Abstract
Free full text

PilZ Domain Proteins Bind Cyclic Diguanylate and Regulate Diverse Processes in Vibrio cholerae*
Abstract
Cyclic diguanylate (c-di-GMP) is an allosteric activator and second messenger implicated in the regulation of a variety of biological processes in diverse bacteria. In Vibrio cholerae, c-di-GMP has been shown to inversely regulate biofilm-specific and virulence gene expression, suggesting that c-di-GMP signaling is important for the transition of V. cholerae from the environment to the host. However, the mechanism behind this regulation remains unknown. Recently, it was proposed that the PilZ protein domain represents a c-di-GMP-binding domain. Here we show that V. cholerae PilZ proteins bind c-di-GMP specifically and are involved in the regulation of biofilm formation, motility, and virulence. These findings confirm a role for PilZ proteins as c-di-GMP-sensing proteins within the c-di-GMP signaling network.
Bis(3′,5′)-cyclic diguanylic acid (c-di-GMP)3 is a cyclic dinucleotide that has recently been recognized as an important intracellular signaling molecule in diverse bacteria. c-di-GMP was originally identified in Gluconacetobacter xylinus (formerly Acetobacter xylinum) as a positive allosteric regulator of cellulose synthase (1–3). Since its discovery almost 20 years ago, c-di-GMP has been implicated in regulation of a variety of biological processes, including production of extracellular polysaccharide matrices in Salmonella enterica serovar Typhimurium, Pseudomonas aeruginosa, Yersinia pestis, and Vibrio cholerae (4–7); motility in S. enterica Typhimurium, P. aeruginosa, Escherichia coli, and Caulobacter crescentus (7, 9–11); and virulence in S. enterica Typhimurium and V. cholerae (12, 13).
The intracellular concentration of c-di-GMP is regulated by the opposing activities of diguanylate cyclase (DGC) and phosphodiesterase A (PDEA) enzymes (14, 15). DGCs catalyze formation of c-di-GMP through the 3′ to 5′ linkage of two GTP molecules into a cyclic molecule releasing two pyrophosphate molecules. Some DGCs contain a feedback-inhibitory site (I-site) that binds dimeric c-di-GMP (16), and thus the I-site comprises one type of c-di-GMP binding domain. PDEAs bind c-di-GMP and degrade it via linearization to pGpG; the linear intermediate is further hydrolyzed to two GMP molecules by a second, presumably ubiquitous phosphodiesterase B (16, 17). Thus, PDEAs must also contain a c-di-GMP binding site, although this site has yet to be defined. The DGC and PDEA activities have been attributed to proteins containing GGDEF and EAL domains, respectively, each named for conserved amino acid residues (17–22). In addition, the HD-GYP domain proteins have recently been shown to degrade c-di-GMP (23).
In V. cholerae, the causative agent of cholera, c-di-GMP is thought to play a role in mediating the transition from environmental reservoirs to the human host. This bacterium normally inhabits temperate waters around the world, including salt, brackish, and some fresh waters (24). Upon entry into a human host via ingestion of contaminated food or water, the bacteria pass through the gastric acid barrier of the stomach and colonize the small intestine. As the bacterium transitions from its natural environment to that of the small intestine, it undergoes a shift from environmental to virulence gene expression (25–29). Depending on the environmental niche, these changes may include a decrease in transcription of Vibrio exopolysaccharide synthesis (vps) genes required for biofilm formation (30). In all cases, however, entry into the small intestine is believed to induce the expression of virulence factors, including the toxin co-regulated pilus, required for colonization of the small intestine, and cholera toxin (CT), the factor responsible for profuse secretory diarrhea (29, 31). It has been shown that c-di-GMP inversely regulates transcription of vps and virulence genes in V. cholerae, where a high level of c-di-GMP favors expression of vps genes and repression of CT expression, and vice versa for a low level of c-di-GMP (8, 13).
There is evidence that V. cholerae forms biofilms on surfaces such as the chitinous exoskeleton of zooplankton and phytoplankton, suggesting that intracellular c-di-GMP levels may be high in V. cholerae inhabiting environmental reservoirs (32–34). In the classical biotype of V. cholerae, it has been shown that expression of the PDEA VieA positively regulates virulence gene expression and negatively regulates vps expression (8, 13, 17). This information supports the model that c-di-GMP assists in the transition from environment to host via modulation of gene expression. However, the mechanism(s) underlying c-di-GMP-mediated regulation, including how changes in c-di-GMP concentration within the cell are sensed, remain unknown.
Recently, Amikam and Galperin (35) reported the in silico identification of a putative c-di-GMP-binding protein domain, PilZ (Pfam domain PF07238) (36), which is distinct from the DGC I-site and PDEA c-di-GMP binding domains. Evidence in support of this claim is as follows. 1) Three proteins known to bind c-di-GMP contain a PilZ domain, including cellulose synthase from G. xylinus, YcgR from E. coli, and PA4608 from P. aeruginosa (3, 37, 38, 39, 40). 2) Several PilZ-containing proteins have been characterized by mutational analysis and appear to regulate phenotypes associated with c-di-GMP-mediated signaling pathways. 3) The phyletic distribution of PilZ domains generally parallels those of the GGDEF and EAL domains, including an absolute correlation between PilZ, GGDEF, and EAL domains in the γ-proteobacteria family, of which V. cholerae is a member. Recently, the binding of E. coli YcgR to c-di-GMP was confirmed by mutational analysis, and this protein was shown to regulate motility in a c-di-GMP-dependent manner (39).
There are five PilZ coding sequences in the V. cholerae genome, (VC0697, VC1885, VC2344, VCA0042, and VCA0735), each having unknown function. We have designated these plzA, plzB, plzC, plzD, and plzE, respectively. Here, we provide evidence in support of the hypothesis that PilZ acts as a c-di-GMP-binding domain and regulator of downstream processes. We show that PilZ proteins specifically bind c-di-GMP in vitro. Moreover, deletion of a subset of PilZ genes results in changes in biofilm formation, motility, and colonization of the small intestine in an animal model of infection, consistent with their role in the c-di-GMP regulatory network.
EXPERIMENTAL PROCEDURES
Growth Conditions
Bacteria were grown in Luria-Bertani (LB) broth at 37 °C with aeration unless otherwise noted. M9 minimal medium supplemented with 0.5% glycerol, trace metals (1 ml/liter 5% MgSO4, 0.5% MnCl2•4H2O, 0.5% FeCl3, 0.4% trinitriloacetic acid) (41), and 25 mm each of l-Asn, l-Arg, l-Glu, and l-Ser (M9 + NRES) was prepared as previously described (42). Expression from the ParaBAD promoter was induced by the addition of l-arabinose to a final concentration of 0.1%. Expression from the Ptac promoter was induced by the addition of isopropyl-β-d-thiogalactopyranoside to a final concentration of 1 mm. Antibiotics were added when appropriate at the following concentrations: streptomycin, 100 µg/ml; ampicillin, 50 µg/ml; kanamycin, 50 µg/ml; chloramphenicol (Cm), 5 or 10 µg/ml (for V. cholerae and E. coli, respectively).
Plasmid and Strain Construction
All strains and plasmids used in this study are listed in Table 1. Plasmids with oriR6K were propagated in DH5αλpir; all other plasmids were propagated in DH5α. For expression and purification of the V. cholerae PlzA, PlzB, PlzC, PlzD, and PlzE proteins, the corresponding genes were amplified by PCR from V. cholerae O395 genomic DNA using oligonucleotide primers listed in Table 2. Each forward primer includes an XbaI restriction site and a strong ribosome binding site; each reverse primer contains an SphI site. A His6 tag was introduced by including the appropriate sequence in the indicated primer. PlzD and PlzE were amplified to include an N-terminal His6 tag using primers A042HF/C0042R and A735HF/C0735R, respectively. Similarly, PlzA, PlzB, and PlzC were tagged with His6 at the C terminus using primers C0697F/0697HR, C1885F/1885HR, and C2344F/2344HR, respectively. PCR products were digested with SphI and XbaI, ligated into similarly digested pBAD33, and transformed into E. coli DH5α. Correct clones were identified by PCR and/or digestion and by detection of the appropriate sized protein in cell extracts using anti-His5 antibodies (Qiagen). The resulting strains (AC2210, AC2212, AC2213, AC2214, and AC2216) are described in Table 1.
TABLE 1
Bacterial strains and plasmids used in this study
Strain or plasmid | Relevant genotype | Source/Reference |
---|---|---|
E. coli strains | ||
DH5α | F-Δ(lacZYA-argF) U169 recA1 endA1 hsdR17 supE44 thi-1 gyrA96 relA1 | Refs. 53 and 54 |
DH5αλpir | F-Δ(lacZYA-argF) U169 recA1 endA1 hsdR17 supE44 thi-1 gyrA96 relA1 λ![]() | Refs. 53 and 54 |
SM10λpir | thi recA thr leu tonA lacY supE RP4-2-Tc![]() ![]() | Laboratory strain |
HCB137 λDE3 | This work | |
AC1173 | E. coli DH5α pBAD33, Cmr | This work |
AC2069 | E. coli HCB137 λDE3 pSB102, Knr | This work |
AC2210 | E. coli DH5α (pBAD33![]() | This work |
AC2212 | E. coli DH5α (pBAD33![]() | This work |
AC2213 | E. coli DH5α (pBAD33![]() | This work |
AC2214 | E. coli DH5α (pBAD33![]() | This work |
AC2216 | E. coli DH5α (pBAD33![]() | This work |
AC2137 | E. coli DH5α (pMMB67EH![]() | This work |
AC2138 | E. coli DH5α (pMMB67EH![]() | This work |
AC2139 | E. coli DH5α (pMMB67EH![]() | This work |
AC2140 | E. coli DH5α (pMMB67EH![]() | This work |
AC2141 | E. coli DH5α (pMMB67EH![]() | This work |
AC2402 | E. coli DH5α (pBAD33![]() | This work |
AC2403 | E. coli DH5α (pBAD33![]() | This work |
AC2404 | E. coli DH5α (pBAD33![]() | This work |
AC2405 | E. coli DH5α (pBAD33![]() | This work |
AC2406 | E. coli DH5α (pBAD33![]() | This work |
V, cholerae strains | ||
O395 | classical biotype, Smr | Ref. 29 |
AC61 | O395 lacZ![]() | Ref. 55 |
AC744 | O395ΔtoxR, Smr | Ref. 56 |
AC1109 | O395 pMMB67EH, Smr, Apr | Ref. 13 |
AC1539 | AC61ΔflaA, Smr, Tcr | Ref. 8 |
AC1596 | AC61 vieAE170A, Smr, Tcr | Ref. 8 |
AC1815 | AC61 vieAE170A (pMMB67EH), Smr, Tcr, Apr | Ref. 13 |
AC1820 | AC1596 (pAT1817), Smr, Tcr, Apr | Ref. 13 |
AC1869 | AC61 vpsR![]() | Ref. 8 |
AC2143 | O395 (pAC2137), Smr, Apr | This work |
AC2144 | O395 (pAC2138), Smr, Apr | This work |
AC2145 | O395 (pAC2139), Smr, Apr | This work |
AC2146 | O395 (pAC2140), Smr, Apr | This work |
AC2147 | O395 (pAC2141), Smr, Apr | This work |
AC2342 | AC61 ΔplzB, Smr, Tcr | This work |
AC2343 | AC1596 ΔplzB, Smr, Tcr | This work |
AC2344 | AC61 ΔplzD, Smr, Tcr | This work |
AC2345 | AC1596 ΔplzD, Smr, Tcr | This work |
AC2346 | AC61 ΔplzC, Smr, Tcr | This work |
AC2347 | AC1596 ΔplzC, Smr, Tcr | This work |
AC2348 | AC2342 (pAC2140), Smr, Tcr, Apr | This work |
AC2349 | AC2343 (pAC2140), Smr, Tcr, Apr | This work |
AC2350 | AC2344 (pAC2137), Smr, Tcr, Apr | This work |
AC2351 | AC2345 (pAC2137), Smr, Tcr, Apr | This work |
AC2352 | AC2346 (pAC2141), Smr, Tcr, Apr | This work |
AC2353 | AC2347 (pAC2141), Smr, Tcr, Apr | This work |
AC2458 | AC61 plzBR96A, Smr, Tcr | This work |
AC2459 | AC2458 (pAC2140)), Smr, Tcr, Apr | This work |
AC2550 | AC2346 ΔplzD, Smr, Tcr | This work |
AC2551 | AC2346 ΔplzD (pAC2141) Smr, Tcr, Apr | This work |
AC2552 | AC2346 ΔplzD (pAC2137) Smr, Tcr, Apr | This work |
AC2553 | AC2346 plzDR140A Smr, Tcr | This work |
AC2554 | AC2346 plzDR140A (pAC2137) Smr, Tcr, Apr | This work |
Plasmids | ||
pBAD33 | pACYC184 ori, araC ParaBAD, Cmr | Ref. 57 |
pMMB67EH | IncQ broad host range cloning vector, Apr | Ref. 58 |
pAT1817 | pMMB67EH![]() | Ref. 8 |
pAC2137 | pMMB67EH![]() | This work |
pAC2138 | pMMB67EH![]() | This work |
pAC2139 | pMMB67EH![]() | This work |
pAC2140 | pMMB67EH![]() | This work |
pAC2141 | pMMB67EH![]() | This work |
pAC2210 | pBAD33![]() | This work |
pCVD442 | oriR6K mobRP4 sacB, Apr | Ref. 42 |
pJP2337 | pCVD442![]() | This work |
pJP2338 | pCVD442![]() | This work |
pJP2339 | pCVD442![]() | This work |
pJP2340 | pCVD442![]() | This work |
pJP2341 | pCVD442![]() | This work |
pJP2460 | pCVD442![]() | This work |
pSB102 | pET28bhisTEV![]() | This work |
TABLE 2
Primers used in this study
Primer name | Primer sequencea |
---|---|
C0042F | 5′ -TTCTAGATTTAGGATACATTTTTATGAATTCACGGCCCGCT-3′ |
C0042R | 5′ -TTGCATGCTTGTTACGCCTTTTTCTC-3′ |
A042HF | 5′ -TCTAGATTTAGGATACATTTTTATGCATCATCATCATCATCATAATTCACGGCCCGCTGAA-3′ |
C0697F | 5′ -TTCTAGATTTAGGATACATTTTTTTGATGTACCCTTTGCTA-3′ |
C0697R | 5′ -TTGCATGCTTACTCATGCAGTCCTAA-3′ |
0697HR | 5′ -TTGCATGCTCAATGATGATGATGATGATGCTCATGCAGTCCTAAATC-3′ |
C0735F | 5′ -TTCTAGATTTAGGATACATTTTTGTGTATAACTTTGTCAAT-3′ |
C0735R | 5′ -TTGCATGCTTAAATTGAGCCAGCGAG-3′ |
A735HF | 5′ -TTCTAGATTTAGGATACATTTTTATGCATCATCATCATCATCATTATAACTTTGTCAATAATAATA-3′ |
C1885F | 5′ -TTCTAGATTTAGGATACATTTTTATGAATGACCAAGAATT-3′ |
C1885R | 5′ -TTGCATGCTCACGCTTGAGGTTTTTC-3′ |
1885HR | 5′ -TTGCATGCTCAATGATGATGATGATGATGCGCTTGAGGTTTTTCTCG-3′ |
C2344F | 5′ -TTCTAGATTTAGGATACATTTTTATGCAACAGACCGAAATT-3′ |
C2344R | 5′ -TTGCATGCTCAGGTCAACTCAAGTCG-3′ |
2344HR | 5′ -TTGCATGCTCAATGATGATGATGATGATGGGTCAACTCAAGTCGGAT-3′ |
VcYF1a | 5′ -GAATTCCATATGGAGGCAATTTTGAAT-3′ |
VcYR1b | 5′ -CCCAAGCTTCTTTTGCGCGTGTGAATA-3′ |
0042F1 | 5′ -GGTCTAGAACCCACTCTCGTTTTCCTGA-3′ |
0042F2 | 5′ -TACGCCTTGCGTGGAGTCTCTGTTTGG-3′ |
0042R1 | 5′ -CTCCACGCAAGGCGTAACAAATTGGGA-3′ |
0042R2 | 5′ -GGTCTAGAGCATCGCCTTTCCTTATCAA-3′ |
0697F1 | 5′ -GGTCTAGAGATGCTGGCTTAGTGGTGGT-3′ |
0697F2 | 5′ -TGGGATTAAGGGTACATCAAATCACCGGA-3′ |
0697R1 | 5′ -TGTACCCTTAATCCCAAGCCCAAATGGA-3′ |
0697R2 | 5′ -GGTCTAGACGATGGTCGTGTTCTGATTG-3′ |
0735F1 | 5′ -GGTCTAGATATTTGATTGCTTGCGGACA-3′ |
0735F2 | 5′ -TTACGGTGGCTGGCTCAATTTAAGCCA-3′ |
0735R1 | 5′ -GAGCCAGCCACCGTAACGCGGGGTAT-3′ |
0735R2 | 5′ -GGTCTAGATGCTCAATAACGCGATCAAG-3′ |
1885F1 | 5′ -GGTCTAGAGGTAAATTGGCTCGCTTCTG-3′ |
1885F2 | 5′ -ACCAAGAACCTCAAGCGTGACTATGACT-3′ |
1885R1 | 5′ -GCTTGAGGTTCTTGGTCATTCATAGAGGGT-3′ |
1885R2 | 5′ -GGTCTAGACTTGGCTGTAAACCGATTCC-3′ |
2344F1 | 5′ -GGTCTAGACGAATCGATGACCATAATGC-3′ |
2344F2 | 5′ -TTATACCCGAGGAAGTGTTGATCCGACT-3′ |
2344R1 | 5′ -ACTTCCTCGGGTATAAGCCTTTCAACCA-3′ |
2344R2 | 5′ -GGGAGCTCGATGCAAGGTGAGCTGGATT-3′ |
R136F2 | 5′ -CCATGCAAGTCTCTCAATTAGCTAAAGAGCCGAGATTTGAGCT-3′ |
R136R1 | 5′ -AGCTCAAATCTCGGCTCTTTAGCTAATTGAGAGACTTGCATGG-3′ |
R140F2 | 5′ -CTCAATTACGTAAAGAGCCGGCTTTTGAGCTAAATTTAGCCGG-3′ |
R140R1 | 5′ -CCGGCTAAATTTAGCTCAAAAGCCGGCTCTTTACGTAATTGAG-3′ |
D162F2 | 5′ -TGGGGATTGTGAACTGCGCGCTTTATCACGCAGTGGTTGCC-3′ |
D162R1 | 5′ -GGCAACCACTGCGTGATAAAGCGCGCAGTTCACAATCCCCA-3′ |
S164F2 | 5′ -ATTGTGAACTGCGCGATTTAGCTCGCAGTGGTTGCCGTTTCAT-3′ |
S162R1 | 5′ -ATGAAACGGCAACCACTGCGAGCTAAATCGCGCAGTTCACAAT-3′ |
G167F2 | 5′ -TGCGCGATTTATCACGCAGTGCTTGCCGTTTCATCACGCCGCC-3′ |
G167R1 | 5′ -GGCGGCGTGATGAAACGGCAAGCACTGCGTGATAAATCGCGCA-3′ |
R96AR1 | 5′ -TCGCACTGGCTGATTGAGGATCATCTTGTTGT-3′ |
R96AF2 | 5′ -TCAATCAGCCAGTGCGACCACTCGTTTTGGT-3′ |
Point mutations that changed conserved residues in PlzD to alanine were generated by splicing by overlapping extension PCR (43), using pAC2210 as a template. Primers R1 and F2 containing the desired point mutation were used in combination with A0042HF and C0042R, respectively. PCR products were annealed together by complementary sequences in R1 and F2 primers and PCR-amplified using A0042HF and C0042R. The final products were digested with XbaI/SphI and cloned into similarly digested pBAD33. Primer pairs used were R136R1/R136F2, R140R1/R140F2, D162R1/D162F2, S164R1/S164F2, and G167R1/G167F2, with the number corresponding to the amino acid altered.
Plasmids for generating in-frame deletions and point mutations in V. cholerae were constructed in the allelic exchange vector pCVD442, which encodes the sacB counter-selectable marker (44). Splicing by overlapping extension PCR was used to generate all deletions (43). DNA fragments of ~ 800 bp upstream and downstream of each deletion were amplified by PCR from V. cholerae N16961 genomic DNA, annealed together by complementary sequences in the R1 and F2 primers, and PCR-amplified with the F1 and R2 primers. The final PCR product was ligated into pCVD442 following digestion with either XbaI (pJP2337, pJP2338, pJP2339, and pJP2340) or XbaI/SacI (pJP2341). The respective F1/R1 and F2/R2 primer pairs used for generating deletion alleles of plzA, plzB, plzC, plzD, and plzE products were 0697F1/0697R1 and 0697F2/0697R2, 1885F1/1885R1 and 1885F2/1885R2, 2344F1/2344R1 and 2344F2/2344R2, 0042F1/0042R1 and 0042F2/0042R2, and 0735F1/0735R1 and 0735F2/0735R2, respectively. The F1/R1 and F2/R2 primer pairs used for generating the plzBR96A and plzDR140A point mutants were 1885F1/R96AR1 and 1885F2/R96AR2 and 0042F1/R140AR1 and R140AF2/0042R2, respectively.
V. cholerae strains AC2342, AC2344, and AC2346 containing in-frame deletions of plzB, plzD, and plzC, respectively, and AC2458 containing the plzBR96A point mutation were generated by allelic exchange with plasmid pJP2339, pJP2340, pJP2341, and pJP2460, respectively, in the wild-type (AC61) background. Strains AC2343, AC2345, and AC2347 were generated by allelic exchange with plasmid pJP2339, pJP2340, and pJP2341, respectively, in the O395 vieAE170A (AC1596) background. Strain AC2553, containing an in-frame deletion of plzC and the plzDR140 point mutation, was generated by allelic exchange with plasmid pJP2555 in the ΔplzC (AC2346) background. Plasmids were conjugated into AC61, AC1596, and AC2346 from E. coli SM10λpir as previously described (45). After one passage in LB broth in the absence of antibiotics, sucrose-resistant colonies were selected and were subsequently screened for the desired deletion by PCR.
For complementation of PilZ mutant phenotypes, plzD, plzA, plzE, plzB, and plzC were PCR-amplified from V. cholerae O395 genomic DNA using the following primer pairs: C0042F/C0042R, C0697F/C0697R, C0735F/C0735R, C1885F/C1885R, and C2344F/C2344R, respectively (Table 1). The PCR products were digested with SphI and XbaI and cloned into pMMB67EH. Correct clones were identified by PCR and are listed in Table 2 (pAC2137-pAC2141). Complementation plasmids were introduced into V. cholerae by electroporation, and ampicillin-resistant transformants were selected. The resulting strains (AC2342 to AC2353, AC2459, AC2551, AC2552, and AC2554) are described in Table 1.
Expression and Purification of PilZ Proteins and Controls
The five V. cholerae PilZ proteins, five PlzD point mutation derivatives, and CheY were overexpressed in and purified from E. coli. Briefly, AC1173 (parent plasmid control), AC2210, AC2212, AC2213, AC2214, AC2216, AC2402, AC2403, AC2404, AC2405, and AC2406 were grown in LB broth supplemented with Cm for 16 h at 37 °C with aeration. The overnight cultures were diluted 1:200 in 200 ml of fresh LB plus Cm and incubated at 30 °C with aeration to midexponential phase (A600 ~ 0.45). l-Arabinose was added to induce expression. AC1173, AC2069, AC2212, AC2213, AC2214, and AC2216 were then grown at 30 °C for an additional 3 h. AC2210, AC2402, AC2403, AC2404, AC2405, and AC2406 were shifted to 18 °C and grown for 5 h more. The CheY control was similarly expressed in and purified from AC2069. Briefly, an overnight culture of AC2069 was diluted 1:100 in LB plus kanamycin; protein expression was induced with isopropyl-β-d-thiogalactopyranoside followed by 3 h of further growth at 30 °C. Cells were pelleted, and His6-tagged PilZ proteins were purified as described previously (17). The concentration of protein in the resulting preparations was measured using a modified Lowry protein assay kit (Pierce). Ten µg of protein were separated by SDS-PAGE on a 4–15% Tris-HCl precast gel (Bio-Rad) and transferred to a nitrocellulose membrane. PilZ proteins of the expected size were detected using mouse anti-His5 antibodies (Qiagen) and horseradish peroxidase-conjugated sheep anti-mouse antibodies (Amersham Biosciences) (data not shown).
c-di-GMP Binding Assays
Four separate methods were used to detect binding of radiolabeled c-di-GMP to the PilZ proteins. [32P]c-di-GMP was synthesized enzymatically as described previously using VCA0956 (17) or WspR (44) DGCs, which work equivalently, and 0.33 µm [α-32P]GTP (PerkinElmer Life Sciences). In the first method, binding was assayed after denaturing conditions. Ten µg of each PilZ protein, the CheY control, or mock-purified vector control were separated by SDS-PAGE on a 4–15% Tris-HCl precast gel and transferred to a nitrocellulose membrane. The membrane was incubated in phosphate-buffered saline containing 1% skim milk for 0.5 h at room temperature and then probed with 2 nm [32P]c-di-GMP for 2 h. After three 5-min washes with phosphate-buffered saline, radiolabeled bands were visualized by phosphorimaging. In the second method, binding was assayed under native conditions. Each PilZ protein, the CheY control, or mock-purified vector control were separated on a 4–15% Tris-HCl precast gel in the absence of SDS or β-mercaptoethanol, transferred to nitrocellulose membranes, and probed with [32P]c-di-GMP as described above. For the third method, 10 µg of each PilZ protein, the CheY control, or mock-purified vector control were spotted directly onto nitrocellulose strips, allowed to dry for 0.5 h at 37 °C, and then probed with radiolabeled c-di-GMP as described above. For the fourth method, binding was assayed in solution. E. coli strains (AC2137, AC2138, AC2139, AC2140, AC2141, and AC2046) or V. cholerae strains (AC1109, AC2143, AC2144, AC2145, AC2146, and AC2147) were grown in LB broth supplemented with Cm or ampicillin for 16 h at 37 °C with aeration. The overnight cultures were diluted 1:200 in 200 ml of fresh LB plus Cm or ampicillin and incubated at 37 °C with aeration to midexponential phase (A600 ~ 0.45). l-Arabinose or isopropyl-β-d-thiogalactopyranoside was added to induce expression, and cultures were then grown at 30 °C for an additional 3 h. Cells were pelleted and resuspended in c-di-GMP binding buffer (50 mm Tris, pH 7.5, 10 mm MgCl2, 5 mm CaCl2, 5 mm KCl) (3) plus 10% glycerol. All subsequent steps were carried out at 4 °C. The bacterial suspensions were lysed by French press at 16,000 p.s.i and fractionated by centrifugation at 10,000 × g for 30 min. The supernatant was removed, and the protein concentration was determined using a modified Lowry protein assay kit. Either 200 µg of cell lysate or 10 µg of purified PilZ proteins were brought to a volume of 100 µl with c-di-GMP binding buffer and incubated on ice for 10 min with 5 nm [32P]c-di-GMP. Immediately following, 900 µl of cold binding buffer was added, and the total volume was added to the barrel of a 3-ml syringe attached to a Swinnex filter holder (Millipore) containing a 0.22-µm nitrocellulose filter (Millipore). The solution was passed through the filter over a period of ~10 s with consistent pressure. The filter was washed once with 900 µl of cold c-di-GMP binding buffer. Radioactivity associated with the flow-through and filter was measured by liquid scintillation counting.
To measure the KD of c-di-GMP binding by purified PlzC and PlzD proteins, solution binding assays were done as above over a 1–2000 nm range of ligand concentration and using 0.25 µg of protein. Nonlinear regression analysis of the binding data was done using a one-site binding equation to calculate the KD.
PlzC and PlzD were tested for specificity of c-di-GMP binding by spotting the proteins on nitrocellulose strips and probing with 0.013 µm [32P]c-di-GMP alone, combined with 0.7 µm cold c-di-GMP, or combined with 0.7 µm cold GTP, using the methods described above.
Motility Assays
Chemotaxis plates composed of either 1% tryptone, 0.5% NaCl, and 0.3% agar or M9 + NRES and 0.3% agar were used to assess motility of V. cholerae strains. Strains were streaked for single colonies on LB agar plus antibiotics and incubated overnight at 30 °C. Single colonies were removed with a toothpick, stabbed into the chemotaxis plate, and incubated for 16 h at 30 °C.
Crystal Violet Staining of Biofilms
Strains were grown for 16 h in LB plus antibiotics, diluted 1:1000 into 0.5 ml of LB plus antibiotics in new 13-mm diameter borosilicate glass tubes, and incubated at room temperature without aeration for 48 h. The medium was aspirated, and adherent biofilms were gently washed three times with LB. Biofilms were then stained with 0.5 mg/ml crystal violet for 5 min and then washed extensively with water. Bound crystal violet was solubilized with 1 ml of 100% ethanol and quantified by absorbance at 570 nm. Each experiment was performed in triplicate.
Cholera Toxin Quantitation
Strains were grown at 30 °C with aeration overnight in M9 + NRES. The final A600 was determined, cells were pelleted by centrifugation, and the supernatant was filter-sterilized (0.45 µm polyvinylidene difluoride filter). The concentration of CT in supernatants was determined by a GM1 enzyme-linked immunosorbent assay using polyclonal goat antiserum to the CT B subunit (List Biolabs) and anti-goat IgG conjugated to alkaline phosphatase (Sigma) as described previously (47). Purified CT B subunit of known concentration (List Biolabs) was used to generate a standard curve for use in determining the amount of CT B subunit in the samples.
In Vivo Competition Assays
Competition assays using the infant mouse model of infection were performed essentially as described (13). The wild-type and PilZ mutant strains were grown overnight on LB agar plus antibiotics at 37 °C. For each strain, ~10 colonies were resuspended in 200 µl of LB, and the A600 was determined. The strains were mixed 1:1 and adjusted to a final A600 of 0.001 (~106 colony-forming units/ml). Five-day-old CD-1 infant mice were anesthetized by isoflurane (2.5%) inhalation and intragastrically inoculated with 50 µl of this mixture. In vitro competitions were performed in parallel by inoculating 2 µl of the mix into 1 ml of LB and incubating overnight at 37 °C with aeration.
RESULTS
V. cholerae PilZ Domain Proteins PlzC and PlzD Specifically Bind c-di-GMP
To test the hypothesis that proteins containing the PilZ domain serve as c-di-GMP-binding proteins, the five V. cholerae proteins that possess this domain (35) were overexpressed, purified, and assayed for binding to 32P-radio-labeled c-di-GMP using both membrane and solution binding methods detailed under “Experimental Procedures.” Each of the PilZ proteins contains one PilZ domain, with the exception of PlzC, which contains two PilZ domains. There are no assigned functions for any of these proteins nor any other identifiable domains.
After native electrophoretic separation and immobilization on a membrane, two PilZ domain proteins, PlzC and PlzD, bound c-di-GMP (Fig. 1A). The remaining three PilZ proteins as well as the negative controls (chemotaxis response regulator CheY and vector alone mock-purified) were unable to bind c-di-GMP. PlzC and PlzD also bound c-di-GMP after spotting the purified proteins directly onto a nitrocellulose membrane (Fig. 1B). When assayed for binding after denaturing electrophoretic separation, PlzC retained the ability to bind c-di-GMP, whereas PlzD lost the ability to bind (Fig. 1C).

A–C, lanes 1–7, PlzD, PlzE, PlzA, PlzB, PlzC, CheY, and mock-purified, respectively. A, after native electrophoretic separation conditions, proteins were transferred to nitrocellulose and probed with [32P]c-di-GMP. PlzD and PlzC bound c-di-GMP. B, to avoid potential protein denaturation during transfer to nitrocellulose, the PilZ domain proteins and controls were spotted directly onto nitrocellulose and probed. Again, PlzD and PlzC bound c-di-GMP. C, after denaturing electrophoretic separation conditions, proteins were transferred to nitrocellulose, renatured, and probed. Only PlzC bound c-di-GMP. D, to test the specificity of PlzD and PlzC binding to c-di-GMP, proteins spotted to nitrocellulose were probed with [32P]c-di-GMP alone, [32P]c-di-GMP plus excess cold c-di-GMP, or [32P]c-di-GMP plus excess cold GTP, as indicated to the right. Incubation with excess cold c-di-GMP dramatically decreased the amount of binding to [32P]c-di-GMP, whereas incubation with excess cold GTP did not, indicating that binding of c-di-GMP to PlzD and PlzC is specific. The fractions of [32P]c-di-GMP bound in the presence of cold competitor compared with that of c-di-GMP alone are shown below each lane.
We also examined solution binding of PilZ proteins to c-di-GMP using a filter binding assay. Whole cell lysates from E. coli and V. cholerae strains overexpressing each of the PilZ proteins as well as purified His6-tagged PilZ domain proteins were tested for binding of c-di-GMP. Consistent with the above results, only lysates from strains overexpressing PlzC and PlzD and purified PlzC and PlzD showed binding above background levels (data not shown).
We determined the binding affinity of PlzC and PlzD using the filter binding assay. Nonlinear regression analysis of c-di-GMP binding by PlzC and PlzD revealed that the proteins have similar affinities for c-di-GMP. The dissociation constants (KD) for PlzC and PlzD were 314 and 293 nm, respectively.
To assess the specificity of PlzC and PlzD binding to c-di-GMP, the two purified proteins were incubated with a mixture of [32P]c-di-GMP and either excess cold competitor c-di-GMP or excess cold GTP. A molar excess of cold GTP did not interfere with [32P]c-di-GMP binding (Fig. 1D), whereas cold c-di-GMP decreased binding of radiolabeled c-di-GMP by both PlzC and PlzD to the indicated amounts. A molar excess of cold competitor also substantially decreased binding of [32P]c-di-GMP by PlzC and PlzD in filter binding experiments (data not shown). These results indicate that PlzD and PlzC bind c-di-GMP specifically.
Conserved Residues in the PilZ Domain Are Required for Binding of c-di-GMP
Multiple alignment of V. cholerae PilZ domain protein sequences with the PilZ domain from G. xylinus cellulose synthase (AscA1) revealed seven conserved residues clustered toward the N terminus of the domain, consistent with the results of Amikam and Galperin (35) (Fig. 2A). These residues fell into two categories, either highly conserved, where the specific residue was found in six out of the seven PilZ domains examined, or conserved, where a hydrophobic residue was consistently found at a given position in all domains examined. Using the crystal structure of the PlzD dimer (Protein Data Bank entry 1YLN),4 we examined the location of the highly conserved residues (Arg136, Arg140, Asp162, Ser164, and Gly167) and found that each of these residues resides in close proximity on the same face of the PilZ domain of each monomer, suggesting that they may be important for c-di-GMP binding (Fig. 2B). In order to determine if these residues are required for PlzD binding of c-di-GMP, we changed each to an alanine. The subsequent mutants were purified in parallel with the wild-type PlzD and tested for binding of c-di-GMP using the filter binding assay. All five mutant proteins were stably expressed at levels equivalent to the wild type (Fig. 2C). In each case, mutation of a highly conserved residue abrogated binding of c-di-GMP (Fig. 2D).
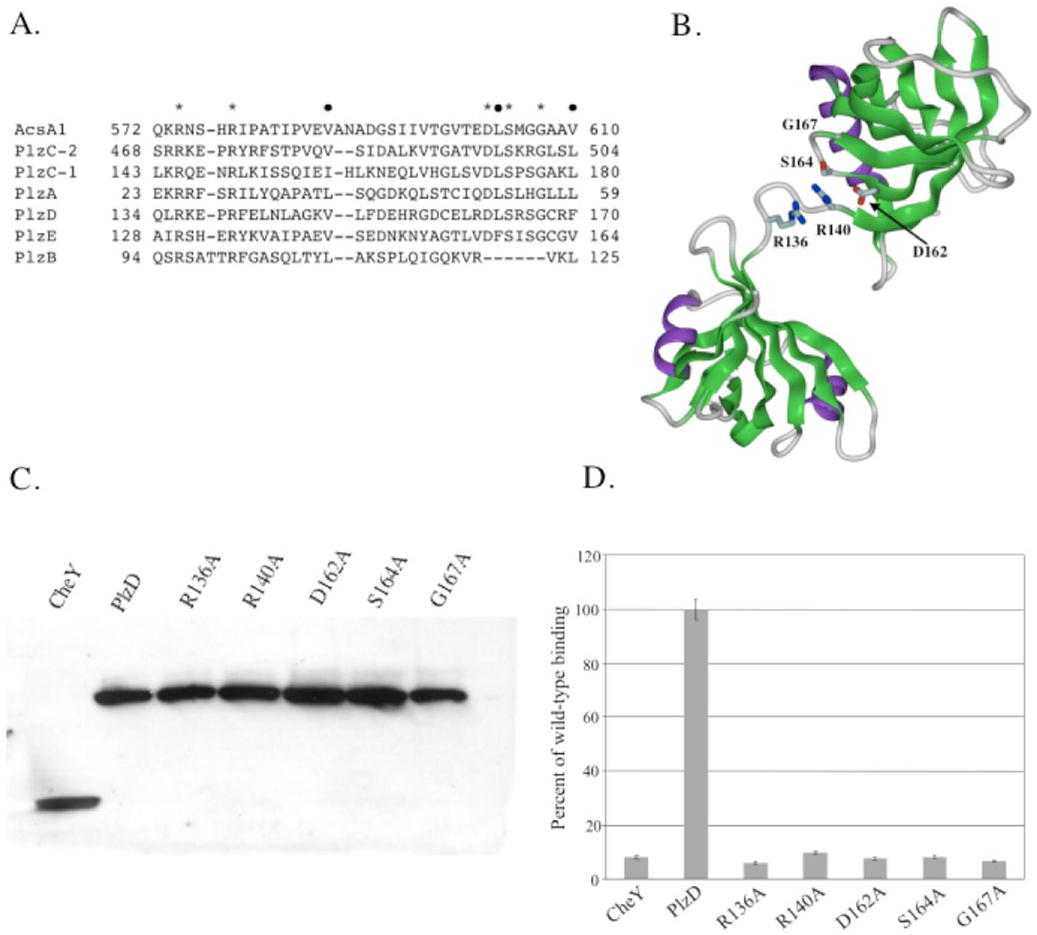
A, multiple alignment of V. cholerae PilZ domains with the first 39 amino acid residues from the AcsA1 PilZ domain using MAFFT (available on the World Wide Web at www.biophys.kyoto-u.ac.jp/~katoh/programs/align/mafft/). V. cholerae proteins are listed by their locus names, and AcsA1 represents the PilZ domain from cellulose synthase 1 from G. xylinus. Each of the two PilZ domains of PlzC are included and labeled accordingly. Highly conserved residues, those present in six of seven PilZ domains, are marked with an asterisk at the top. Conserved hydrophobic residues are marked with a filled circle. B, ribbon diagram of one monomer of the PlzDdimer showing the locations of highly conserved residues targeted for mutation, Arg136, Arg140, Asp162, Ser164, and Gly167. C, Western blot of purified His6-tagged wild-type and point mutant PlzD proteins. Protein purification and Western blots were performed as described under “Experimental Procedures.” D, binding of [32P]c-di-GMP by PlzD point mutants. Binding of 10 µg of each His6-tagged purified protein (CheY, PlzD, PlzDR136A, PlzDR140A, PlzDD162A, PlzDS164A, and PlzDG167A) to [32P]c-di-GMP was measured in solution as described under “Experimental Procedures.” The amount of [32P]c-di-GMP bound is shown relative to that bound by wild-type PlzD. The mean and S.D. values from triplicate assays are shown.
PlzB, PlzC, and PlzD Regulate Motility in V. cholerae
In order to investigate potential roles of V. cholerae PilZ proteins in c-di-GMP-regulated processes, we attempted to make in-frame deletions of each of the five genes in both the wild-type and vieAE170A backgrounds. The vieAE170A strain harbors a point mutation in a conserved residue shown to be required for PDEA activity, leading to an increased cellular concentration of c-di-GMP (8, 17). We were successful in making deletions of plzB, plzC, and plzD. Our results suggest that the other two PilZ genes, plzA and plzE, are essential.
To test whether plzB, plzC, or plzD regulates motility in V. cholerae, we assayed their motility in rich (tryptone-based) and minimal (M9 + NRES) media semisolid agar (chemotaxis) plates for 16 h at 30 °C. As shown in Fig. 3A, deletion of plzB reduces motility in tryptone-based chemotaxis media, whereas deletion of plzC or plzD has no effect. The motility defect of ΔplzB was confirmed using phase-contrast microscopy (data not shown), and this defect was fully complemented by the addition of plzB on a low copy number plasmid (Fig. 3A). The vieAE170A motility phenotype is consistent with our previous report that increased intracellular c-di-GMP concentration leads to a reduction in motility (48). However, there was no restoration of motility in the vieAE170A background by any of the three PilZ gene deletions (Fig. 3A).
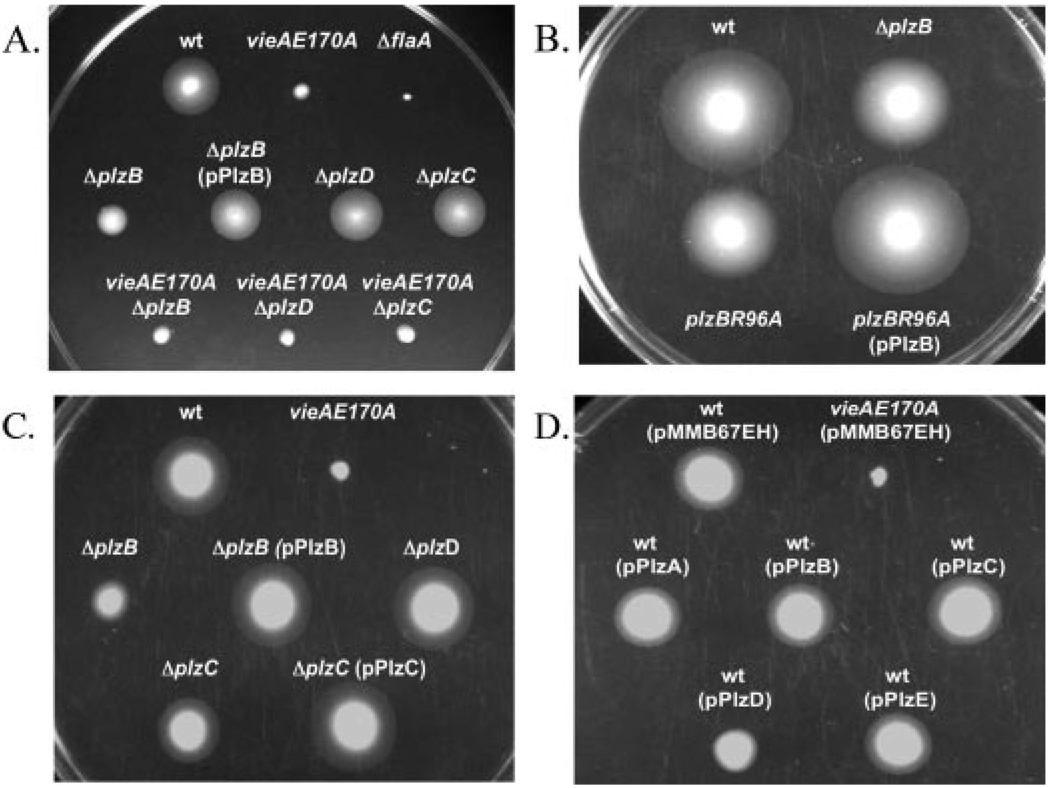
V. cholerae strains were inoculated into chemotaxis plates and incubated at 30 °C for 16 h. A (tryptone-based chemotaxis plate), wild type (wt), AC61; vieAE170A, AC1596; ΔflaA, AC1539; ΔplzB, AC2342; ΔplzB (pPlzB) (pAC2140), AC2348; ΔplzD, AC2344; ΔplzC, AC2346; vieAE170A ΔplzB, AC2343; vieAE170A ΔplzD, AC2345; vieAE170A ΔplzC, AC2347. B (tryptone-based chemotaxis plate), wt, AC61; ΔplzB, AC2342; plzBR96A, AC2458; plzBR96A (pPlzB) (pAC2140), AC2459. Magnification is X 3. C (M9 + NRES chemotaxis plate), wt, AC61; vieAE170A, AC1596; ΔplzB, AC2342; ΔplzB (pPlzB) (pAC2140), AC2348; ΔplzD, AC2344; ΔplzC, AC2346; ΔplzC (pPlzC) (pAC2141), AC2347. D (M9 + NRES chemotaxis plate plus 1 mm isopropyl-β-d-thiogalactopyranoside), wt (pMMB67EH), AC1109; vieAE170A (pMMB67EH), wt (pPlzA) (pAC2138), AC2144; wt (pPlzB) (pAC2140), AC2146; wt (pPlzC) (pAC2141), AC2147; wt (pPlzD) (pAC2137), AC2143; wt (pPlzE) (pAC2139), AC2145.
To test whether the PilZ domain itself is involved in regulation of motility, we assayed motility of a plzB point mutant in a tryptone-based chemotaxis plate. A strain with a mutation in one of the highly conserved arginine residues in PlzB (R96A) had a reduced motility phenotype similar to that of ΔplzB (Fig. 3B). PlzBR96A is stably expressed (data not shown), and the motility defect was fully complemented by the addition of plzB in trans (Fig. 3B).
Experiments using M9 + NRES semisolid agar plates gave slightly different results. As expected, ΔplzB showed decreased motility, but in this condition, ΔplzC also showed a decrease in motility (Fig. 3C). Each of these defects could be fully complemented by the addition of the corresponding gene in trans (Fig. 3C). Therefore, PlzB and PlzC are positive regulators of motility. Again, deletion of plzD had no effect on motility, and no changes in motility were observed for PilZ mutants in the vieAE170A background (Fig. 3C and data not shown).
Because we were not able to delete two of the PilZ genes, V. cholerae strains overexpressing each of the five PilZ proteins were analyzed for potential phenotypes in both conditions. Overexpression of each protein in either the wild-type or vieAE170A background had no effect on motility in tryptone-based chemotaxis plates (data not shown). However, overexpression of PilZ domain proteins in M9 + NRES chemotaxis plates gave different results. In the wild-type background, over-expression of plzC led to a slight increase in motility compared with wild-type, whereas overexpression of plzD had a negative effect on motility (Fig. 3D). However, overexpression of plzDR140A had the same negative effect on motility as overexpression of plzD (data not shown), suggesting that this effect is not dependent on the ability to bind c-di-GMP and that PlzD may have functions independent of c-di-GMP binding. These data further support a role for PlzC as a positive regulator of motility and suggest that PlzD may act as a negative regulator of motility in a c-di-GMP-independent manner. Overexpression of plzA, plzB, and plzE had no effect on motility, and no effects were observed in the vieAE170A background (Fig. 3D; data not shown).
PilZ Proteins PlzB and PlzC Regulate V. cholerae Biofilm Formation
We have previously reported that c-di-GMP regulates biofilm formation in V. cholerae (8). To identify whether PilZ proteins play a role in this process, we measured biofilm formation at the air-liquid interface of standing cultures by crystal violet staining (Fig. 4). A strain with an insertion mutation in vpsR (vpsRpGP704), a positive regulator of vps genes (49), was used as a control. Deletion of plzB or mutation of the PlzB PilZ domain, plzBR96A, reduced biofilm formation compared with wild type. Therefore, PlzB positively regulates biofilm formation in a PilZ-dependent manner.

V. cholerae strains were grown in LB broth standing cultures at room temperature for 16 h, and adherent biofilms were stained with crystal violet. Staining was quantified by solubilizing bound crystal violet in ethanol and measuring the absorbance at 570 nm. The mean and S.D. values from triplicate cultures are shown. wt, AC61; vieAE170A, AC1596; vpsRpGP704, AC1869; ΔplzB, AC2342; plzBR96A, AC2458; ΔplzB (pPlzB) (pAC2140), AC2348; plzBR96A (pPlzB) (pAC2140), AC2459; vieAE170A ΔplzB, AC2342; vieAE170A ΔplzB (pPlzB) (pAC2140), AC2349; ΔplzC, AC2346, vieAE170A ΔplzC, AC2347; vieAE170A ΔplzC (pPlzC) (pAC2141), AC2347; ΔplzD, AC2344; vieAE170A ΔplzD, AC2345.
As has been described previously, the vieAE170A mutant forms a more robust biofilm as a result of increased c-di-GMP concentration (8) (Fig. 4). Deletion of plzB or plzC in the vieAE170A background abrogated this effect, and the mutants formed biofilms similar to wild type. In each case, the complementing plasmid restored biofilm formation to parental strain levels (Fig. 4). A similar result was seen using a ΔplzB or ΔplzC strain overexpressing the DGC VCA0956 (data not shown). Thus, PlzB and PlzC function as positive regulators of biofilm formation when the cellular level of c-di-GMP is elevated.
plzD did not play a role in biofilm formation under the conditions tested, since deletion of the gene had no effect on biofilm formation (Fig. 4). In addition, overexpression of each of the five PilZ domain proteins in either the wild-type or vieAE170A background had no effect on biofilm formation.
PilZ Proteins PlzB, PlzC, and PlzD Are Required for Intestinal Colonization
It was previously shown that control of c-di-GMP concentration by the PDEA VieA in the V. cholerae classical biotype is required for colonization of the infant mouse small intestine (13). Therefore, we investigated whether individual PilZ proteins were required for intestinal colonization. To address this question, competition assays in mice were performed. We found that deletion of plzB or mutation of a conserved PilZ domain residue (R96A) resulted in a 10-fold decrease in colonization (Fig. 5A). These colonization defects were fully complemented by expression of plzB in trans (Fig. 5A).

Competition assays were performed using the infant mouse model of infection. A, strains ΔplzB (AC2342), plzBR96A (AC2458), were competed against wild-type O395 (AC50). Strains ΔplzB (pPlzB (pAC2140)) (AC2348) and plzBR96A (pPlzB (pAC2140)) (AC2459) were competed against O395 (pMMB67EH) (AC1109), which contained the empty vector. B, strains ΔplzC (AC2356), ΔplzD (AC2344), ΔplzC ΔplzD (AC2550), and ΔplzC plzDR140A (AC2553) were competed against wild-type O395 (AC50). Strains ΔplzC ΔplzD (pPlzC (pAC2141)) (AC2551), ΔplzC ΔplzD (pPlzD (pAC2137)) (AC2552), and ΔplzC plzDR140A (pPlzD (pAC2137)) (AC2554) were competed against O395 (pMMB67EH) (AC1109). The competitive index is the ratio of mutant to wild-type bacteria recovered from the small intestine corrected for the input ratio. Each data point represents the competitive index from an individual mouse; the gray bar represents the geometric mean. The ΔplzB, plzBR96A, ΔplzC ΔplzD, and ΔplzC plzDR140A strains are significantly attenuated (p < 0.01) by Student’s two-tailed t test.
Single deletion of either plzC or plzD had no effect on colonization, whereas deletion of both (ΔplzC ΔplzD) led to a 3-fold colonization defect (Fig. 5B). This colonization defect could be complemented by expression of eiher plzC or plzD in trans. Additionally, mutation of residue Arg140 of PlzD, which was shown to be required for c-di-GMP binding (Fig. 2C), in the ΔplzC background resulted in the same 3-fold attenuation as ΔplzC ΔplzD, and this attenutation could be complemented by the addition of plzD in trans (Fig. 5B). Thus, the PilZ domain of PlzD plays a role in the colonization of the infant mouse small intestine.
PlzB, PlzC, and PlzD Are Not Required for c-di-GMP-mediated Regulation of Cholera Toxin Expression
We have previously shown that c-di-GMP regulates CT expression in V. cholerae O395 and that VieA is required for this regulation (13). To determine if PilZ proteins are involved in the c-di-GMP-mediated regulation of CT expression, we measured CT expression by enzyme-linked immunosorbent assay in supernatants from overnight cultures grown under virulence gene-inducing conditions, incubation at 30 °C in M9 + NRES (42). However, CT expression was not altered by deletion of plzB, plzC, or plzD in the wild-type or vieAE170A backgrounds (data not shown). Additionally, overexpression of each PilZ protein in the wild-type or vieAE170A background showed no effect on CT expression under these conditions (data not shown).
DISCUSSION
In this report, we experimentally confirm the hypothesis of Amikam and Galperin (35) by showing that a subset of PilZ proteins from V. cholerae specifically bind c-di-GMP. Additionally, we show that mutation of the PilZ genes plzB, plzC, and plzD results in alterations in cellular processes known to be regulated by c-di-GMP, including motility, biofilm formation, and virulence. Together, these data are consistent with a role for at least some PilZ proteins as c-di-GMP-sensing proteins and regulators of diverse processes in bacteria.
Binding of c-di-GMP by PilZ Proteins
To date, only three PilZ domain proteins have been shown to bind c-di-GMP, including cellulose synthase from G. xylinus, YcgR from E. coli, and PA4608 from P. aeruginosa (3, 39, 40). Here we show that two V. cholerae PilZ proteins (PlzC and PlzD) present in cell extracts, as well as purified, bind c-di-GMP specifically and with submicromolar affinities. Additionally, we show that five highly conserved amino acid residues in the PlzD PilZ domain are required for binding of c-di-GMP. Each of these residues is found in a patch on the same face of the PilZ domain, suggesting that they may act to coordinate c-di-GMP. Thus, it is likely that the PilZ domain functions in c-di-GMP binding, as hypothesized by Amikam and Galperin (35).
Our observation that PlzC but not PlzD was able to bind c-di-GMP after denaturation suggests that either the latter protein cannot refold properly under the conditions used or that it requires dimer- or multimerization in order to bind c-di-GMP, as suggested by the recently solved crystal structure of PlzD (Protein Data Bank entry 1YLN).4 Our inability to show PlzA, PlzB, and PlzE binding to c-di-GMP may be explained by one or more of the following: 1) their binding affinities are low relative to PlzC and PlzD, and thus binding is undetectable by the methods used in this study; 2) these proteins do not bind c-di-GMP; 3) additional cellular components lost during the protein purification are required for their binding to c-di-GMP (i.e. their PilZ domains are not sufficient for binding of c-di-GMP); or 4) these proteins do not fold properly or achieve their correct quaternary structure when overexpressed in and purified from E. coli. We attempted to rule out some of these possibilities by assaying c-di-GMP binding of PilZ proteins in E. coli and V. cholerae lysates; however, we were unable to observe binding by PilZ proteins other than PlzC and PlzD. Further structural and biochemical characterization of PilZ domain proteins may shed light on which of these possibilities is correct. It is worth noting that one of the PilZ proteins for which we did not detect c-di-GMP binding (PlzB) also lacks a number of the conserved residues required for c-di-GMP binding by PlzD (Fig. 2A). Nevertheless, the ΔplzB mutant had the most profound phenotypes observed in this study, and mutation of a conserved PilZ domain residue (Arg96) mirrored the phenotypes of the deletion mutant. Thus, it remains a possibility that this protein binds c-di-GMP in vivo and/or interacts via its PilZ domain with other c-di-GMP regulatory components.
PilZ-mediated Regulation of Motility
Previous results have shown that c-di-GMP acts as a negative regulator of motility in V. cholerae (48). Here we found that motility of ΔplzB, plzBR96A, and ΔplzC mutants was reduced, showing an intermediate phenotype between wild type and vieAE170A. Thus, PlzB and PlzC are positive regulators of motility under growth conditions that result in a low intracellular concentration of c-di-GMP. c-di-GMP has been shown to regulate biofilm and virulence genes at the transcriptional level, so it seems reasonable that motility would be regulated in the same manner by controlling transcription of flagellar biosynthesis genes. The regulatory cascade controlling flagellar biosynthesis in V. cholerae has been well characterized, with multiple proteins having been identified as transcriptional regulators within the hierarchy (50, 51). It is possible that c-di-GMP affects the expression or activity of one of these regulators through PlzB and PlzC. Additionally, we found that overexpression of PlzD resulted in reduced motility in M9 + NRES chemotaxis plates, suggesting that PlzD acts as a negative regulator of motility in this condition. Surprisingly, this regulation was independent of c-di-GMP binding, since overexpression of PlzDR140A gave the same motility defect.
PilZ-mediated Regulation of Biofilm Formation
We have previously shown that c-di-GMP regulates biofilm formation by positively regulating the transcription of vpsR, a positive regulator of vps gene transcription (8, 49). Here we find that deletion of plzB in both wild-type and vieAE170A backgrounds and deletion of plzD in the vieAE170A background results in a decrease in biofilm formation. Also, mutation of the highly conserved PilZ domain residue Arg96 in plzB showed the same decrease in biofilm formation as ΔplzB. These data suggest that PlzB and PlzD act as positive regulators of biofilm formation at both low (PlzB) and high (PlzB and PlzC) c-di-GMP levels.
One contradictory finding of this work is that PlzB and PlzD function as positive regulators of both motility and biofilm formation, whereas c-di-GMP has an inverse effect on these two processes, acting as a negative regulator of motility and a positive regulator of biofilm formation. This suggests that regulation of these processes by c-di-GMP involves additional proteins besides PlzB and PlzD. Further studies are under way to understand how PlzB and PlzD regulate these processes.
Role of PilZ Proteins in Intestinal Colonization
Deletion of plzB and mutation of the conserved PilZ domain residue Arg96 reduced colonization of the infant mouse small intestine by 10-fold. This intestinal colonization defect may be due to the observed reduction in motility. It has previously been shown that deletion of flrA or flrC, two major positive regulators of flagellar biosynthesis, also results in an approximately 10-fold attenuation in colonization (13, 52). However, at this point, we cannot rule out the possibility that other PlzB-regulated factors play a role in virulence.
Deletion of either plzC or plzD individually had no effect on colonization, but deletion of both genes resulted in a 3-fold defect in colonization. This colonization defect was PilZ domain-dependent, since we observed a similar 3-fold colonization defect for a ΔplzC plzDR140A mutant strain. We did not observe changes in any other phenotypes of the double mutant compared with the single deletion strains; therefore, we are unsure of the reason for the observed attenuation. We predict that other processes that play a role in colonization are jointly regulated by PlzC and PlzD in response to c-di-GMP concentration during infection. Finally, our data suggest that PlzD has both c-di-GMP-dependent and -independent functions; PlzDR140A can function similar to the wild-type protein in regulating motility in M9 + NRES chemotaxis plates but does not have wild-type function in the mouse model of infection.
CT Expression Is Not Regulated by PlzB, PlzD, or PlzC
We have previously shown that c-di-GMP regulates CT expression in V. cholerae (13). However, we did not observe changes in CT expression in any of the PilZ mutant strains tested in the present study. Nor did we observe changes in CT expression when PilZ domain proteins were overexpressed. It is possible that PlzB, PlzC, and PlzD perform redundant roles in regulation of CT expression. Also, the PilZ proteins we were unable to delete, PlzA and PlzE, may be involved in regulation of CT. Further study, perhaps involving multiple PilZ gene deletions, is needed to determine what role if any PilZ proteins play in c-di-GMP-mediated regulation of CT.
CONCLUSION
Based on our observations that PlzC and PlzD bind c-di-GMP tightly and specifically via their PilZ domains and that mutation of PlzB, PlzC, and PlzD causes changes in motility, biofilm formation, and virulence in a PilZ domain-dependent manner, it is tempting to speculate that, by analogy to G. xylinus cellulose synthase, unknown output domains of PlzB, PlzC, and PlzD are allosterically regulated by c-di-GMP binding. Such binding may induce structural changes that regulate activity of these hypothetical PlzB, PlzC, and PlzD output domains. Future structural and biochemical studies of PlzB, PlzC, and PlzD may shed light on this possibility.
We have previously proposed a model of c-di-GMP-mediated regulation of V. cholerae biofilm formation, motility, and virulence that includes factors downstream of c-di-GMP that sense changes in intracellular c-di-GMP concentration and relay this signal downstream, leading to changes in gene transcription. Our data suggest that PilZ proteins represent this factor. Additional work to characterize the role of individual PilZ proteins within the c-di-GMP regulatory network is under way and will be needed to elucidate how these proteins mediate c-di-GMP-dependent regulation.
Acknowledgments
We thank Susan Butler for cloning and purification of CheY and John Hunt and Jordi Benach for providing the PlzD monomer figure.
Footnotes
*This work was supported by National Institutes of Health Grant AI45746 (to A. C.) and Center for Gastroenterology Research on Absorptive and Secretory Processes, New England Medical Center, Grant P30DK34928. The costs of publication of this article were defrayed in part by the payment of page charges. This article must therefore be hereby marked “advertisement” in accordance with 18 U.S.C. Section 1734 solely to indicate this fact.
3The abbreviations used are: c-di-GMP, cyclic diguanylate; PDEA, phosphodiesterase A; DGC, diguanylate cyclase; CT, cholera toxin; Cm, chloramphenicol.
4R. Zhang, M. Zhou, S. Moyi, F. Collart, and A. Joachimiak, unpublished data.
REFERENCES
Full text links
Read article at publisher's site: https://doi.org/10.1074/jbc.m611593200
Read article for free, from open access legal sources, via Unpaywall:
http://www.jbc.org/article/S0021925818950623/pdf
Citations & impact
Impact metrics
Article citations
Genome-Wide Computational Prediction and Analysis of Noncoding RNAs in Oleidesulfovibrio alaskensis G20.
Microorganisms, 12(5):960, 10 May 2024
Cited by: 0 articles | PMID: 38792789 | PMCID: PMC11124144
Nucleotides as Bacterial Second Messengers.
Molecules, 28(24):7996, 07 Dec 2023
Cited by: 0 articles | PMID: 38138485 | PMCID: PMC10745434
Review Free full text in Europe PMC
PlzD modifies Vibrio vulnificus foraging behavior and virulence in response to elevated c-di-GMP.
mBio, 14(5):e0153623, 06 Oct 2023
Cited by: 0 articles | PMID: 37800901 | PMCID: PMC10653909
Functional diversity of c-di-GMP receptors in prokaryotic and eukaryotic systems.
Cell Commun Signal, 21(1):259, 25 Sep 2023
Cited by: 1 article | PMID: 37749602 | PMCID: PMC10519070
Review Free full text in Europe PMC
During heat stress in Myxococcus xanthus, the CdbS PilZ domain protein, in concert with two PilZ-DnaK chaperones, perturbs chromosome organization and accelerates cell death.
PLoS Genet, 19(6):e1010819, 20 Jun 2023
Cited by: 1 article | PMID: 37339150 | PMCID: PMC10313047
Go to all (129) article citations
Data
Data behind the article
This data has been text mined from the article, or deposited into data resources.
BioStudies: supplemental material and supporting data
Pfam
- (1 citation) Pfam - PF07238
Similar Articles
To arrive at the top five similar articles we use a word-weighted algorithm to compare words from the Title and Abstract of each citation.
The structural basis of cyclic diguanylate signal transduction by PilZ domains.
EMBO J, 26(24):5153-5166, 22 Nov 2007
Cited by: 140 articles | PMID: 18034161 | PMCID: PMC2140105
Identification and characterization of cyclic diguanylate signaling systems controlling rugosity in Vibrio cholerae.
J Bacteriol, 190(22):7392-7405, 12 Sep 2008
Cited by: 69 articles | PMID: 18790873 | PMCID: PMC2576663
Quorum sensing controls biofilm formation in Vibrio cholerae through modulation of cyclic di-GMP levels and repression of vpsT.
J Bacteriol, 190(7):2527-2536, 25 Jan 2008
Cited by: 270 articles | PMID: 18223081 | PMCID: PMC2293178
The ins and outs of cyclic di-GMP signaling in Vibrio cholerae.
Curr Opin Microbiol, 36:20-29, 05 Feb 2017
Cited by: 80 articles | PMID: 28171809 | PMCID: PMC5534393
Review Free full text in Europe PMC
Funding
Funders who supported this work.
Howard Hughes Medical Institute
NIAID NIH HHS (3)
Grant ID: R01 AI045746
Grant ID: AI45746
Grant ID: R01 AI045746-06A1
NIDDK NIH HHS (2)
Grant ID: P30 DK34928
Grant ID: P30 DK034928