Abstract
Free full text

Neutrophils mediate immune modulation of dendritic cells through glycosylation-dependent interactions between Mac-1 and DC-SIGN
Abstract
Neutrophils are key players of the innate immune system that provide a first line of defense against invading pathogens. However, it is unknown whether neutrophils can interact with dendritic cells (DCs) to modulate adaptive immune responses. We demonstrate that neutrophils strongly cluster with immature DCs and that activated, not resting, neutrophils induce maturation of DCs that enables these DCs to trigger strong T cell proliferation and T helper type 1 polarization of T cells. This neutrophil–DC interaction is driven by the binding of the DC-specific, C-type lectin DC-SIGN to the β2-integrin Mac-1. Strikingly, DC-SIGN only interacts with Mac-1 from neutrophils, but not from other leukocytes, mainly because of specific Lewisx carbohydrates that are present on the αM chain of Mac-1 from neutrophils. Furthermore, we show that besides the formation of cellular contact, the tumor necrosis factor-α produced by activated neutrophils is essential for inducing DC maturation. Our data demonstrate that DC-SIGN and Mac-1 define a molecular pathway to establish cellular adhesion between DCs and neutrophils, thereby providing a novel cellular link between innate and adaptive immunity.
DCs are antigen-presenting cells that play an essential role in bridging innate and adaptive immunity. Immature DCs are situated underneath epithelia throughout peripheral tissues, where they are ideally located to acquire antigens of invading pathogens. Pathogenic structures such as lipopolysaccharide, lipoteichoic acid, and peptidoglycans, but also endogenous signals like CD40–CD40L ligation by T cells (1) and TNF-α production by NK cells (2), trigger DC maturation. DC maturation is an intricate process that includes a switch in the chemokine receptor profile, the translocation of MHC molecules from the cytosol to the cell membrane, and the up-regulation of costimulatory molecules and cytokines. This allows mature DCs to leave the periphery and migrate to the lymph nodes, and endows them with the capacity to present antigen in the context of MHC molecules in order to initiate T cell responses within the lymph nodes (3).
DC-SIGN is a C-type lectin that is expressed in vitro on monocyte-derived DCs and in situ on DC subsets in the skin, mucosal tissues, tonsils, lymph nodes, and spleen (4, 5). DC-SIGN plays an important role in many aspects of DC function. DC-SIGN has specificity for high-mannose moieties (6), and it functions as an adhesion receptor that establishes cellular interactions with endothelial cells through ICAM-2 (7) and with T cells through ICAM-3 (4), probably by recognizing high-mannose moieties on these counterstructures. In addition, DC-SIGN has been resolved as the receptor on DCs for HIV-1 that facilitates in trans infection of CD4 T cells (5). DC-SIGN not only binds HIV-1, but also serves as a pathogen recognition receptor with broad specificity that recognizes and may contribute to the pathophysiology of the hepatitis C virus (8), Mycobacterium tuberculosis (9), Helicobacter pylori, Schistosoma mansoni (10, 11), and other pathogens (12–14).
We have resolved the carbohydrate ligand of DC-SIGN on H. pylori and S. mansoni (10, 11). Strikingly, nonsialylated Lewisx was identified as the high-affinity carbohydrate ligand of DC-SIGN rather than high-mannose structures (6, 10). Nonsialylated Lewisx is a carbohydrate structure that is also found on human tissue, in particular on neutrophilic granulocytes (15). Neutrophils are key players of the innate immune system and provide a first line of defense against invading pathogenic bacteria (16). Recently, it has become clear that neutrophils also have a regulatory role and are able to signal to other players of the immune system. Activated neutrophils produce and release chemokines such as IL-8 and GRO-α, which enable them to attract additional neutrophils (17, 18). Through the release of the chemokines MIP-1α, MIP-3α, and MIP-3β, neutrophils also actively recruit other immune cells like T cells, monocytes, macrophages, and DCs (19, 20). Antimicrobial peptides produced by neutrophils such as α-defensins have chemotactic functions and attract T cells and immature DCs (21). This endows neutrophils with the potential to orchestrate ongoing immune responses at the site of infection.
Neutrophils may also indirectly modulate adaptive immune responses in distant lymph nodes through interactions with immature DCs. This is supported by recent data showing that TNF-α derived from PMN induces maturation and cytokine production in murine DCs (22). Although to date no cellular interactions between DCs and PMN have been described, we hypothesized that, based on the Lewisx specificity of DC-SIGN, DCs would be able to engage PMN, and that this would result in cross talk between DCs and PMN. Indeed, we were able to demonstrate both in vitro and in vivo that DCs and PMN associate, and that DC-SIGN mediates this cellular interaction. We identified Mac-1 as the ligand of DC-SIGN on PMN, and show that the interaction between DC-SIGN and Mac-1 depends on PMN-specific glycosylation of Mac-1. Moreover, we found that activated PMN induce maturation of DCs, which enables these DCs to trigger strong Th type 1 cell responses. This indicates that neutrophils contribute to adequate adaptive immune responses through interactions with DCs.
Results
Cellular interactions between DCs and PMN
Neutrophils are key effector cells of innate immune responses. However, it has recently become clear that neutrophils may also play a role in the polarization of adaptive T cell responses (23–25). Because DCs, in contrast to neutrophils, are able to migrate to the lymph nodes and can efficiently present antigens to T cells, we considered the possibility that immune modulation by neutrophils is indirect and requires prior interactions with DCs. To examine whether cellular interactions occur between DCs and PMN, we labeled DCs with a red fluorescent dye, hydroethidin, and determined clustering with CFSE-labeled PMN (green). Monocyte-derived immature DCs strongly interacted with PMN after 30 min of coincubation (Fig. 1 A). Cell–cell clustering was followed by a FACS analysis, and was rapid and sustained (Fig. 1 B). We investigated whether DC-SIGN was involved in DC–PMN interactions. Indeed, blocking anti–DC-SIGN antibodies strongly diminished adhesion, demonstrating that DC-SIGN mediates cellular interactions between DCs and PMN (Fig. 1, A and E). Mature DCs that expressed reduced levels of DC-SIGN also interacted with PMN, but to a lesser extent (Fig. 1, A–C). K562 transfectants expressing high levels of DC-SIGN, but not mock-transfected K562 cells, strongly bound to PMN and the kinetics of cluster formation were similar to those of immature DCs and PMN (Fig. 1, A and D). The addition of blocking anti–DC-SIGN antibodies diminished cluster formations between PMN and K562-DC-SIGN (Fig. 1, A and E), supporting a role for DC-SIGN in cellular adhesion to PMN. To our knowledge, this is the first evidence showing that DCs interact with PMN; moreover, we show that DC-SIGN is the main DC receptor establishing this cell–cell interaction.
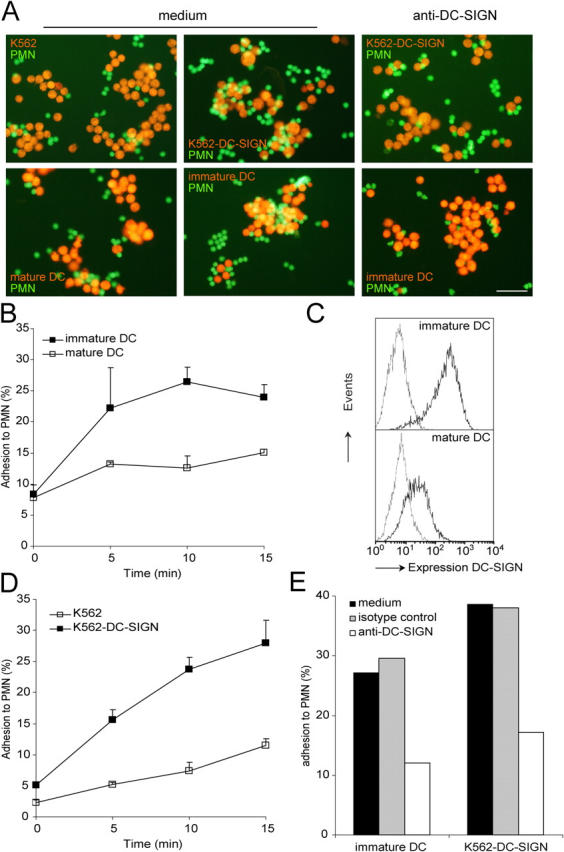
DC-SIGN mediates clustering of DCs and PMN. (A) CFSE-labeled PMN (green) were incubated with hydroethidine-labeled K562, K562-DC-SIGN, immature DCs, or mature DCs (red) for 30 min at 37°C. Anti–DC-SIGN antibodies (AZN-D1 and AZN-D2; 20 μg/ml) were used to block DC-SIGN dependent adhesion. Cell–cell clustering was visualized using fluorescent microscopy and representative pictures were taken. Bar, 50 μm. Three independent experiments with similar results were performed. (B) Using FACS analysis cell–cell clustering was followed in time by scoring the percentage of immature or mature DCs that have bound PMN. (C) The expression of DC-SIGN was analyzed on immature and mature DCs by flow cytometry. (D) The percentage of K562 or K562-DC-SIGN that have bound PMN was measured in time by FACS analysis. (E) DC-SIGN–dependent adhesion of PMN to immature DCs and K562-DC-SIGN was determined with blocking anti–DC-SIGN antibodies at 10 min of cell–cell clustering. One out of three independent experiments with similar results is shown.
Mac-1 is the ligand for DC-SIGN on PMN
ICAM-2 and -3 are cellular ligands of DC-SIGN (4, 7). Because neutrophils express high levels of ICAM-3, but not of ICAM-2, we examined whether ICAM-3 is the ligand of DC-SIGN on neutrophils. Therefore, we immunoprecipitated ICAM-3 from PMN and determined DC-SIGN binding by blotting it with a chimeric protein consisting of DC-SIGN and the human IgG1-Fc domain (DC-SIGN-Fc; reference 26). Strikingly, in contrast to ICAM-3 that was obtained from 293T-ICAM-3 transfectants, ICAM-3 that was derived from PMN was poorly bound by DC-SIGN (Fig. 2 C). This indicates that ICAM-3 on PMN does not contain the proper carbohydrate moieties for binding to DC-SIGN, and that DC-SIGN recognizes a novel ligand on PMN. To identify this novel DC-SIGN ligand on PMN, we performed an immunoblot analysis on the entire PMN lysate with DC-SIGN-Fc. A 160-kD major ligand specifically interacted with DC-SIGN-Fc, but not with control-Fc (Fig. 2 A). DC-SIGN-Fc did not interact with the lysate from an irrelevant cell line (Fig. 2 A). To investigate whether the DC-SIGN ligand is expressed on the cell surface, we performed immunoprecipitations with DC-SIGN-Fc on a lysate of surface-biotinylated PMN. Immunoblotting for the biotin-labeled membrane proteins showed that, indeed, DC-SIGN recognized a 160-kD membrane protein (Fig. 2 B). Notably, a second 100-kD membrane protein that did not appear on the DC-SIGN immunoblot (Fig. 2 A) was immunoprecipitated using DC-SIGN-Fc (Fig. 2 B). The immunoprecipitated proteins are identical to those of the β2-integrin Mac-1 that consists of a 160-kD αM chain (CD11b) and a 100-kD β2 chain (CD18) (Fig. 2 B). To determine whether Mac-1 is the ligand of DC-SIGN on PMN, we analyzed the binding of DC-SIGN-Fc to Mac-1, immunoprecipitated from PMN using anti–Mac-1 antibodies. We found that the αM chain of Mac-1 binds much stronger to DC-SIGN than the β2 chain (Fig. 2 C). Thus, the 100-kD membrane protein is not a strong DC-SIGN ligand, but represents the coimmunoprecipitated β2 chain of Mac-1 that noncovalently associates with the αM chain of Mac-1. Immunoprecipitation of DC-SIGN ligands using a recombinant DC-SIGN and immunoblot analysis with antibodies directed against the αM chain of Mac-1 confirmed that the αM chain of Mac-1 is the main DC-SIGN ligand on PMN (Fig. 2 D).
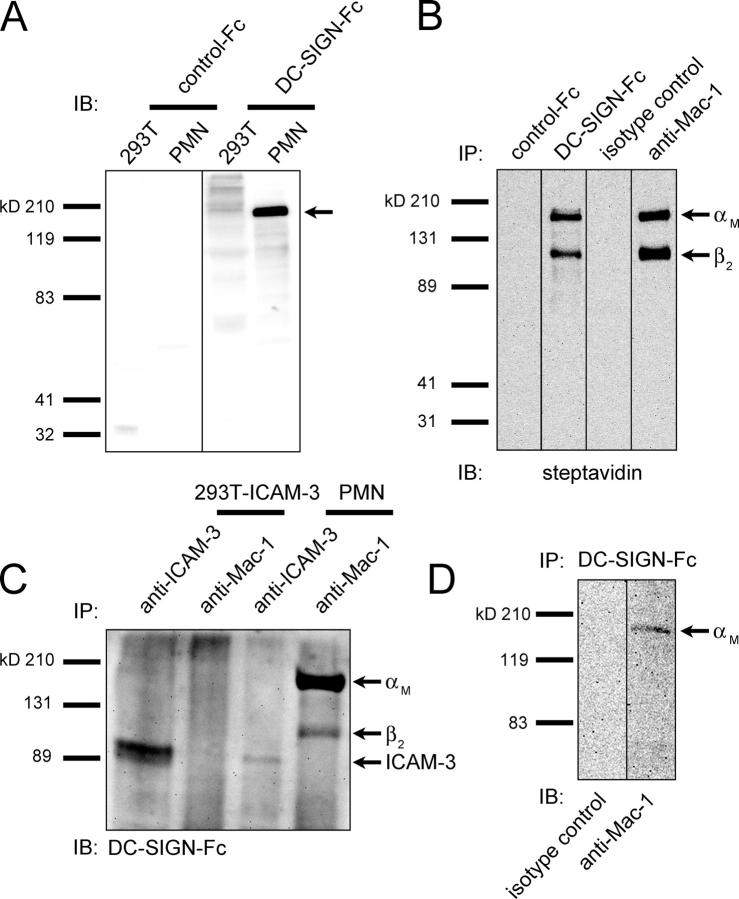
Identification of PMN Mac-1 as a novel ligand of DC-SIGN. (A) Whole PMN and 293T lysate were analyzed on immunoblot (IB) with control-Fc and DC-SIGN-Fc. The arrow indicates the main DC-SIGN ligand of ~160 kD. (B) Surface-biotinylated PMN were lysed and immunoprecipitated (IP) with control-Fc, DC-SIGN-Fc, mouse IgG1 isotype control antibodies, and anti–Mac-1 (αM chain) antibodies. Immunoprecipitates were analyzed on immunoblot with streptavidin. Arrows indicate the αM and β2 chains of Mac-1. (C) 293T-ICAM-3 and PMN were immunoprecipitated with anti–Mac-1 and anti–ICAM-3 antibodies and immunoblotted with DC-SIGN-Fc. Arrows indicate ICAM-3 and the αM and β2 chains of Mac-1. (D) DC-SIGN-Fc immunoprecipitates of PMN lysate were immunoblotted with isotype control and anti–Mac-1 (αM chain) antibodies. Arrow indicates the αM chain of Mac-1. Results are representative of three independent experiments.
Cell-specific glycosylation regulates DC-SIGN–Mac-1 interactions
Mac-1 is broadly expressed on cells of the myeloid lineage and is present on neutrophils, eosinophils, monocytes, macrophages, and DCs. As glycosylation is cell type specific, the cellular origin of Mac-1 may be of importance in binding to DC-SIGN. We investigated the interaction of DC-SIGN with PMN, monocytes, and immature DCs using DC-SIGN-Fc. Although monocytes, immature DCs, and PMN all express Mac-1, DC-SIGN only binds PMN (Fig. 3 A). The interaction was DC-SIGN specific, because blocking antibodies against DC-SIGN inhibited binding (Fig. 3 A). As in human blood, 95% of PMN are neutrophils and 5% are eosinophils; we thus investigated the interaction of DC-SIGN in both PMN populations. Costaining for CD16, which is expressed on neutrophils but not on eosinophils, demonstrated the binding of DC-SIGN to both populations that were sensitive to blocking anti–DC-SIGN antibodies. However, the CD16+ neutrophils bound much stronger to DC-SIGN than the CD16− eosinophils (Fig. 3 B).
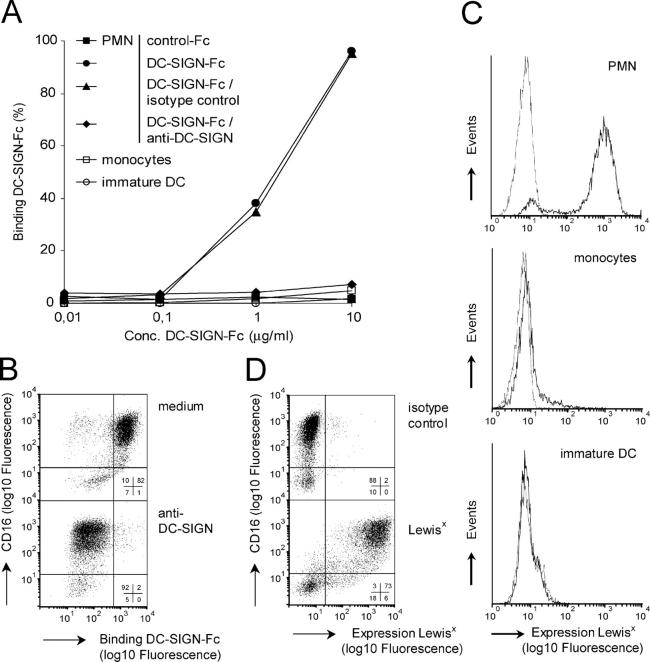
DC-SIGN specifically binds Lewisx-expressing PMN. (A) The binding of control-Fc and DC-SIGN-Fc to PMN, monocytes, and immature DCs was analyzed by flow cytometry. Binding of DC-SIGN-Fc to PMN is sensitive to blocking anti–DC-SIGN antibodies (AZN-D1; 50 μg/ml). (B) DC-SIGN-Fc preferably binds CD16+ neutrophils and not CD16− eosinophils (top). Blocking anti–DC-SIGN antibodies (AZN-D1; 50 μg/ml) inhibit binding of DC-SIGN-Fc (bottom). Insets represent the percentage of cells within a quadrant. (C) Isotype (thin line) and Lewisx (thick line) expressions were measured on PMN, monocytes, and immature DCs. (D) Isotype (top) and Lewisx expression (bottom) were examined on CD16+ neutrophils and CD16− eosinophils. Insets represent the percentage of cells within a quadrant. One out of three independent experiments with similar results is shown.
Recently, we have identified nonsialylated Lewisx (CD15) as an alternative high-affinity carbohydrate ligand of DC-SIGN (10) in lieu of high mannose (6). Whereas nonsialylated Lewisx is absent from immature DCs and monocytes, it is present at high levels on PMN (Fig. 3 C). The labeling of PMN for CD16 and Lewisx shows that CD16+ neutrophils express higher levels of Lewisx than CD16− eosinophils (Fig. 3 D). Thus, although Mac-1 is also expressed on other cells, DC-SIGN specifically recognized PMN. The binding of DC-SIGN corresponded to the expression of Lewisx, indicating that cell-specific glycosylation regulates interactions with DC-SIGN.
DC-SIGN recognizes Lewisx moieties on PMN-derived Mac-1
Next to Mac-1, PMN express other integrins that share the β2 chain, but have a distinct α chain, such as LFA-1 (αLβ2) and p150,95 (αXβ2). We developed an ELISA-based binding assay to analyze the binding of DC-SIGN to these β2-integrins. Using specific antibodies for Mac-1, LFA-1, p150,95, or all β2-integrins combined, we captured these proteins from PMN under nondenaturing conditions (Fig. 4 A) and determined their binding to recombinant DC-SIGN. DC-SIGN specifically interacted with Mac-1, but not with the other β2-integrins (Fig. 4 C). We analyzed the expression of Lewisx on these β2-integrins and found that neither LFA-1 nor p150,95, in contrast to Mac-1, expressed Lewisx (Fig. 4 D), as has been previously reported (27, 28). This indicates that the expression of Lewisx determines the binding of DC-SIGN. In this assay, ICAM-3 that was derived from PMN, in contrast to ICAM-3 that was derived from 293T-ICAM-3 transfectants, did not bind to DC-SIGN, confirming that ICAM-3 from PMN is not a DC-SIGN ligand (Fig. 4, A–C).
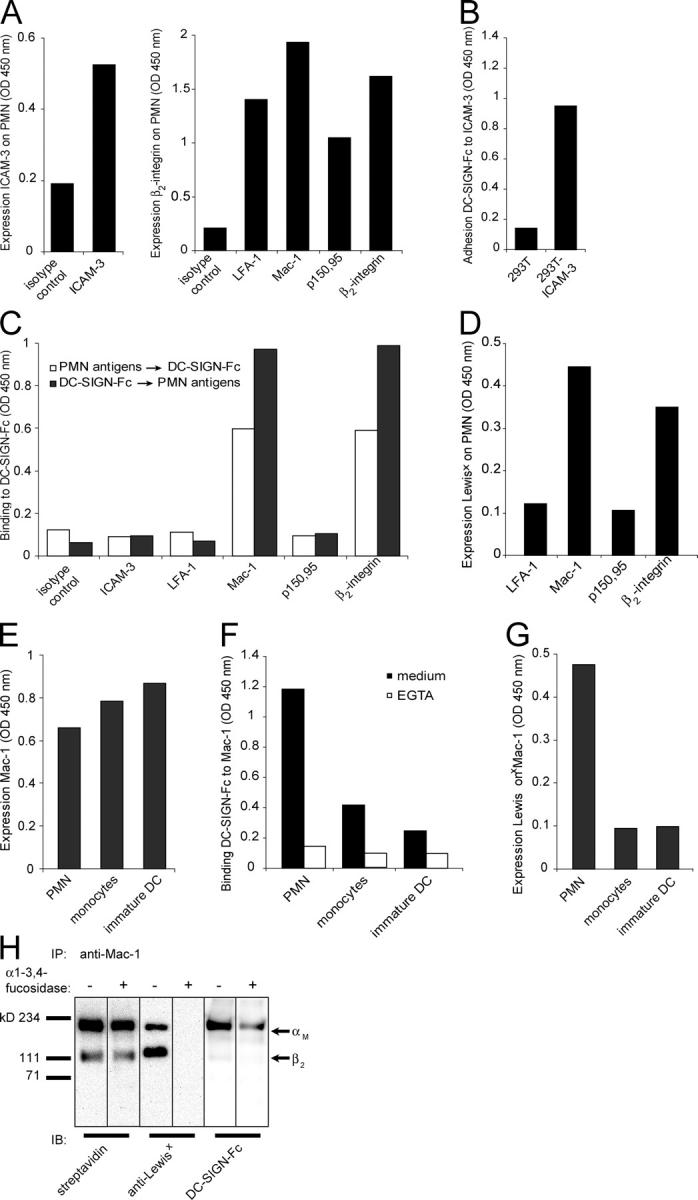
DC-SIGN binds Mac-1 expressed on PMN through Lewisx carbohydrates. (A) Sandwich ELISA on ICAM-3, LFA-1, Mac-1, p150,95, and β2-integrins from PMN. (B) The binding of DC-SIGN-Fc to ICAM-3 that was captured from mock and ICAM-3–transfected 293T cells was measured in an ELISA-based binding assay. (C) DC-SIGN-Fc binding to ICAM-3, LFA-1, Mac-1, p150,95, and β2-integrins that were captured from PMN (white bars) and the reverse reaction—the capture of DC-SIGN ligands using DC-SIGN-Fc and the detection of these ligands using antibodies (black bars)—were measured in an ELISA-based binding assay. (D) Using ELISA, the presence of Lewisx on LFA-1, Mac-1, p150,95, and β2-integrins derived from PMN was determined. (E) The quantity of Mac-1 of PMN, monocytes, and immature DCs was determined using a sandwich ELISA for Mac-1 (capture, αM chain; detection, β2 chain). (F) DC-SIGN-Fc binding to Mac-1 captured from lysate of PMN, monocytes, and immature DCs was analyzed in an ELISA-based binding assay. Ca2+ specificity of DC-SIGN binding was determined using the Ca2+ chelator EGTA (10 mM). (G) The presence of Lewisx on Mac-1 that was derived from PMN, monocytes, and immature DCs was measured by ELISA (capture, Mac-1; detection, Lewisx). (H) Lysates of surface-biotinylated PMN were immunoprecipitated with anti–Mac-1 antibodies. The immunoprecipitates were incubated overnight with or without α1-3,4-fucosidase (Xanthomonas; Calbiochem) in sodium phosphate buffer (50 mM, pH 5) at 37°C. Immunoprecipitates were then immunoblotted with streptavidin, anti-Lewisx antibodies, and DC-SIGN-Fc. Arrows indicate the αM and β2 chains of Mac-1. Results are representative of three independent experiments.
To molecularly define the cellular specificity of DC-SIGN for PMN-derived Mac-1, we captured Mac-1 from PMN, monocytes, and immature DCs (Fig. 4 E), and examined DC-SIGN binding and Lewisx expression. DC-SIGN bound much stronger to Mac-1 from PMN than to similar levels of Mac-1 from either monocytes or immature DCs (Fig. 4 F). The presence of Lewisx on Mac-1 from PMN, in contrast to Mac-1 that was derived from either monocytes or immature DCs, indicates that the glycosylation of Mac-1 is cell specifically regulated and determines the high affinity of DC-SIGN for Mac-1 present on PMN (Fig. 4 G). Thus, cell-specific glycosylation of Mac-1 in PMN results in the addition of Lewisx moieties, and we have identified this Lewisx-expressing glycoform of Mac-1 as a novel cellular ligand of DC-SIGN.
Because Mac-1 on PMN contains Lewisx (27, 28), a high-affinity carbohydrate ligand of DC-SIGN (10), we examined whether the Lewisx moieties mediated binding of DC-SIGN to PMN-expressed Mac-1. Therefore, we immunoprecipitated Mac-1 from surface-biotinylated PMN using anti–Mac-1 antibodies, and incubated this with α1-3,4-fucosidase, which is an enzyme that specifically targets Lewisx and removes the fucose moiety essential for binding to DC-SIGN (29). This enzyme did not disrupt Mac-1 integrity, but completely removed the Lewisx epitope from Mac-1, because Mac-1 treated with α1-3,4-fucosidase did not react with anti-Lewisx antibodies (Fig. 4 H). The α1-3,4-fucosidase treatment substantially decreased the binding of DC-SIGN to Mac-1, showing that Lewisx on Mac-1 is important for interactions with DC-SIGN (Fig. 4 H). Interactions of DC-SIGN with alternative carbohydrate ligands on Mac-1 may explain the residual DC-SIGN binding after the enzymatic removal of Lewisx, because treatment of Mac-1 with PNGase F, which removes all N-linked glycosylation, completely abrogated DC-SIGN binding (unpublished data). Strikingly, although both the αM, as well as the β2, chain of Mac-1 express Lewisx, DC-SIGN only bound the αM chain of Mac-1. This indicates that the structural conformation of the Lewisx glycosylation site is important for binding to DC-SIGN.
Cellular DC-SIGN binds to PMN-derived Mac-1
Next, we investigated the interaction of cellular DC-SIGN with Mac-1. We measured the adhesion of fluorescent beads coated with PMN-derived Mac-1 to DC-SIGN–expressing cells. K562 transfectants expressing high levels of DC-SIGN strongly bound to PMN-derived Mac-1, in contrast to mock-transfected K562 cells (Fig. 5 A). The C-type lectin domain of DC-SIGN mediated adhesion, because anti–DC-SIGN antibodies that targeted this domain inhibited the binding of PMN-derived Mac-1 to K562-DC-SIGN (Fig. 5 A). In contrast, beads coated with PMN-derived LFA-1 or p150,95 did not interact with K562-DC-SIGN (Fig. 5 A), and beads coated with monocyte- or DC-derived Mac-1 interacted poorly with K562-DC-SIGN (Fig. 5 B), indicating that cellular DC-SIGN shows specificity similar to recombinant DC-SIGN. Multivalent binding of Mac-1–coated beads to K562-DC-SIGN that may support low-affinity interactions more easily could explain the low level of binding of monocyte- and DC-derived Mac-1 to DC-SIGN. To investigate the involvement of the C-type lectin domain in Mac-1 binding in more detail, we used DC-SIGN mutants in which amino acids essential to ligand binding and the positioning of the Ca2+ ions have been mutated. Changing the amino acids Glu347 into Gln, or Asn349 and Asn365 into Asp, prevented the formation of hydrogen bonds between DC-SIGN and its ligands (26) and completely disrupted adhesion to PMN-derived Mac-1 (Fig. 5 C). The C-type lectin domain of DC-SIGN contains two pockets for calcium ions that are crucial for ligand binding (6, 26). Substitution of the amino acid Asp366 with Ala resulted in the loss of Ca2+ at site 2, the primary ligand-binding site, and completely abrogated adhesion to Mac-1 derived from PMN (Fig. 5 C). Changing the amino acids Asp320, Glu324, or Asp355 that form Ca2+ site 1 into Ala resulted in a complete loss of adhesion to PMN Mac-1 (Fig. 5 C). This shows that the binding site of DC-SIGN for PMN-derived Mac-1 is located within the C-type lectin domain.
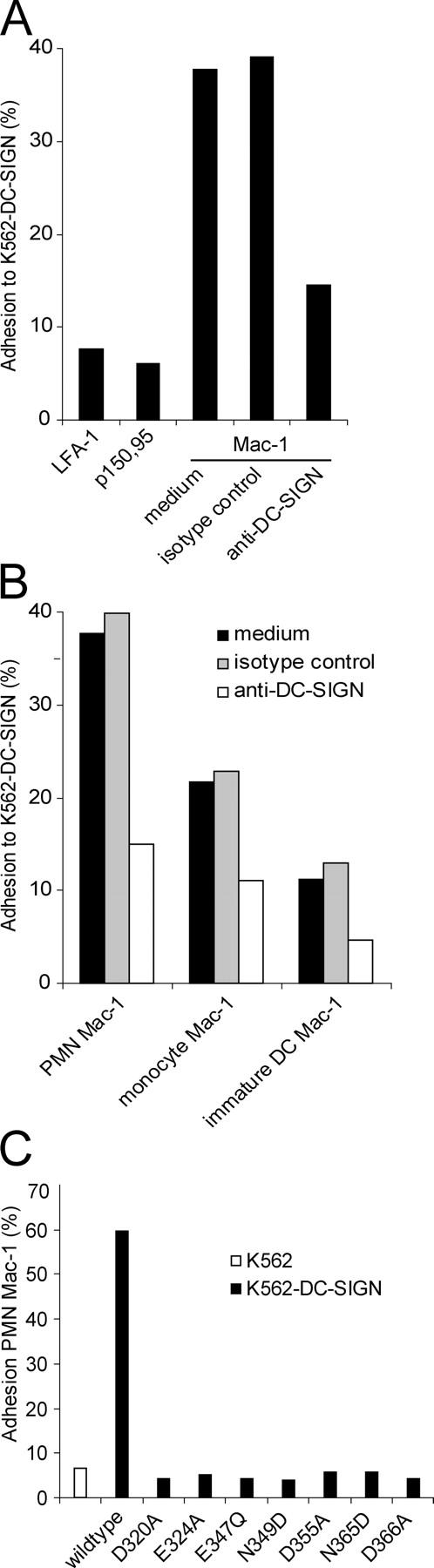
The C-type lectin domain of DC-SIGN binds PMN Mac-1. (A) The binding of fluorescent beads coated with PMN-derived LFA-1, p150,95, and Mac-1 was measured to K562-DC-SIGN. Adhesion of PMN-derived Mac-1 beads was sensitive to blocking anti–DC-SIGN antibodies (AZN-D1; 20 μg/ml). (B) The adhesion of Mac-1 derived from PMN, monocytes, and immature DCs to K562-DC-SIGN was analyzed in a fluorescent bead adhesion assay. Anti–DC-SIGN antibodies were used to determine the specificity of DC-SIGN binding. (C) The adhesion of fluorescent beads coated with PMN Mac-1 to K562 cells and K562 transfectants expressing wildtype or mutant DC-SIGN, in which amino acids within the C-type lectin domain essential for ligand binding or the positioning of Ca2+ ions are mutated, is shown. One out of three independent experiments is shown.
Interactions between DCs and PMN at inflammatory sites
Little is known about the occurrence of cellular contacts between DCs and PMN in situ. However, DCs and PMN are present at the sites of infection during early inflammation. To examine whether DCs and PMN associate, we investigated colonic mucosa from patients with Crohn's disease, a chronic inflammatory bowel disease, and stained for DC-SIGN and the PMN markers CEACAM1 (CD66a) and Lewisx. In colonic mucosa, DC-SIGN was present on a distinct population of CD83− DCs that increased in number upon chronic inflammation during Crohn's disease (30). CEACAM1 and Lewisx were expressed on an identical PMN population within the colonic mucosa of patients with Crohn's disease (Fig. 6 A) that was not present in noninflammatory colonic mucosa (unpublished data). PMN within inflammatory colonic mucosa that were identified by Lewisx staining also expressed the DC-SIGN ligand Mac-1 (Fig. 6 B). We found that DC-SIGN+ DCs and PMN expressing CEACAM1 or Lewisx were both scattered throughout the mucosa. We also observed frequent contacts between DCs and PMN, suggesting that they interact at inflammatory sites in colonic mucosa of patients with Crohn's disease (Fig. 6, C and D). This supports the hypothesis that interactions between DCs and PMN occur in vivo during inflammation.
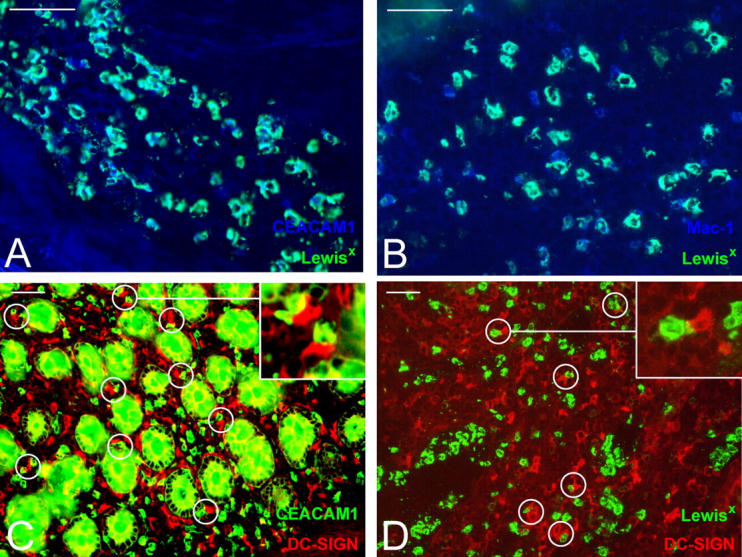
In vivo localization of PMN and DCs in colonic mucosa of patients with Crohn's disease. Inflammatory intestinal tissue sections of patients with Crohn's disease were stained for the PMN markers (A) Lewisx (green) and CEACAM1 (blue), (B) Lewisx (green) and Mac-1 (blue), (C) CEACAM1 (green) and the DC marker DC-SIGN (red), or (D) Lewisx (green) and DC-SIGN (red). Interactions between CEACAM1- or Lewisx-expressing PMN and DC-SIGN+ DCs are encircled. Insets in the top right corner are magnifications of interacting cells. The anti–CEACAM1 antibody stains colonic crypts because colonic epithelial cells also express CEACAM-1. Bars, 50 μm. Results are representative of three independent experiments.
Activated PMN induce DC maturation through DC-SIGN
Between DCs and interacting cells, such as T cells or NK cells, bidirectional cross talk occurs, resulting in DC maturation and activation of the interacting cells (1, 2). To examine whether the interaction between DCs and PMN would result in DC maturation, we coincubated immature monocyte-derived DCs overnight with resting or activated PMN and determined the expression level of the DC maturation marker CD83. PMN were activated with FMLP, TNF-α, or LPS for 1 h. Because TNF-α and LPS can directly trigger DC maturation, activated PMN were rigorously washed before incubating with DCs. Strikingly, we found that the resting PMN were not able to induce DC maturation, despite the fact that they formed robust cellular clusters (Figs. 1 and and77 A). However, induction of DC maturation was observed with FMLP-, TNF-α–, and LPS-activated PMN, as these PMN induced expression of CD83 on DCs, 18 h after initial cell–cell contact (Fig. 7 A). Expression of the costimulatory molecule CD86, but not of CD80, was also increased (unpublished data). The PMN-induced DC maturation was dependent on cellular interactions, because separation of DC and LPS-activated PMN, using a 0.4-μm Transwell system that only allows transmission of soluble factors, completely abrogated PMN-induced DC maturation (Fig. 7 B). Blocking anti–DC-SIGN antibodies inhibited PMN-induced DC maturation, further supporting the finding that cellular interactions are essential in PMN-induced DC maturation and further demonstrating that these interactions occur through DC-SIGN (Fig. 7 B). The finding that resting PMN can form cellular clusters with immature DCs without inducing DC maturation indicates that the DC-SIGN binding to Mac-1 on PMN is not alone sufficient to trigger DC maturation. It has been previously reported that TNF-α derived from activated PMN may trigger DC maturation (22). Indeed, blocking anti–TNF-α antibodies inhibited DC maturation by LPS-activated PMN (Fig. 7 B). Thus, we conclude that cellular interactions established by Mac-1 and DC-SIGN are essential for efficient delivery of the low amounts of TNF-α secreted by activated PMN to induce DC maturation.
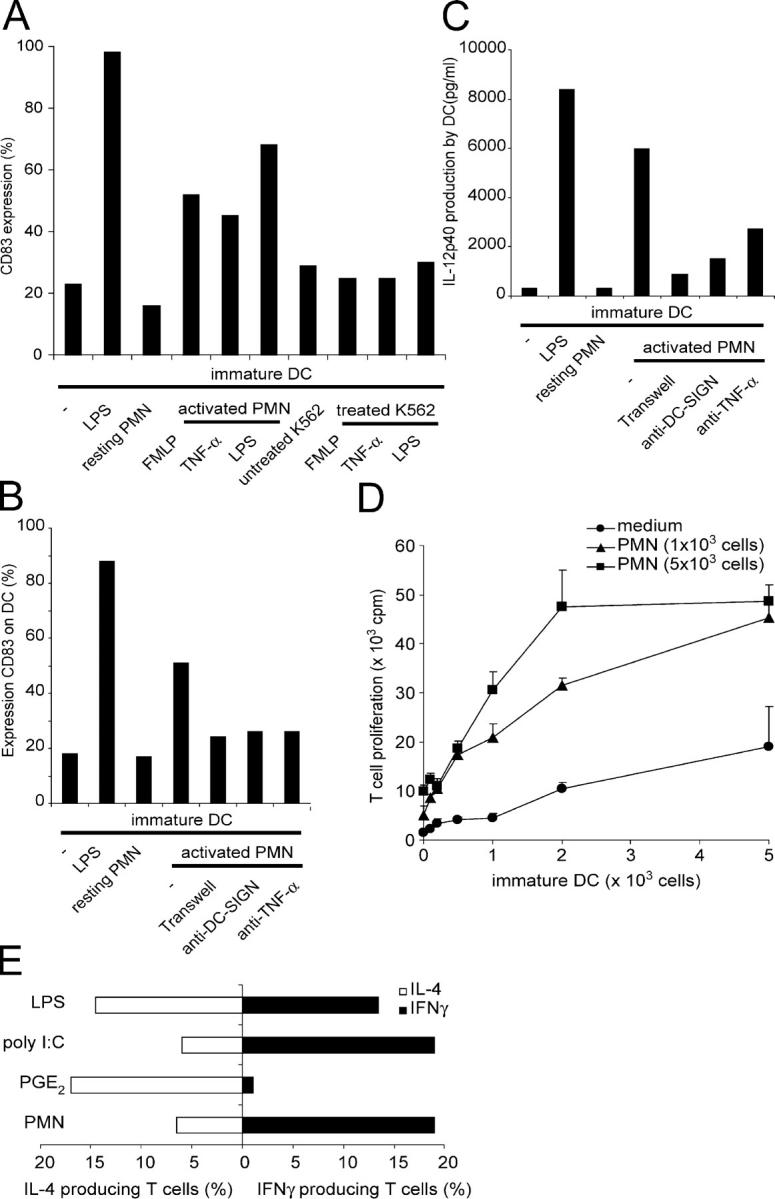
Cross talk between DCs and PMN. (A) The maturation of DCs was induced by activated, but not resting, PMN. Nonstimulated or FMLP-, TNF-α–, or LPS-stimulated PMN and K562 cells (1:3) were incubated with immature DCs for 18 h, and the expression of the DC maturation marker CD83 was determined. The LPS addition to immature DCs was used as a positive control. (B) The DC maturation induced by LPS-activated PMN depends on the cellular contact mediated by DC-SIGN and the secretion of TNF-α. Immature DCs and PMN were cultured together both with and without anti–DC-SIGN antibodies or anti–TNF-α antibodies, as well as cultured separately using a 0.4-μm Transwell system (Corning), and the expression of CD83 on DCs was measured. The LPS addition to immature DCs was used as a positive control. (C) LPS- activated PMN induced the release of the cytokine IL-12p40 by DCs. Both LPS-activated PMN and resting PMN were co-cultured with immature DCs, and the supernatant was examined for IL-12p40 using sandwich ELISA. A 0.4-μm Transwell system separation of PMN and DCs, anti–DC-SIGN antibodies, and anti–TNF-α antibodies was used to block the release of IL-12p40 by DCs after triggering them with LPS-activated PMN. (D) The activated PMN boost DC-induced T cell proliferation. PMN were activated using LPS, rigorously washed, and incubated for 5 d with syngenic T cells and allogenic immature DCs. Overnight [3H]thymidine incorporation was analyzed at day 5 as a measure for T cell proliferation. (E) Activated PMN trigger DCs to induce Th1 cell polarization. DCs were stimulated with LPS in the absence or presence of PMN, and the percentage of IL-4– and IFNγ-producing T cells was analyzed upon restimulation. Poly IC was used as a positive control for Th1 cell polarization, whereas PGE2 was used for Th2 cell polarization. One out of three independent experiments is shown.
Next to DC maturation, we investigated whether PMN trigger cytokine production upon interactions with DCs. We observed that LPS-activated, but not resting, PMN induced the production of IL-12p40 by DCs (Fig. 7 C). Similar to the induction of DC maturation, production of IL-12p40 by DCs was abrogated by the separation of PMN and DCs using a 0.4-μm Transwell system, by anti–DC-SIGN antibodies, and by anti–TNF-α antibodies (Fig. 7 C). Although DCs produced low levels of IL-12p70 upon LPS stimulation, the bioactive heterodimer of IL-12 was not detected after incubation of DCs with activated PMN (unpublished data). Thus, in contrast to resting PMN, activated PMN have an instructive role in DC maturation and cytokine production by DCs through interactions of Mac-1 with DC-SIGN and TNF-α signaling.
Because activated PMN trigger DC maturation, and mature DCs stimulate more potent T cell responses than immature DCs, we investigated whether activated PMN boost T cell proliferation in an MLR. Therefore, we incubated syngenic LPS-activated PMN and T cells together with allogenic immature DCs and determined T cell proliferation at day 5. Indeed, DC-induced T cell proliferation was enhanced in the presence of activated PMN (Fig. 7 D). IL-12 is a Th1 cell–inducing cytokine that is produced upon stimulation of immature DCs by activated PMN, and we therefore investigated whether PMN were able to induce DC1 that trigger Th1 cell polarization. In the presence of LPS, DCs induced a mixed T cell response, consisting of equal numbers of IL-4– and IFNγ-producing T cells (Fig. 7 E). The addition of PMN skewed this T cell response into a Th1 cell response that is comparable with that of the Th1 cell–inducing factor poly IC, but contrasts with the Th2 cell response induced by the Th2 cell–inducing factor PGE2 (Fig. 7 E). Thus, PMN stimulation of DCs enables these DCs to induce Th1 cell polarization. We therefore conclude that PMN may indirectly influence the outcome of T cell responses through interactions with DCs, particularly when the innate response leads to strong activation of PMN.
Discussion
Neutrophils are the dominant effector cells of the innate immune system that phagocytize extracellular bacteria and release antimicrobial compounds during early infection. This enables them to contain the microbial infection and allows time for the establishment of adaptive immune responses that achieve sterile clearance. Recently, it has become clear that neutrophils may pass information on to other players of the innate and adaptive immune response, as they are able to release proinflammatory cytokines and chemokines that attract other neutrophils, activated T cells, monocytes, macrophages, and immature DCs (31). We demonstrate that regulated cellular interactions between neutrophils and immature DCs contribute to neutrophil-induced adaptive responses.
Cell-specific glycosylation regulates DC–PMN interactions
Previously, ICAM-2 and -3 have been described as cellular ligands of DC-SIGN on endothelial cells and T cells, respectively (4, 7). Here, we have identified Mac-1 as a novel high-affinity cellular DC-SIGN ligand that mediates interactions between DCs and PMN with its PMN-specific glycosylation pattern. Both recombinant and cellular DC-SIGN specifically bind native Mac-1 that is expressed on PMN. The finding that DC-SIGN binds Mac-1 on PMN is striking, because neutrophils also express the DC-SIGN ligand ICAM-3. However, ICAM-3 derived from PMN does not bind DC-SIGN, in contrast to ICAM-3 expressed by other cells (4). This is likely because of the fact that ICAM-3 from PMN does not contain high-mannose structures or Lewis antigens, as DC-SIGN displays an affinity for those carbohydrate structures (6, 10). Because glycosylation is a cell-specific process that depends on the expression levels of many glycosyltransferases and the protein backbone of the glycoprotein, neutrophils may express an alternatively glycosylated glycoform of ICAM-3 that is not recognized by DC-SIGN. This illustrates the importance of the cellular source of ICAM-3 in establishing binding to DC-SIGN.
Similar to ICAM-3, cell-specific glycosylation of Mac-1 determines binding to DC-SIGN. Mac-1 is expressed on cells of myeloid origin (32). Although we observed strong binding of DC-SIGN to PMN and to Mac-1 isolated from PMN, DC-SIGN bound poorly to Mac-1 derived from monocytes and immature DCs. This low binding activity of DC-SIGN to isolated monocyte- or DC-derived Mac-1 was insufficient to support binding of DC-SIGN to monocytes or DCs. This indicates that PMN contain a distinct glycoform of Mac-1 with specificity for DC-SIGN. Indeed, in contrast to Mac-1 of monocytes and immature DCs, Mac-1 of PMN contains Lewisx (27, 28), a high-affinity carbohydrate structure that functions as a ligand of DC-SIGN (10). Other carbohydrates that are present on Mac-1 from monocytes and DCs may have initiated the low level of DC-SIGN binding to isolated monocyte- and DC-derived Mac-1. In addition to Mac-1, PMN express the β2-integrins LFA-1 and p150,95. These β2-integrins neither express Lewisx nor bind DC-SIGN, indicating that the expression of Lewisx determines binding to DC-SIGN. Indeed, we were able to identify Lewisx as a determinant on PMN Mac-1 for binding to DC-SIGN by the enzymatic treatment of Mac-1 that specifically removes the Lewisx epitope. Whether Lewisx is the only glycan involved in the binding to DC-SIGN is currently unclear. We observed residual binding of DC-SIGN to PMN Mac-1 after removal of Lewisx, indicating that other carbohydrates on PMN Mac-1 participate in the interaction with DC-SIGN. These carbohydrates do not include the DC-SIGN sugar ligands Lewisa and Lewisb, because neutrophils do not express these Lewisx-related carbohydrates (unpublished data). In contrast, the Lewisx-related structure Lewisy, which has also been described as a DC-SIGN ligand, has been reported to be expressed at low levels on neutrophils (33). Yet, we could neither detect Lewisy on neutrophils nor on Mac-1 derived from neutrophils using our antibody (unpublished data). A detailed carbohydrate analysis of Mac-1 from neutrophils by mass spectrometry is essential to determine the exact carbohydrate structures that are recognized by DC-SIGN.
Novel cellular interactions within the innate immune system
We have shown that immature monocyte-derived DCs and PMN cluster in vitro and that the interaction is mediated by DC-SIGN. Although we have established Mac-1 as a ligand of DC-SIGN by immunoprecipitation, ELISA, and bead adhesion assays, we were unable to study the involvement of Mac-1 in the DC–neutrophil cluster experiments, because of a general lack of anti–Mac-1 antibodies that block the DC-SIGN–Mac-1 interaction (unpublished data). A future analysis of the interaction of DCs with neutrophils from LAD patients that do not have CD18 and, therefore, lack Mac-1 may be an elegant way to address this issue. However, conclusions from cell–cell adhesion experiments with LAD neutrophils should be drawn with caution, because LAD patients also lack LFA-1, which is an important adhesion receptor involved in many cellular interactions.
Under physiological conditions, neutrophils and immature DCs localize in different compartments; neutrophils are mainly present within the blood, whereas immature DCs appear as sentinel cells within the peripheral tissues. In contrast, neutrophils and DCs both accumulate at the site of infection during inflammation. Moreover, neutrophils are able to actively attract immature DCs by the release of chemokines like MIP-1α and MIP-1β (19) and by chemoattractants such as α-defensins (21). In turn, DCs have been demonstrated to transiently produce the neutrophil chemoattractant IL-8 shortly after receiving a maturation stimulus (34). Thus, DCs and neutrophils are likely to encounter each other at peripheral sites during inflammation. Indeed, we were able to demonstrate that DCs and PMN associate in vivo in colonic mucosa during chronic inflammation in patients with Crohn's disease. The colonic mucosa harbors two DC populations that can be distinguished by the expression of CD83 and DC-SIGN (30). CD83+ DCs associate with T cells in lymphoid structures, whereas DC-SIGN+ DCs do not; rather, they are scattered throughout the colonic mucosa. DC-SIGN+ DCs may play a major role in the pathogenesis of Crohn's disease, as they express the Th1 cell–polarizing cytokines IL-12 and -18 that would enable them to induce Th1 cell lymphocytes, which are a main cause of pathology in Crohn's disease (30). As we have demonstrated that the DC-SIGN+ DCs primarily interact with PMN within the colonic mucosa, cross talk between these cells may occur.
Next, we considered whether the cellular interaction between DCs and PMN, especially activated PMN, might modulate DC function. DC maturation is crucial for the establishment of adequate T cell responses, as it is essential for DC migration to draining lymph nodes, and for antigen delivery and activation of T cells. Invariant pathogen structures that bind Toll-like receptors, as well as endogenous signals from T, B, and NK cells, trigger DC maturation (3). We have demonstrated that activated, but not resting, PMN induce DC maturation and that this results in the increased capacity of these DCs to induce T cell proliferation. Thus, PMN require stimulation by other signals such as FMLP, TNF-α, or LPS before they are able to trigger DC maturation. Because we were unable to show that interactions between immature DCs and PMN directly activate PMN (unpublished data), this may ensure that PMN activate and mature DCs only during infection. PMN-induced DC maturation depends completely on cellular interactions with DC-SIGN and Mac-1. Because resting PMN adhere to DCs but do not establish DC maturation, DC-SIGN–Mac-1 interactions are likely essential to establishing a cellular contact zone, or synapse, that is required for the transmission of other maturation signals. It has recently been shown in mice that TNF-α in PMN-conditioned medium triggers maturation of DCs (22). We demonstrate that TNF-α is involved in DC maturation by human PMN. We therefore conclude that the PMN-induced DC maturation is mediated by a two-step model in which DC-SIGN–Mac-1 interactions establish a synapse-like structure between PMN and immature DCs to facilitate TNF-α transmission to DCs that will lead to DC maturation.
Neutrophils have been implicated in the Th1 cell polarization of T cells. In murine infection models, antibody-mediated depletion of PMN shifts the Th1 cell response against Candida albicans, Legionella pneumophila, and H. pylori toward a Th2 cell response (23–25). It has been suggested that neutrophils provide a Th1 cell cytokine environment by the production of IL-12 (35). However, neutrophils produce low amounts of IL-12, and only a few neutrophils reach the lymph nodes, where T cell polarization occurs. Our data support the hypothesis that neutrophils induce Th1 cell polarization indirectly through interactions with DCs. Indeed, we showed that activated PMN induce the release of high levels of the Th1 cell–polarizing cytokine IL-12 by DCs, and enable these DCs to induce Th1 cell responses. Strikingly, DC-SIGN+ DCs within the colonic mucosa in Crohn's disease that associate with PMN express IL-12 (30). Thus, peripheral interactions of neutrophils with immature DCs may result in the development of mature DCs of the DC1 type that migrate to the lymph nodes and induce Th1 cell responses through the production of IL-12. However, we were only able to detect IL-12p40, not bioactive IL-12p70 (unpublished data). Because DCs produce IL-12p40 in 100–1,000-fold excess over IL-12p70, the production of IL-12p70 may have been below the detection limit.
Neutrophils have a higher phagocytic potential than DCs (36). Moreover, antigens taken up by neutrophils are efficiently degraded, whereas antigens acquired by DCs can remain intact for prolonged times (37, 38). In contrast to DCs, neutrophils do not express MHC class II and costimulatory molecules, and, therefore, possess a poor capacity to present antigen-derived peptides to naive T cells. However, in vitro experiments have shown that neutrophils may assist DCs in the acquisition of peptide antigens, because neutrophils regurgitate peptides derived from phagocytized antigens, and these expelled peptides can be taken up by neighboring DCs to allow efficient presentation to T cells (39). Future studies will address whether cellular interactions between neutrophils and DCs are required for antigen transfer and whether these cellular interactions occur through Mac-1 and DC-SIGN.
In conclusion, we establish Mac-1 as a novel ligand of DC-SIGN that mediates adhesion of DCs to PMN, and show that this interaction provides a novel cellular link between innate and adaptive immunity under inflammatory conditions. We therefore hypothesize that neutrophils become activated after an encounter with pathogens, and they not only attract but also instruct immature DCs to elicit the appropriate immune responses.
Materials and Methods
Antibodies and reagents.
The following antibodies were used: ED8 (mouse IgG1 isotype control; reference 40), SPV-L7 (LFA-1, CD11a; reference 41), bear-1 (41), and KIM225 (Mac-1, CD11b; gift of M. Robinson, Celltech Ltd., Slough, UK); HC1/1 (p150,95, CD11c; reference 42), 6.7 (43), NKI-L19, and 60.3 (β2-integrins, CD18; reference 44); and MEM04 (ICAM-3, CD50; gift of V. Horesij, Institute of Molecular Genetics, Prague, Czech Republic), 6H3 (Lewisx, CD15; gift of B.J. Appelmelk, Vrije Universiteit Medical Center), F3 (Lewisy, CD174; Calbiochem), T174 (Lewisa; Calbiochem), T218 (Lewisb; Calbiochem), AZN-D1, AZN-D2, and CSRD (DC-SIGN, CD209; references 4, 45). CD16-biotin and CD83-PE were purchased from BD Biosciences, and CLB-gran/10 (CEACAM1, CD66a) was purchased from Sanquin.
Recombinant DC-SIGN consists of the extracellular portion of DC-SIGN (amino acid residues 64–404) fused at the COOH terminus to the human IgG1-Fc domain (26). DC-SIGN-Fc was produced in Chinese hamster ovary K1 cells after transfection with the DC-SIGN-Sig-pIgG1-Fc vector (20 μg). ICAM-3-Fc (gift of D.L. Simmons, SmithKline Beecham Pharmaceuticals, Harlow, UK) containing an Fc domain of the same isotype was used as a negative control (control-Fc).
Cells.
Stable transfectants of K562 cells expressing DC-SIGN were obtained by electroporation of pRC-CMV-DC-SIGN (400 V, 960 μF) as previously described (46).
PMN were isolated from the fresh blood of healthy volunteers. After performing Ficoll gradient centrifugation, PMN were cleared from contaminating erythrocytes using an erylysis buffer (155 mM NH4Cl, 10 mM KHCO3, and 0.1 mM EDTA). Isolated PMN were >95% pure as assessed by CD11a, CD11b, CD11c, CD18, CD66acd, CD66b, and Lewisx expression. Activated PMN expressed higher levels of CD11b and CD18 compared with resting PMN.
Immature and mature DCs were derived from monocytes as previously described (47, 48). Isolated monocytes were cultured in RPMI 1640 with 10% FCS in the presence of 600 U/ml IL-4 and 800 U/ml GM-CSF (Schering-Plough) for 7 d to obtain immature DCs; and in the presence of 2 μg/ml LPS (derived from Escherichia coli; Sigma-Aldrich) in the final 2 d to obtain mature DCs. Immature DCs expressed no or low levels of CD80, CD83, CD86, and HLA-DR, and high levels of DC-SIGN; mature DCs expressed high levels of CD80, CD83, CD86, and HLA-DR, and low levels of DC-SIGN.
Immunoprecipitation and Western blotting.
PMN were surface biotinylated with 0.5 mg/ml sulfo-NHS-biotin (Pierce Chemical Co.) in PBS for 30 min at 4°C, and subsequently lysed in lysis buffer (1% Triton X-100, 10 mM TEA pH 8.2, 150 mM NaCl, 1 mM MgCl2, and 1 mM CaCl2) containing a cocktail of EDTA-free protease inhibitors (Roche Diagnostics) for 1 h at 4°C. DC-SIGN ligands were immunoprecipitated with recombinant DC-SIGN coated onto protein A–Sepharose beads (CL-4B; GE Healthcare). Immunoprecipitates were analyzed by SDS-PAGE (7% polyacrylamide gel under reducing conditions), transferred onto a nitrocellulose blot, and immunoblotted with streptavidin-peroxidase (Vector Laboratories) or with anti–Mac-1 antibodies and secondary peroxidase-conjugated goat anti–mouse antibodies (Jackson ImmunoResearch Laboratories).
Similarly, Mac-1 was immunoprecipitated from biotinylated PMN lysates with anti–Mac-1 antibodies coated onto protein A beads. Next, immunoprecipitated Mac-1 was analyzed by SDS-PAGE, transferred onto a nitrocellulose blot, and stained with streptavidin-peroxidase, anti-Lewisx antibodies, and secondary peroxidase-conjugated anti–mouse IgM antibodies (Nordic Immunological Lab.) or stained with recombinant DC-SIGN and secondary peroxidase-conjugated goat anti–human antibodies (Jackson ImmunoResearch Laboratories).
Fluorescent bead adhesion assay.
Native Mac-1 that was derived from PMN was coated onto carboxylate-modified TransFluoSpheres (488/645 nm, 1.0 μm; Molecular Probes; reference 49). In brief, streptavidin was covalently coupled onto TransFluoSpheres as described by the manufacturer. Subsequently, streptavidin-coated beads were incubated with biotinylated goat anti–mouse Fc Fab2 fragments (5 μg/ml; Jackson ImmunoResearch Laboratories) in PBS 0.5% BSA for 2 h at 37°C. Thereafter, beads were washed, incubated for 18 h at 4°C with mouse antibodies directed against Mac-1, washed again, and incubated for 48 h at 4°C with PMN lysate (20 × 106 cells/ml) to capture PMN Mac-1 onto the beads.
K562-DC-SIGN cells were incubated with PMN Mac-1–coated beads for 45 min at 37°C in TSM (20 mM Tris-HCl, pH 8.0, 150 mM NaCl, 1 mM CaCl2, and 1 mM MgCl2) 0.5% BSA. After washing, adhesion of the beads to the cells was assessed by flow cytometry (FACS Calibur; Beckton Dickinson).
DC-SIGN-Fc binding.
Cells were incubated with DC-SIGN-Fc for 30 min at 37°C in TSM 0.5% BSA, and subsequently with FITC-conjugated goat anti–human secondary antibodies (Jackson ImmunoResearch Laboratories) for 30 min at 37°C in TSM 0.5% BSA. Binding of recombinant DC-SIGN to the cells was monitored by flow cytometry.
ELISA-based binding assay.
The DC-SIGN-Fc binding assay to native Mac-1 derived from PMN was performed as follows: 4 μg/ml of goat anti–mouse antibodies (Jackson ImmunoResearch Laboratories) were coated onto ELISA plates (NUNC maxisorp; Nunc) for 1 h at 37°C. Thereafter, ELISA plates were blocked with TSM 1% BSA for 30 min at 37°C. After incubating with 1 μg/ml nonblocking mouse antibodies directed against Mac-1 for 1 h at 37°C, ELISA plates were washed and incubated with PMN lysate (20 × 106 cells/ml) for 18 h at 4°C to allow the capture of PMN Mac-1. Then, 1 μg/ml DC-SIGN-Fc was added and allowed to bind for 2 h at room temperature. Unbound DC-SIGN-Fc was washed away, and binding was determined using a peroxidase-conjugated goat anti–human Fc antibody.
Cell–cell adhesion.
To monitor cellular interactions between PMN and either K562-DC-SIGN or DCs, cells were labeled with fluorescent dyes. PMN were labeled with the green fluorescent dye CFSE (5 μM in PBS; Molecular Probes) for 15 min at 37°C. K562-DC-SIGN and DCs were labeled with the red fluorescent dye hydroethidin (40 μg/ml in Iscoves 5% FCS; Polysciences) for 15 min at 37°C. Labeled cells were coincubated for the times indicated in Fig. 1, and adhesion was visualized by fluorescence microscopy and quantified by FACS analysis. Specificity was determined using 50 μg/ml of blocking anti–DC-SIGN antibodies (AZN-D1).
Coculture experiments.
PMN were pulsed for 1 h with 100 ng/ml LPS, rigorously washed, and then incubated with allogenic immature DCs at a ratio of 3:1 for 18 h at 37°C in RPMI 1640 with 10% FCS. Maturation of DCs was assessed by flow cytometry analysis of the expression of the DC maturation marker CD83, and analysis of the production of IL-12p40 in the supernatant was performed using sandwich ELISA according to the manufacturer's protocol (Biosource International). Incubation of DCs with 100 ng/ml LPS was used as a positive control for DC maturation. For T cell proliferation, PBLs were cocultured with syngenic LPS-activated PMN and allogenic immature DCs for 5 d. At day 4, cell cultures were pulsed with 0.5 μCi [3H]thymidine (GE Healthcare) for 18 h, and [3H]thymidine incorporation was measured using a liquid scintillation counter (PerkinElmer). For T cell polarization, DCs were cocultured with PMN (1:3) in the presence of 10 ng/ml LPS for 2 d, washed, and incubated with CD45RA+ CD4+ T cells (5,000 T cells/20,000 DCs). Quiescent T cells were restimulated with 10 ng/ml PMA (Sigma-Aldrich) and 1 μg/ml ionomycin (Sigma-Aldrich) for 6 h. During the last 5 h of that time, they were in the presence of 10 μg/ml brefeldin A (Sigma-Aldrich). Single cell production of IL-4 and IFNγ was determined by intracellular flow cytometry.
Acknowledgments
We thank A.A. te Velde for the kind gift of colonic tissue samples from patients with Crohn's disease, as well as B.J. Appelmelk, I. van Die, D. Roos, T. Kuijper, and G. Kraal for helpful discussions.
This work is supported by the Dutch Scientific Research Council (grant 901-07-220) and the Dutch Cancer Society (grant 2002-2558).
The authors have no conflicting financial interests.
References
Articles from The Journal of Experimental Medicine are provided here courtesy of The Rockefeller University Press
Full text links
Read article at publisher's site: https://doi.org/10.1084/jem.20041276
Read article for free, from open access legal sources, via Unpaywall:
http://jem.rupress.org/content/201/8/1281.full.pdf
Citations & impact
Impact metrics
Article citations
Lower leukocytes pretreatment as a possible risk factor for therapy-induced leukopenia in interferon-beta-treated patients with multiple sclerosis.
Ther Adv Neurol Disord, 17:17562864241286497, 24 Oct 2024
Cited by: 0 articles | PMID: 39479177 | PMCID: PMC11523160
Innate immune response in acute critical illness: a narrative review.
Ann Intensive Care, 14(1):137, 04 Sep 2024
Cited by: 0 articles | PMID: 39227416 | PMCID: PMC11371990
Review Free full text in Europe PMC
Predictive biomarkers and initial analysis of maternal immune alterations in postpartum preeclampsia reveal an immune-driven pathology.
Front Immunol, 15:1380629, 30 Apr 2024
Cited by: 0 articles | PMID: 38745664 | PMCID: PMC11091301
The different impact of drug-resistant Leishmania on the transcription programs activated in neutrophils.
iScience, 27(5):109773, 18 Apr 2024
Cited by: 1 article | PMID: 38711445 | PMCID: PMC11070714
The Exploitation of the Glycosylation Pattern in Asthma: How We Alter Ancestral Pathways to Develop New Treatments.
Biomolecules, 14(5):513, 24 Apr 2024
Cited by: 0 articles | PMID: 38785919 | PMCID: PMC11117584
Review Free full text in Europe PMC
Go to all (252) article citations
Similar Articles
To arrive at the top five similar articles we use a word-weighted algorithm to compare words from the Title and Abstract of each citation.
Interactions of DC-SIGN with Mac-1 and CEACAM1 regulate contact between dendritic cells and neutrophils.
FEBS Lett, 579(27):6159-6168, 13 Oct 2005
Cited by: 61 articles | PMID: 16246332
Dendritic cells recognize tumor-specific glycosylation of carcinoembryonic antigen on colorectal cancer cells through dendritic cell-specific intercellular adhesion molecule-3-grabbing nonintegrin.
Cancer Res, 65(13):5935-5944, 01 Jul 2005
Cited by: 102 articles | PMID: 15994972
Two way communication between neutrophils and dendritic cells.
Curr Opin Pharmacol, 6(4):408-413, 05 Jun 2006
Cited by: 39 articles | PMID: 16750420
Review
Dendritic cell activation by sensing Mycobacterium tuberculosis-induced apoptotic neutrophils via DC-SIGN.
Hum Immunol, 71(6):535-540, 11 Mar 2010
Cited by: 19 articles | PMID: 20219612