similarity with mouse rapsyn. Comparison of the amino acid sequences between wild-type and twitch once rapsyn revealed a single amino acid difference at position 130 wherein a glycine (G) in the wild-type sequence was replaced by a glutamate residue (E) in the mutant sequence (Fig. (Fig.5).5). It was the presence of this mutation that allowed us to genotypically distinguishtwo−/− from wild-type ortwo+/− fish in the behavioral and cell biological analyses described above (Figs. (Figs.4,4, ,55B)
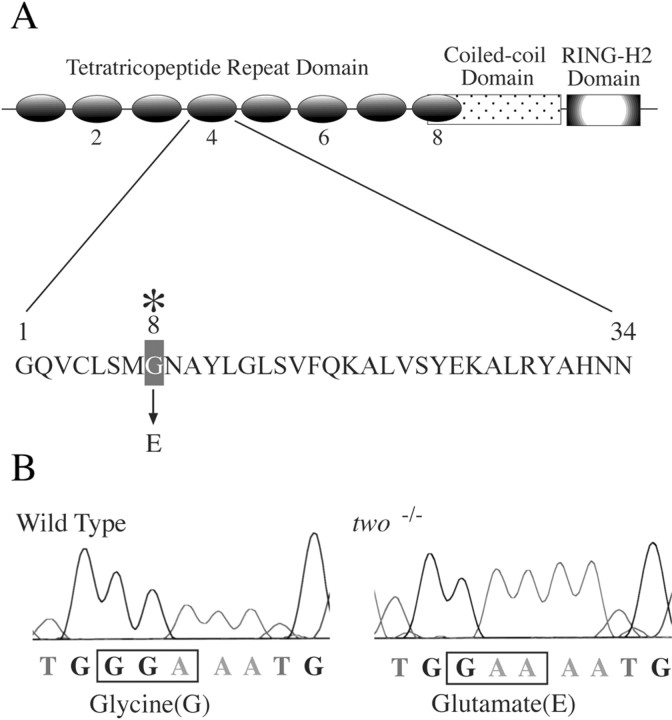
Identification of the mutation in thetwitch once rapsyn gene. A, The rapsyn protein motifs are shown, as well as the location of twitch once (twoth26e) mutation in the TPR domain. The 34 residue sequence of the fourth TPR domain is shown. In twitch once rapsyn, the eighth residue in the fourth TPR domain is mutated from glycine to glutamate.B, Raw chromatogram of sequences used to genotype wild-type fish and two−/−fish.
The G130E mutation corresponds to the eighth amino acid in the fourth tetratricopeptide repeat domain in rapsyn (TPR) (Pont-ing and Phillips, 1996), a position known to be important for the folding of two α helices within each TPR domain (Fig. (Fig.55A) (Das et al., 1998). Our results suggested the possibility that the G130E mutation was critical for rapsyn function. To confirm that rapsyn was responsible for the mutant phenotype, wild-type (WT rapsyn-GFP) and mutant (G130E rapsyn-GFP) rapsyn cDNAs fused with GFP were transiently expressed in myotomal muscle oftwo−/− zebrafish embryos. The rapsyn-GFP transgene was expressed specifically in myotomal muscle because it was under control of the α-actin promoter (Higashijima et al., 1997). Plasmids were injected into 1–16 cell stage embryos, and the fluorescence distribution from the rapsyn-GFP protein was compared with Rh-α-Btx-labeled AChRs in 5-d-old fish. In transient assays such as these, the transgene is expressed in a stochastic manner such that there is mosaicism within the muscle, with some cells expressing the transgene and others not. The mosaicism was fortuitous, permitting comparison between effects of rapsyn-GFP expression in different muscle cells of the same fish.
When rapsyn-GFP cDNA was injected into fish, transgene expression was first observed at 2 d after the injection (Fig.(Fig.6).6). The level of transgene expression was highly variable among the muscle cells. Clusters of rapsyn were easier to identify in those muscle cells that expressed somewhat lower levels of rapsyn-GFP (Fig. (Fig.6).6). Tail muscles in these fish were subsequently colabeled with Rh-α-Btx to examine the distribution of the AChRs in muscle cells with and without exogenous rapsyn-GFP. In >100twitch once muscle cells that expressed exogenous wild-type rapsyn-GFP, we observed well defined large AChR clusters (Fig. (Fig.6).6). These clusters were similar in number and position to those present in labeled muscle cells from wild-type fish. In the sametwo−/− fish, we examined the AChR distribution in muscle cells that lacked GFP fluorescence, indicating the absence of expressed WT rapsyn-GFP (Fig. (Fig.6).6). In all cells examined, we observed no evidence of bona fide AChR clusters. Instead, the receptors were homogenously and diffusely distributed over the entire plasma membrane surface of the muscle. This distribution was indistinguishable from that observed intwo−/− fish without a transgene (Fig. (Fig.33).
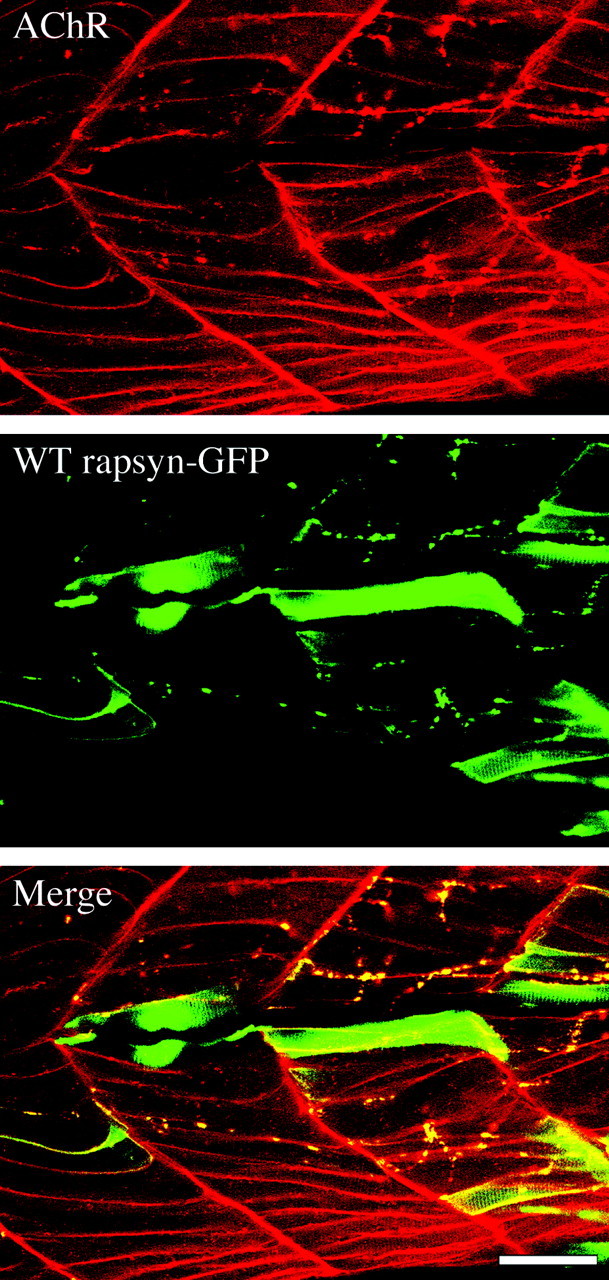
Transient expression of wild-type rapsyn intwo−/− fish. 1–16 cell stage fish embryos were microinjected with DNA constructs encoding wild-type rapsyn-GFP. Fish embryos were examined by confocal microscopy on day 5 for distribution of rapsyn and receptors. Confocal fluorescence images of red rhodamine-conjugated α-bungarotoxin (A), green rapsyn-GFP (B), and merged images (C). Note the presence of punctate clusters of both receptor and rapsyn that appear to colocalize in the merged image. Scale bar, 50 μm.
Distinctly different results were obtained when G130E rapsyn-GFP was injected into two−/− fish (Fig.(Fig.7).7). In all cells examined, the distribution of AChRs remained diffuse, even in muscle cells expressing the exogenous G130E rapsyn-GFP (Fig. (Fig.7).7). These results show definitively that the G130E mutation was causal to the twitch once phenotype. The distribution of the expressed G130E rapsyn-GFP in two−/− muscle also yielded insights into the probable distribution of the endogenous mutant rapsyn. The exogenously expressed G130E rapsyn-GFP was present at high levels throughout the plasma membrane of muscle, even entering into the T-tubules (Fig. (Fig.77C). High-resolution images revealed that, unlike the homogenous diffuse organization of the AChRs, mutant rapsyn formed some small microaggregates in the muscle membrane. These rapsyn microaggregates failed to cluster AChRs along the edges of the muscle cells (Fig. (Fig.77B,C), indicating that the principal action of the mutation was to block the rapsyn–receptor association. In other words, there is no difference of AChR distribution between areas of plasma membrane with and without rapsyn microclusters. However, the smaller size of the mutant rapsyn clusters, compared with wild-type clusters, suggested that the point mutation in the TPR domain also interfered with the ability to form large homomeric clusters.
Synaptic depression underlies the use-dependent fatigue in mutant fish
Is there a physiological effect of mutated rapsyn? The synaptic responses observed for twitch once muscle were greatly reduced in amplitude compared with those in wild-type fish (Fig. (Fig.2).2). However, a reduction in amplitude alone could not account for the defective swimming behavior in these mutant fish. Twitch once fish generate one or two power strokes before the onset of fatigue, suggesting that the initial muscle responses are stronger than subsequent responses. To test for the ability of muscle to follow high-frequency volley from the nervous system, motor neurons were stimulated with 50 Hz trains, close to the native firing rate for the motor swimming muscles (Fig. (Fig.8).8). Each 50 Hz train was composed of 10 pulses, and the trains were separated by 10 sec intervals. Whole-muscle patch-clamp recordings measured the associated EPCs.
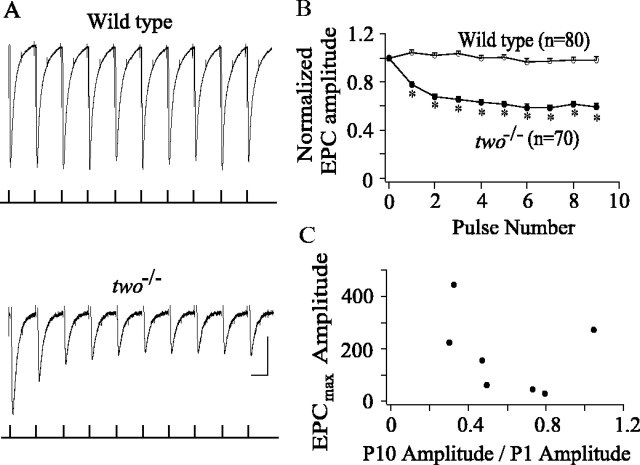
Endplate synaptic currents intwo−/− muscle exhibit marked depression in response to 50 Hz stimulation. A, Ten consecutive endplate currents (top traces) recorded in response to 50 Hz pulse train applied to the spinal cord. The timing of the stimuli relative to the whole-cell muscle recordings is shown in the bottom traces. The spinal cord was stimulated extracellularly using 300 μsec, 30 V pulses. The muscle cells were held at −50 mV to minimize contamination by sodium current. Note the depression of endplate current amplitude in twitch oncemuscle compared with wild type. Calibration: 20 msec, 100 (wild type) or 50 (twitch once) pA. B, The average ± SE ratio of each successive EPC to the amplitude of the first EPC recorded. The EPC ratios for wild-type fish are represented by filled circles (n = 80 from 8 cells), and two−/− fish are represented by open circles (n = 70 from 7 cells). Each average is based on 10 consecutive traces in a single muscle cell. *p < 0.01; Student'st test. C, The scatter plot relating maximum EPC amplitude recorded from eachtwo−/− fish versus the amount of depression observed. The depression is indicated by the ratio of the averaged 10th EPC amplitude to the averaged first EPC recorded in the train. Each average is based on 10 consecutive traces in a single muscle cell.
Recordings from eight wild-type fish indicated no significant changes in the average peak amplitudes of 10 successive EPCs (Fig.(Fig.88A,B). There was some variability observed within the train, but the average of several individual trains demonstrated that the successive EPCs were statistically invariant in amplitude. Recordings from seventwo−/− fish indicated that a majority of muscle cells were not able to follow 50 Hz stimulation without signs of synaptic depression (Fig.(Fig.88A,B). In six of seven recordings, decreases in average peak EPC amplitude occurred with successive pulses until an apparent plateau was reached by the end of the train (Fig.(Fig.88B). The tendency to depress was observed in all of the individual runs, but the average of the individual runs shows a smooth trend. The greatest depression in synaptic current amplitude occurred between pulses 1 and 2. However, significant depression continued throughout the successive EPCs in the train (Fig.(Fig.88B). In contrast, one of the seventwo−/− muscle recordings exhibited no statistically significant depression during the train. Thus, the ability to exhibit depression is not shared by all of the mutant synapses.
The question arose as to whether differences in the ability of wild-type and two−/− muscle to follow trains of 50 Hz stimuli resided in the differences in EPC amplitude. As a test of this idea, the amount of depression for each muscle recording was compared with the average EPC amplitude (Fig.(Fig.88C). In both wild-type andtwo−/− muscle, the average peak EPC amplitude was quite variable, likely attributable to the variability in synaptic receptor site densities. In particular, some of the mutant synaptic responses were comparable with the smaller-amplitude wild-type recordings (Fig. (Fig.2).2). Comparisons of peak EPC amplitudes to the amount of depression observed showed that there was no relationship between amplitude and the tendency to depress (Fig.(Fig.88C), supporting the idea that depression was not a simple consequence of lowered EPC amplitude.
DISCUSSION
Multiple lines of evidence indicated that defects in rapsyn were directly causal to the twitch once zebrafish phenotype. First, stable expression of a murine rapsyn-GFP intwo−/− fish greatly improved the ability to mount escape responses to tail taps, and the behaviorally rescued fish also exhibited wild-type synaptic clusters of ACh receptors. Second, comparison of wild-type and twitch oncerapsyn sequences revealed an amino acid difference in the alleletwoth26e. At this location, the predicted 130th amino acid of rapsyn was changed from a highly conserved glycine to a glutamate residue. Third, transient expression of wild-type zebrafish rapsyn-GFP intwo−/− fish resulted in mosaic animals wherein muscle cells expressing GFP fluorescence exhibited AChR clusters. In contrast, when G130E rapsyn-GFP was transiently expressed, no receptor clusters formed in muscle, despite the fact that the rapsyn was still able to self-cluster. Additional comparisons between wild-type and G130E rapsyn-GFP clusters indicated that the maximal cluster size was smaller for the latter. Most important, however, was the observation that G130E-GFP clusters occurred in the absence of AChR clusters, indicating that the major effect of the mutation was a block of the rapsyn–receptor interaction. One consequence of this blocked interaction was a widespread distribution of G130E rapsyn-GFP clusters. Thus, as demonstrated previously in AChR-null mutant fish, rapsyn, in the absence of interaction with receptor, was unable to effectively localize to postsynaptic membrane (Ono et al., 2001a).
Rapsyn is a modular protein that is constructed of three functionally distinct domains: tetratricopeptide repeat, coiled-coil, and RING-H2 domain (Scotland et al., 1993; Ramarao and Cohen, 1998; Ramarao et al., 2001). Mutagenesis and deletional analyses of rapsyn have provided tentative identification of the domains mediating self-aggregation and receptor association (Ramarao and Cohen, 1998; Ramarao et al., 2001). Disruption of the coiled-coil domain interferes with the ability of rapsyn to aggregate AChRs in heterologous cells but does not interfere with the self-clustering of rapsyn. The identification of the regions mediating self-clustering of rapsyn is less clear, but the bulk of evidence points to the involvement of the TPR domains (Ramarao and Cohen, 1998; Ramarao et al., 2001). Our observation that a mutation in a single TPR disrupts the size of clusters but not the ability to form clusters is consistent with the published role of the TPR domain in self-clustering. However, our finding that mutation in the TPR domain disrupted interaction with AChRs appears to be at odds with studies pointing to the coiled-coil domain as the site of interaction (Ramarao et al., 2001). It is possible that the mutation in the fourth TPR of rapsyn caused global changes in protein conformation, thereby altering the function of the coiled-coil region. The eighth residue has been shown, through structural analysis, to represent a key structural feature of TPR domains (Das et al., 1998), and mutations at this site effectively disrupt function in protein phosphatases containing TPR regions (Sikorski et al., 1993). Additional evidence for the importance of this key position is reflected in the fact that the hereditary human disorder chronic granulomatous disease results from a mutation in the TPR of the p67 protein (de Boer et al., 1994). Remarkably, in the p67 protein, just as is found for the twitch once mutation in rapsyn, glutamate is substituted for glycine in the eighth position.
Based on preliminary findings from our laboratory (Ono et al., 2001b), mutations in TPR regions of rapsyn were subsequently identified in congenital types of myasthenic syndrome (Ohno et al., 2002). Patients afflicted with this disorder show use-dependent muscle fatigue that is very similar in pattern to two−/−swimming. As with two−/− fish, myasthenic patients exhibit reduced subsynaptic receptor densities and elevated nonsynaptic receptor number (Fambrough et al., 1973). The myasthenic phenotype in human rapsyn patients was proposed to result from either lowered muscle cell resistance or reduced Na+ channel activation attributable to deformation of postsynaptic folds (Ohno et al., 2002). Our physiological analyses of synaptic function intwo−/− fish offer an alternative explanation. In mutant fish, the weakened muscle response is a consequence of the combined effects of lowered receptor density and short-term depression in synaptic response amplitude. Repetitive 50 Hz stimulation of two−/− motor neurons led to significant cumulative depression of endplate currents. Because muscle cells are weakly excitable at early stages in development (Buss and Drapeau, 2000), the force of contraction is proportional to the magnitude of the synaptic potential. Thus, the steep decline in amplitude that occurred within the first three EPCs in mutant fish may have resulted in a peak response that was too small to result in movement. In contrast, wild-type synaptic responses showing no depression led to normal nonfatiguable responses.
How might a mutation in rapsyn augment synaptic depression? Synaptic depression at neuromuscular junctions is common at newly formed synapses in culture in which both presynaptic and postsynaptic differentiation are incomplete (Dan et al., 1995). However, depression at adult neuromuscular junctions is only observed during intense and prolonged stimulation (Betz, 1970). The bulk of studies on neuron–neuron synapses point to presynaptic mechanisms as causal to synaptic depression (Thomson, 2000). However, recent studies have indicated that postsynaptic receptor desensitization can contribute to depression at certain of those synapses (Trussell et al., 1993; Jones and Westbrook, 1996). The ability to assign depression at the neuromuscular junction to either presynaptic or postsynaptic mechanisms has proven very difficult (Sandrock et al., 1997). In the case oftwitch once fish, the most likely mechanism underlying the synaptic depression appears to be postsynaptic receptor desensitization. Studies using fast application of ACh on embryonic-type amphibian nicotinic receptors predict that frequencies corresponding to those in swimming fish will result in pronounced desensitization (Paradiso and Brehm, 1998).
A possible link between receptor desensitization and rapsyn is offered by the finding that at least one of the principal sites of receptor phosphorylation requires association with rapsyn for the modification (Wallace et al., 1991; Gillespie et al., 1996; Apel et al., 1997;Mittaud et al., 2001). Furthermore, tyrosine kinase phosphorylation, as well as phosphorylation by protein kinase A, affects the kinetics of desensitization (Hopfield et al., 1988; Paradiso and Brehm, 1998). Thus, receptors in twitch once may be prone to desensitization attributable to the absence of functional interactions with rapsyn, and this desensitization may underlie the synaptic depression. Until the mechanisms underlying depression in twitch once fish have been identified, we cannot rule out indirect effects on presynaptic release or alterations in synaptic morphology. We can rule out the possibility that the depression is a simple consequence of lowered receptor density based on the lack of correlation between tendency to depress and postsynaptic response amplitude (Fig. (Fig.88C). Although the mechanisms through which rapsyn affects synaptic function are not known, it is clear that rapsyn directly or indirectly plays a role in synaptic function.
Our studies on twitch once zebrafish may also offer a new perspective on nonhereditary forms of myasthenia gravis. Eighty percent of patients afflicted with this disease test positive for anti-AChR antibodies (Lindstrom et al., 1976). However, important new insights have been gained recently from a study of patients that failed to test positive for receptor antibodies. A majority of these patients tested positive for antibodies against either muscle-specific kinase (MuSK) or rapsyn (Agius et al., 1998; Hoch et al., 2001). MuSK is required for the proper anchoring of the rapsyn-AChR complex to the subsynaptic site, as well as for the rapsyn-dependent phosphorylation of AChR (DeChiara et al., 1996; Apel et al., 1997). A direct involvement of rapsyn antibodies in the disease process was suggested by the onset of myasthenia gravis-like symptoms in mice immunized with rapsyn (Agius et al., 1998). Thus, based on our results from twitch oncemutant fish, the MuSK-rapsyn-AChR pathway may be involved in receptor desensitization and associated synaptic depression at the neuromuscular junction. Interference with any one of these components of receptor localization may result in the use-dependent fatigue in movement associated with this disease.
Footnotes
This study was supported by National Institutes of Health Grant NS-8205 (P.B.). G.M. is an investigator of the Howard Hughes Medical Institute. We thank Dr. Frohnhoefer (Max-Planck-Institute, Tuebingen, Germany) for providing twitch once mutant zebrafish (allele twoth26e). Dr. Joseph Fetcho provided technical advice and insights throughout the project. Joan Speh offered expert technical assistance with confocal microscopy and Shelagh Palma provided expert care of the fish. We thank Dr. Howard Sirotkin for critical reading of this manuscript.
Correspondence should be addressed to Paul Brehm, Department of Neurobiology and Behavior, State University of New York at Stony Brook, Stony Brook, NY 11794. E-mail: ude.bsynus.cc.seton@mherbp.
REFERENCES
Articles from The Journal of Neuroscience are provided here courtesy of Society for Neuroscience
Full text links
Read article at publisher's site: https://doi.org/20026669
Read article for free, from open access legal sources, via Unpaywall:
http://www.jneurosci.org/content/jneuro/22/15/6491.full.pdf
Free to read at www.jneurosci.org
http://www.jneurosci.org/cgi/content/abstract/22/15/6491
Free after 6 months at www.jneurosci.org
http://www.jneurosci.org/cgi/content/full/22/15/6491
Free after 6 months at www.jneurosci.org
http://www.jneurosci.org/cgi/reprint/22/15/6491.pdf
Citations & impact
Impact metrics
Citations of article over time
Alternative metrics

Discover the attention surrounding your research
https://www.altmetric.com/details/102518641
Smart citations by scite.ai
Explore citation contexts and check if this article has been
supported or disputed.
https://scite.ai/reports/20026669
Article citations
Neuropeptidergic regulation of neuromuscular signaling in larval zebrafish alters swimming behavior and synaptic transmission.
iScience, 27(9):110687, 06 Aug 2024
Cited by: 0 articles | PMID: 39252958 | PMCID: PMC11381845
Context-dependent hyperactivity in syngap1a and syngap1b zebrafish models of SYNGAP1-related disorder.
Front Mol Neurosci, 17:1401746, 10 Jul 2024
Cited by: 0 articles | PMID: 39050824 | PMCID: PMC11266194
Single-cell RNAseq analysis of spinal locomotor circuitry in larval zebrafish.
Elife, 12:RP89338, 17 Nov 2023
Cited by: 3 articles | PMID: 37975797 | PMCID: PMC10656102
Digital Video Acquisition and Optimization Techniques for Effective Animal Tracking in Behavioral Ecotoxicology.
Environ Toxicol Chem, 41(10):2342-2352, 06 Sep 2022
Cited by: 0 articles | PMID: 35848752 | PMCID: PMC9826254
Agrin/Lrp4 signal constrains MuSK-dependent neuromuscular synapse development in appendicular muscle.
Development, 148(21):dev199790, 29 Oct 2021
Cited by: 5 articles | PMID: 34714331 | PMCID: PMC8602948
Go to all (55) article citations
Data
Data behind the article
This data has been text mined from the article, or deposited into data resources.
BioStudies: supplemental material and supporting data
Nucleotide Sequences
- (1 citation) ENA - AB070208
Similar Articles
To arrive at the top five similar articles we use a word-weighted algorithm to compare words from the Title and Abstract of each citation.
Paralytic zebrafish lacking acetylcholine receptors fail to localize rapsyn clusters to the synapse.
J Neurosci, 21(15):5439-5448, 01 Aug 2001
Cited by: 84 articles | PMID: 11466415 | PMCID: PMC6762670
Fatigue in Rapsyn-Deficient Zebrafish Reflects Defective Transmitter Release.
J Neurosci, 36(42):10870-10882, 01 Oct 2016
Cited by: 6 articles | PMID: 27798141 | PMCID: PMC5083014
The dynamics of the rapsyn scaffolding protein at individual acetylcholine receptor clusters.
J Biol Chem, 282(13):9932-9940, 05 Feb 2007
Cited by: 17 articles | PMID: 17283077
Recombinant neuromuscular synapses.
Bioessays, 14(10):671-679, 01 Oct 1992
Cited by: 9 articles | PMID: 1365879
Review