Abstract
Free full text

THE CRITICAL ROLE OF VOLTAGE-DEPENDENT CALCIUM CHANNEL IN AXONAL REPAIR FOLLOWING MECHANICAL TRAUMA
Abstract
Membrane disruption following mechanical injury likely plays a critical role in the pathology of spinal cord trauma. It is known that intracellular calcium is a key factor that is essential to membrane resealing. However, the differential role of calcium influx through the injury site and through voltage dependent calcium channels (VDCC) has not been examined in detail. Using a well established ex vivo guinea pig spinal cord white matter preparation, we have found that axonal membrane resealing was significantly inhibited following transection or compression in the presence of cadmiun, a non-specific calcium channel blocker, or nimodipine, a specific L-type calcium channel blocker. Membrane resealing was assessed by the changes of membrane potential and compound action potential (CAP), and exclusion of horseradish peroxidase 60 minutes following trauma. Furthermore, 1 μM BayK 8644, a VDCC agonist, significantly enhanced membrane resealing. Interestingly, this effect was completely abolished when the concentration of BayK 8644 was increased to 30 μM. These data suggest that VDCC play a critical role in membrane resealing. Further, there is likely an appropriate range of calcium influx through VDCC which ensures effective axonal membrane resealing. Since elevated intracellular calcium has also been linked to axonal deterioration, blockage of VDCC is proposed to be a clinical treatment for various injuries. The knowledge gained in this study will likely help us better understand the role of calcium in various CNS trauma, which is critical for designing new approaches or perhaps optimizing the effectiveness of existing methods in the treatment of CNS trauma.
INTRODUCTION
The most important function of the plasma membrane is to protect the cell and maintain a functional intracellular environment. When the integrity of the neuronal membrane is disrupted, ions and other molecules leak in and out of the cell down their electrochemical gradients, resulting in cell death through various mechanisms (Lucas et al., 1985; Gross and Higgins, 1987; Fitzpatrick et al., 1998; Fiskum, 2000; Lewen et al., 2000; Ray et al., 2003). It is clear that membrane repair following physical insult is critical for survival and functional recovery in neurons, as it is for other cells. In the case of axons, membrane resealing is also required to prevent further degeneration of the severed fibers (Gross et al., 1983; Lucas et al., 1985; Yawo and Kuno, 1985; Shi et al., 1989; Xie and Barrett, 1991; Shi and Borgens, 1999; Shi et al., 2000; Shi and Borgens, 2000). Hence, the earlier the breach is closed, the better the prospects for maintaining the residual functions of the neuron and for potential regrowth and recovery of function through plasticity and regeneration.
Neuronal membrane disruption following mechanical insult has been documented extensively in vitro, ex vivo (Xie and Barrett, 1991; Spira et al., 1993; Krause et al., 1994; Eddleman et al., 1997; Shi et al., 2000; Shi and Borgens, 2000; Shi and Pryor, 2002) and in vivo (Pettus et al., 1994; Maxwell et al., 1999; Shi, 2002). Extended membrane disruption likely plays a key role in cell death, tissue loss, and neurological dysfunction. However, the environment following acute traumatic spinal cord injury in vivo is believed to be hostile for membrane repair (Stokes et al., 1983; Young and Koreh, 1986), and prolonged axonal membrane damage appears to exist following in vivo injury (Shi, 2004). Therefore, it is important to elucidate the mechanisms of membrane repair and to formulate effective pharmaceutical interventions that can promote membrane repair and enhance functional recovery.
It is well established that membrane resealing in mammalian nerve cells is calcium dependent (Xie and Barrett, 1991; Shi et al., 2000). Furthermore, it is believed that calcium influx following injury initiates many biochemical reactions that are critical for repair of damaged membranes (Yawo and Kuno, 1983, 1985; Terasaki et al., 1997). The most obvious avenue of calcium influx following mechanical insults is at the primary site of membrane disruption (Borgens et al., 1980; Strautman et al., 1990; Ziv and Spira, 1993; Krause et al., 1994; Eddleman et al., 2000). However, there is also a possible, and sometimes overlooked, pathway of calcium influx through depolarized voltage dependent calcium channels (VDCC) (Ziv and Spira, 1993; Sattler et al., 1996; Agrawal et al., 2000; Ouardouz et al., 2003; Ouardouz et al., 2005). It is commonly believed that calcium influx through the injury site is sufficient to trigger effective membrane resealing due largely to the assumption that this calcium influx is much greater than through individual calcium channels. However, the role of calcium influx through calcium channels has not been examined in the context of membrane resealing. Considering the fact that calcium channel blockers have been postulated as a potential treatment for traumatic CNS injury (Pointillart et al., 1993; Rhee et al., 1996; Imaizumi et al., 1999; Mason et al., 1999), it is particularly important to determine their role in influencing membrane repair, a critical factor in the recovery of CNS traumatic injury (Shi and Borgens, 1999; Shi et al., 2000; Shi and Borgens, 2000; Shi and Pryor, 2000).
The overall objective of this study was to examine the role of calcium influx through VDCC in axonal membrane resealing following two types of mechanical injuries, transection and compression. Two known calcium channel blockers, nimodipine, a specific L-type calcium channel blocker, and cadmium, a non-specific calcium channel blocker, were used to block calcium influx through Ca2+ channels. Finally, a specific L-type calcium channel agonist, BayK 8644, was used to study the effect of increased calcium channel activity on membrane resealing. This study was carried out using a well-established isolated spinal cord model where the membrane integrity can be assessed both functionally and anatomically (Shi et al., 2000; Shi and Borgens, 2000; Shi and Pryor, 2000; Peasley and Shi, 2002). The findings in this study support the hypothesis that calcium influx through VDCC likely plays an important role in membrane resealing after mechanical spinal cord injury.
EXPERIMENTAL PROCEDURES
The experimental protocols have been reviewed and approved by the Purdue University Animal Care and Use Committee (PACUC). All experiments were performed ex vivo on strips of spinal cord white matter, isolated from adult, female, Hartley guinea pigs weighing 300–500g. Histological studies were performed on the same tissue that was used for physiological analysis.
Isolation of spinal cord
Guinea pigs were anesthetized with a combination of ketamine (80 mg/kg) and xylazine (12 mg/kg), and were perfused through the heart with 500 mL oxygenated, cold Krebs’ (15°C) solution to remove blood and lower cord temperature. The spinal cord was removed from the vertebral column and was immediately subdivided, first along the sagittal midline, and then radially, to produce strips of ventral white matter. The strips were then incubated in fresh Krebs’ solution for one hour and continuously bubbled with 95% O2, 5% CO2 before experimentation. The composition of the Krebs’ solution was as follows (in mM): 124 NaCl, 2 KCl, 1.2 KH2PO4, 1.3 MgSO4, 1.2 CaCl2, 10 dextrose, 5.6 sodium ascorbate, and 26 NaHCO3. The pH of Krebs’ solution was 7.2–7.4.
Recording chamber
Variations of the double sucrose gap recording chamber have been described. Further details can be found in our previous publications (Shi and Blight, 1996; Shi and Borgens, 1999; Shi et al., 2000; Shi and Pryor, 2002). Figure 1 shows a diagram of the recording chamber which consists of two outer wells containing isotonic potassium chloride (120 mM), and a center well containing oxygenated Krebs solution, separated by two middle gaps containing isotonic sucrose solution (320 mM). A strip of isolated spinal cord white matter, approximately 35 mm in length, was supported in the central compartment and continuously supplied with oxygenated Krebs’ solution (~2 mL/min). The ends of the tissue were drawn through the sucrose gap channels and ended in side compartments filled with isotonic potassium chloride (Fig. 1). The white matter strip was sealed on either side of the sucrose gap channels, using fragments of plastic cover-slip and a small amount of silicone grease to prevent any mixing of the solutions. Isotonic sucrose solution was continuously run through the gap channels at a rate of 1 mL/min. The temperature of the chamber was maintained at 37°C with a thermostatically controlled inline heater (Warner Instruments). Axons were stimulated and compound action potentials were recorded at opposite ends of the white matter with silver-silver chloride electrodes. The central bath was connected to an instrument ground. Stimuli approximately 0.1 ms in duration were delivered in the form of constant-current unipolar pulses through a stimulus isolation unit. Recordings were made with silver/silver chloride wire electrodes positioned within the side chambers and the central bath using a bridge amplifier. Subsequent analysis was performed using custom Labview® software (National Instruments™) on a Compaq PC™.
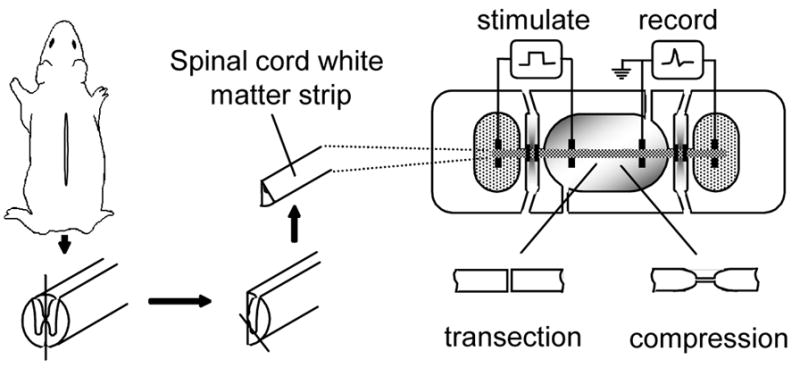
Diagrams showing the method for guinea pig spinal cord white matter isolation (left) recording apparatus and injury technique (right). Once the spinal cord was removed from the vertebral column it was cut longitudinally down the center axis and then subdivided again to extract only ventral white matter strips. The isolated spinal cord tract is shown mounted in the middle of the center well, which is continuously perfused with oxygenated Krebs’ solution. The two end chambers containing the ends of the spinal cord segment contained isotonic KCl. These chambers were divided from the center chamber by two thinner gaps filled with flowing, isotonic sucrose solution. Stimuli were presented at the left with axonal activity recorded at the right. The two injury models used were a transection model in which the entire white matter strip was transected using micro scissors, or a crush model in which the white matter strips were injured using a pair of modified forceps.
Perfusion of Drugs
Cadmium (100 μM), Nimodipine (10 μM), and BayK 8644 (1 and 30 μM) were introduced through the same perfusion mechanism as the Krebs solution. Once the membrane and action potential had stabilized, the drug was perfused along with Krebs’ solution. The drug was allowed to run for at least 15–20 minutes to ensure complete perfusion before the transection and compression injury. This also allowed for the reestablishment of a stable base line due to transient disturbance as a result of drug perfusion.
Transection and compression injury
Transection injuries were produced by cutting the tissue strip at the face of the recording sucrose gap, using a micro-scissors (Shi et al., 2000; Shi and Pryor, 2000). Crush injuries were performed at the face of the recording sucrose gap using blunt forceps with a spacer placed between the tips. The severity of the crush injury was controlled by varying the width of the spacer so compression could be applied accordingly (Borgens and Shi, 2000; Luo et al., 2002). In the present study, the first group of crush injury (Fig. 4) was designed so the recovery of CAP was at approximately 45% of pre-crush levels determined at 60 min. post injury. The second group of crush injury (Fig. 6) was designed so the CAP recovery was approximately 10% of pre-crush levels examined at 60 min post injury.
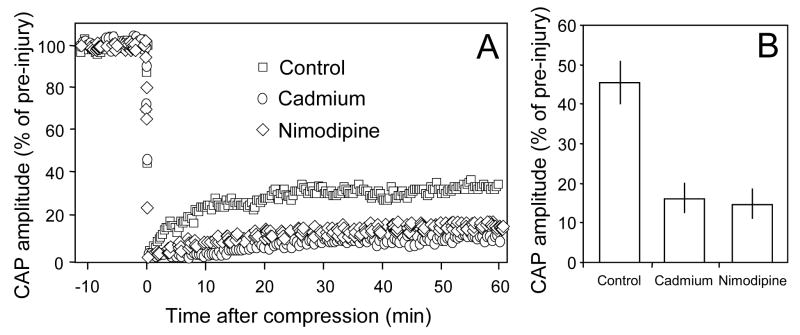
The response of the compound action potential amplitude to compression injury in 3 groups of spinal cord strips. A: Examples of CAP changes in response to compression injury in control conditions or in the presence of cadmium or nimodipine. The compound action potential was extinguished initially after the crush injury, but recovered and reached a new plateau level. Note CAP recovery was noticeably less when cadmium or nimodipine was present compared to control conditions. B: Average CAP recovery after injury in control and cadmium or nimodipine treated injury groups. The recovery plateau amplitude was approximately 2 ½ times greater in the control solution (45.61±5.18% of pre-injury, n=9) compared to the solution containing cadmium (16.87±3.69% of pre-injury, n=9) or nimodipine treated group (14.48±5.28%, n=4). The recovered CAP amplitude in both cadmium and nimodipine treated groups are significantly lowered that that in control (P<0.01, ANOVA). However, there is no significant difference between cadmium and nimodipine treated group (P>0.05, ANOVA).
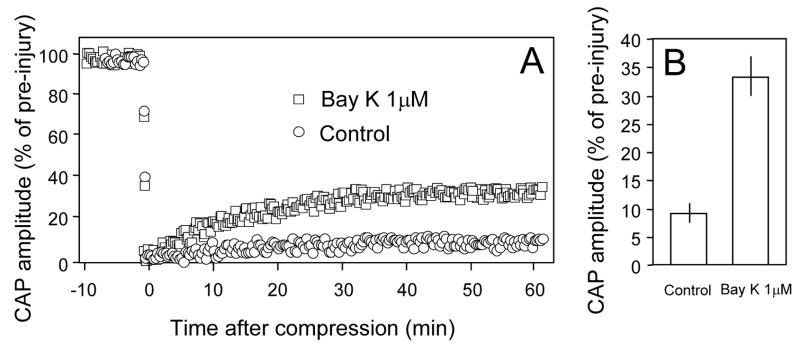
The response of the compound action potential amplitude to compression injury in 2 groups of spinal cord strips. A: Individual examples of CAP changes in response to compression injury in control conditions or in the presence of BayK 8644 (1 μM). The compound action potential was almost extinguished after the crush injury, but recovered and reached a new plateau level. Note CAP recovery was noticeably higher when BayK 8644 (1 μM) was present compared to control conditions (2 mM Ca2+ and 37°C). B: Average CAP recovery after injury in control and BayK 8644 treated injury groups. The recovery plateau amplitude was approximately 3 times greater in the compression injury bathed in the Krebs’ solution containing BayK 8644 (1 μM) (9 ± 1.67% of pre, N=8) than in the control solution (33.25 ± 3.41% of pre, N=8). (P<0.001).
HRP histochemistry
At the conclusion of electrophysiological recording (~60 min post injury), tissue samples containing the cut end or crush site were processed for HRP histochemistry based on methods described previously (Shi and Blight, 1996; Shi and Borgens, 1999; Shi et al., 2000; Shi and Pryor, 2002). Specifically, white matter strips were transferred to oxygenated Krebs’ solution containing 0.015% HRP (Sigma type VI). After incubation for 1 hour at room temperature, the tissue was fixed by immersion in 2.5% glutaraldehyde in phosphate buffer for 1 hour. Transverse sections of the tissue were cut at 30 μm on a Vibratome (Electron Microscopy Science) and stained with a diaminobenzidine reaction to reveal the extent of HRP uptake into damaged axons. Images of the sections were examined and photographed with a Leitz Orthoplan® microscope and a Nikon video camera (Shi and Borgens, 2000; Shi et al., 2001).
2-D Morphology
This method of analysis has previously been described (Shi and Borgens, 2000; Shi et al., 2001). Briefly, the images from the HRP stained sections were digitized and captured to a Macintosh computer using a Leitz Ortoplan microscope and JVC video camera. Cross sections were selected using a 6.3X objective. Representative area samples were taken from peak regions of HRP uptake to quantify axonal resealing. The area was selected in such a way that could be divided into 3 squares from the pia to the gray matter: lateral, intermediate, and medial. The size of the region depended on the thickness of the white matter. Using a 16X-objective, the number of unsealed (HRP-labeled) axons were counted, normalized per unit area, and expressed as a density (axons/mm2).
Statistical treatment
One-way unpaired ANOVA was used to conduct multiple comparisons followed by poct-hoc Tukey test to evaluate the significance between each of the individual groups. Unpaired Student’s t-test was used in all other analyses where a single comparison between two groups was made. Statistical significance was attributed to values P<0.05. Averages were expressed as mean ± standard error of mean (SEM).
RESULTS
Cadmium and nimodipine inhibit membrane repair following transection
It is known that transected guinea pig spinal cord axons are capable of resealing, both functionally and anatomically, within 60 minutes of injury (Shi et al., 2000). Successful resealing of the disrupted membrane could be achieved with normal extracellular calcium concentrations (1–2 mM), and normal physiological temperature (37°C) (Shi et al., 2000; Shi and Pryor, 2000). However, we have found that when calcium channel blockers, cadmium or nimodipine were introduced to the solution, recovery of resting membrane potential following transection (expressed as % pre-transection value) is significantly inhibited (Fig. 2). Specifically, in control (transection only) conditions (2 mM Ca2+, 37°C), the average membrane depolarization at 60 minutes post-injury was 5.6 ± 1.5% (N = 8). In the presence of cadmium, however, the membrane depolarization was 33.1 ± 5.0% (N = 8) at the same time point. This value is significantly higher than the transection only group (P < 0.001, ANOVA). Similarly, the application of nimodipine also significantly increased the membrane depolarization to 43 ± 5.0% at the same time point post injury (P < 0.001 when compared to control, ANOVA). However, there is no significant difference between the cadmium and nimodipine treated groups (P>0.05, ANOVA). Application of cadmium or nimodipine for up to 80 minutes did not induce significant membrane depolarization or reduction of CAP amplitude in control axons (data not shown).
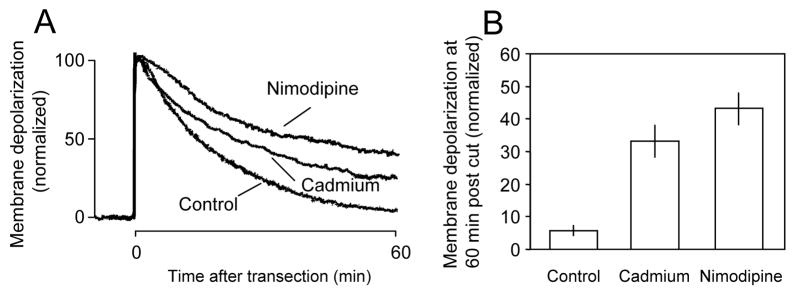
Individual examples (A) and averaged membrane depolarization potential recordings (B) in response to transection under different calcium blocking conditions. A: There was a significant decrease in the rate of recovery of membrane repolarization when cadmium (100 μm) was added to the Krebs’ solution. Membrane repolarization was inhibited further when the specific L-type calcium channel blocker nimodipine (10 μm) was added to the Krebs’ solution. B: Average membrane depolarization at 60 minutes post injury. There was a significant increase in membrane depolarization when cadmium (33.13±5.04%, N=8) or nimodipine (43.13±4.8%, N=8) was added to the perfusion solution compared to Krebs’ alone (5.63±1.52%, n=8) (P < 0.001 when compared to control in both treatment groups, ANOVA). However, there is no significant difference between cadmium and nimodipine treated group (P>0.05, ANOVA).
In addition to monitoring membrane potential after axonal transection, we also examined the status of membrane integrity using a previously described HRP-exclusion assay (Shi et al., 2000; Shi and Borgens, 2000; Shi et al., 2001). The entry of HRP has been used to indicate the loss of membrane integrity in both in vitro (or ex vivo) and in vivo studies (Pettus et al., 1994; Shi et al., 2000; Shi and Borgens, 2000; Shi et al., 2001; Shi, 2004). Consistent with the membrane recovery profile, both cadmium and nimodipine significantly increased the number of axons labeled with HRP examined at 60 minutes post-injury (Fig. 3). Specifically, the density of axons labeled with HRP in control conditions was 34 ± 8.3 axons/mm2 when examined 60 minutes post-transection. The presence of cadmium increased this value to 758.4 ± 83.9 axons/mm2 while the application of nimodipine increased HRP density to 1153.8 ± 203.3 axons/mm2 (P < 0.01 when compared to control in both treatment groups, ANOVA). However, there is no statistical difference between cadmium and nimodipine treated group (P>0.05, ANOVA).
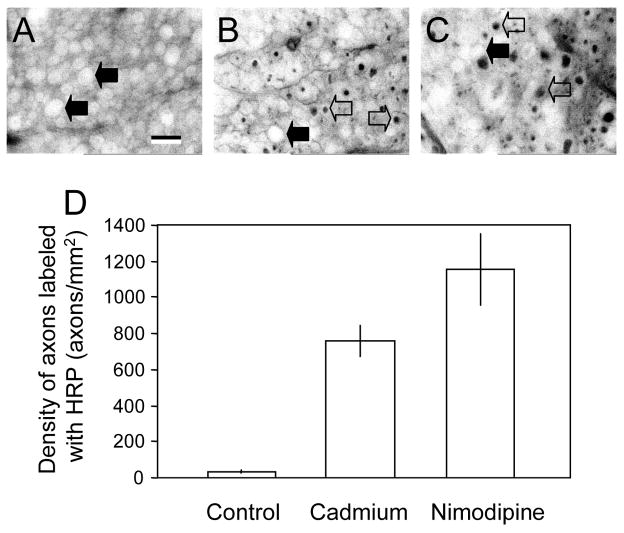
Micrographs of vibratome sections showing HRP labeling (A, B, C) and the average density of labeled axons under different conditions (D). A: Micrographs of spinal cord strips stained for HRP at 60 minutes after transection in control conditions (2mM Ca2+ in Krebs’ solution, 37°C). B and C: Spinal cord strips stained with HRP at 60 minutes following transection and treated with either the general calcium channel blocker cadmium (B) or the L-type specific calcium channel blocker nimodipine (C). Notice the appearance of HRP-labeled axons in both conditions indicated by the open arrows while solid arrows indicate axons which have excluded HRP stain. D: Bar graph showing the quantification of HRP-labeled axons in different conditions. Note that there is a significant increase in the density of axons stained with HRP in axons treated with cadmium (758.4±83.92 axons/mm2, N=5) as well as nimodipine (1153±203.31 axons/mm2, n=5) compared to control conditions (34±8.32 axons/mm2, N=5). (P<0.01 when cadmium treated groups were compared to control, P<0.001 when nimodipine treated groups were compared to control, ANOVA). However, there is no statistical difference between cadmium and nimodipine treated group (P>0.05, ANOVA). Scale bar indicates a distance of 10 μm.
Cadmium and nimodipine inhibit CAP recovery following compression
In this study, the spinal cord strip was compressed so that the average CAP recovery in the control condition was near 45% of pre-compression value determined 60 minutes post-injury (see methods). This injury severity was chosen to compare the post-injury CAP conduction recovery in control (compression only) to those treated with cadmium. Specifically, the compression resulted in complete loss of CAP conduction immediately after injury. Subsequently, the CAP amplitude gradually recovered and plateaued 60 minutes post-compression to an average of 45.6 ± 5.2% of pre-injury levels. When cadmium was introduced in the perfusion medium, however, the CAP amplitude recovery at 60 minutes after injury was reduced to 16.87 ± 3.69% of pre-injury levels, which is significantly lower than control (P < 0.01, ANOVA, Fig. 4B). Similarly, in the presence of nimodipine, the CAP amplitude recovery at 60 minutes after injury was also reduced to 14.48 ± 5.28% of pre-injury levels, which is significantly lower than control (P < 0.01, ANOVA, Fig. 4B). However, there is no significant difference between cadmium and nimodipine treated group (P>0.05, ANOVA).
Calcium channel agonist influences membrane resealing and CAP recovery
In order to examine whether enhanced calcium influx through VDCC promotes membrane resealing, we used BayK 8644, a calcium agonist that is known to promote calcium influx through VDCC. We have found that, at a concentration of 1 μM, BayK 8644 accelerates membrane resealing following transection compared to control (transection only) (Fig. 5). Specifically, the time required for the membrane to recover 95% of the pre-injury resting membrane potential was significantly less in the presence of BayK 8644 (1 μM) (43.1 ± 1.62 minutes) than control (55.4 ± 1.18 minutes, P < 0.001 student’s unpaired, two tail t-test). The time required for 50% repolarization was similar in both control (14.9 ± 0.53 minutes) and BayK 8644 (1 μM) treated groups (15.1 ± 1.05 minutes, P > 0.05, n=7 in both groups, student’s unpaired, two tail t-test).
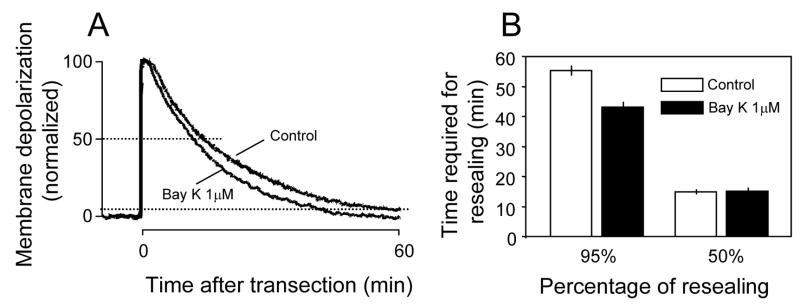
Individual examples and averaged quantification of membrane potential recordings in response to transection injury in 2 different conditions. A: Individual examples of membrane potential recordings in response to transection injury. There was an obvious acceleration of membrane depolarization when BayK 8644 (1 μM) was added to the Krebs’ solution compared to the control condition. B: Examples of averaged quantification of membrane potential recordings in response to transection injury. The time it took for the membrane to reach 95% repolarization was significantly less in BayK 8644 (1 μM) treated groups compared to control rates (P<0.005, N=7 for both control and BayK8644 treated groups). Note that the time required for 50% of membrane repolarization was similar in both control and BayK 8644 (1 μM) treated (P>0.05).
Similarly, BayK 8644 at 1 μM also enhanced CAP recovery following compression. In this case, a more severe compression injury was chosen so that the average CAP recovery was approximately 10% of pre-crush levels (see method). Specifically, the CAP amplitude recovered to 9 ± 1.67% of pre-injury levels at 60 minutes after compression in the control (injury only) group. When BayK 8644 1 μM was introduced to the perfusion solution, the CAP amplitude at 60 minutes post compression was 33.25 ± 3.41% of pre-injury levels, which is significantly higher than control groups (P < 0.001, student’s unpaired, two tail t-test, Fig. 6).
The next group of experiments were designed to examine the effect of high level BayK 8644 (30 μM) on membrane resealing following transection. When BayK 8644 (30 μM) was added to the solution the resultant time required for 50% of membrane repolarization (19.73 ± 2.42 minutes) did not significantly differ from control (transection only, 14.9 ± 0.53 minutes) or the group incubated with 1 μM BayK 8644 (P > 0.05, student’s unpaired, two tail t-test). However, the time it took for the membrane to repolarize 95% in the BayK 8644 (30μM) treated group (59.8 ± 8.32 min) was significantly longer than the group treated with 1 μM BayK 8644 (43.1 ± 1.62 minutes, P < 0.05, student’s unpaired, two tail t-test, Fig. 7A). Interestingly, there is no significant difference of the time required for 95% repolarization in the group with BayK 8644 30μM and control (transection only) (p > 0.05, student’s unpaired, two tail t-test, Fig. 7A). This indicates that the accelerated membrane resealing in the presence of BayK 1 μM was largely eliminated when the concentration of BayK 8644 was elevated to 30 μM.
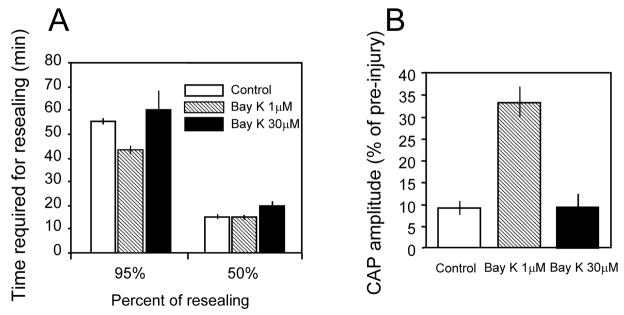
Averaged membrane potential recordings and average CAP recovery in response to transection (A) and crush injury (B) in control and in the presence of either 1 μm or 30 μm Bay K 8644. A: Data with Bay K 8644 1 μM was included here for ease of comparison. The time required for the membrane to repolarize to 50% was similar between the three groups. The time it took for the membrane to repolarize to 95% of pre injury levels was not significantly different between control conditions (55.4 ± 1.18min, N=9) and BayK 8644 (30 μM) treated groups (59.8 ± 8.2min, N=9). However, the repolarization rate was significantly faster when BayK 8644 1 μM was used compared to the other 2 groups. Note that the time required for 95% membrane resealing was similar to the time it took for control axons to reseal when BayK 8644 was increased from 1 μM to 30 μM. B: Average CAP recovery 60 minutes post crush injury was relatively equal when bathed in either the control Krebs’ solution (9 ± 1.67% of pre, N=5) or the BayK 8644 (30 μM) treated solution (9.53 ± 3.01% of pre, N=9, P>0.05). Note that the CAP recovery was reduced back to control levels when BayK 8644 was increased from 1 μM to 30 μM.
A similar result was also seen in compression injuries. Specifically, the average CAP recovery at 60 minutes post crush was 9 ± 1.67% of pre-crush, which was increased to 33.25 ± 3.41% when 1 μM BayK 8644 was added to the perfusion (Fig. 6, ,7A).7A). However, this enhanced recovery of CAP was largely eliminated when BayK 8644 was increased to 30 μM (9.53 ± 3.01%, Fig. 7B). Therefore, even though BayK at 1 μM significantly enhanced CAP recovery, there was a reversal of CAP recovery to near control levels when BayK 8644 was increased from 1μM to 30μM.
DISCUSSION
The current study examines the role of VDCC in membrane resealing by blocking (nimodipine and cadmium) or enhancing (BayK 8644) VDCC in the context of axolemmal resealing following trauma. We have found that blocking VDCC significantly suppressed membrane resealing while moderate enhancement of VDCC was associated with significant acceleration of membrane resealing. These findings seem to suggest an important role of VDCC-mediated calcium influx in axonal resealing. We believe that this is the first observation to suggest that VDCC may play an important role in membrane resealing following traumatic injury.
We have found that there is no significant difference between nimodipine (a specific L-type channel blocker) and cadmium (non-specific calcium channel blocker) treated groups in membrane potential, action potential, or HRP labeling evaluation following trauma. Furthermore, membrane resealing is enhanced by 1 μM BayK 8644, an L-type calcium channel agonist. This suggests that L-type channels plays a significant role in mediating calcium influx and promoting membrane resealing while other types of calcium channels play a minor, if any role in this regard. One piece of evidence consistent with our data is that blocking L- type calcium channels has been shown to inhibit the regeneration of injured axons after transection injury (Kulbatski et al., 2004). One possible explanation is that the axons which received a calcium channel blocker failed to reseal the cut end properly and therefore show delayed or reduced regeneration.
Currently, it is not clear how the calcium influx through VDCC would contribute to the membrane resealing. One possibility is that this may relate to vesicle transport, a calcium-dependent process that is involved in membrane resealing (Steinhardt et al., 1994; Eddleman et al., 1997; Terasaki et al., 1997; Detrait et al., 2000; McNeil and Terasaki, 2001; McNeil and Steinhardt, 2003). It is possible that, in order for successful membrane resealing to occur, a critical number of vesicles must be produced and placed at the injury site. Membrane vesicles are likely formed not just near the focus of the disruption, but also at some distance along the axolemmal and subsequently are transported to the injury site. Indeed, it is well established vesicles and other cellular materials that are transported down the axon by fast and slow axonal transport are produced continuously at the soma, and in the case of axonal disruption, tend to accumulate at the site of blockage (Povlishock, 1992). It is likely that calcium influx through the injury site does not flow very far along the axon due to intrinsic cellular ability to impede diffusion of calcium (Ziv and Spira, 1993; Terasaki et al., 1997). Therefore, it is possible that calcium influx through VDCC ensures adequate calcium influx along the entire length of the damaged axon in response to axonal injury. Subsequently, the elevated calcium level along the axons will ensure the efficient transport of vesicles to the site of membrane disruption. One piece of evidence that supports this hypothesis is that calcium ionophores can enhance the overall number of vesicles near the membrane and induce considerable surface enlargement, both of which are known to enhance membrane resealing (Coorssen et al., 1996; Rodriguez et al., 1997; Cocucci et al., 2004).
Despite the importance of calcium influx in the axonal membrane resealing, it is understandable that excessive accumulation of intracellular calcium could be harmful. It is well established that uncontrolled high levels of calcium can trigger axonal degeneration (Schlaepfer and Bunge, 1973; Schlaepfer, 1974; Schanne et al., 1979; George et al., 1995; Ueda et al., 1997; Cano-Abad et al., 2001). This may explain why the enhanced axonal resealing in the presence of BayK 8644 at 1 μM was largely overcome when the concentration was increased to 30 μM. Interestingly, the biphasic effect of calcium influx through VDCC was also seen in a study done by Kulbatski et al. (Kulbatski et al., 2004). In their study, while 1 μM BayK8644 aided in neurite regeneration, this beneficial effect was largely eliminated or reversed when higher concentrations (up to 30 μM) were used.
Even though calcium influx through VDCC appears to be important for resealing, it does not mean that this will be beneficial in other types of injury. The overall effect of calcium influx through VDCC probably depends on whether mechanical membrane disruption is the primary cause of the injury. For example, unlike mechanical injury where membrane disruption is the key initial pathology, ischemic injury stems from the lack of oxygen and other life-supporting chemicals with little membrane disruption initially (Shi et al., 2000; Shi and Borgens, 2000; Shi and Pryor, 2000; Peasley and Shi, 2002). As a result, the axons depolarize slowly which causes prolonged calcium influx and degradation along an extensive length of axon from which no amount of resealing can protect them. Therefore, the net effect of calcium influx in ischemic insult is harmful which can elicit many pathological process leading to cell death. This may explain why calcium channel blockers are beneficial in ischemic injury (Fern et al., 1995; George et al., 1995; Sattler et al., 1996; Imaizumi et al., 1999; Agrawal et al., 2000), but not in transection injury where the membrane disruption is the primary injury mechanism and membrane resealing is the key to stop uncontrolled influx of calcium (Gross et al., 1983; Lucas et al., 1985; Yawo and Kuno, 1985; Shi et al., 1989; Xie and Barrett, 1991; Shi and Borgens, 1999; Shi et al., 2000; Shi and Borgens, 2000).
Another factor that supports the beneficial effects of Ca2+ following mechanical injuries is that intracellular Ca2+ activity is actually depressed following physical insults. For example, in traumatic injury, degradative processes are set in motion to a greater or lesser degree which result in binding most intracellular Ca2+. As elaborated by Young (Young, 1992) phospholipids are degraded after injury and bind to Ca2+, making intracellular Ca2+ very low. This could explain why adequate Ca2+ (sufficient to promote vesicle fusion with axon membranes but not so high as to exacerbate the degradative processes) is beneficial.
One complication of the current study regarding cadmium-induced impairment in membrane repair is the possible toxicity of cadmium in CNS axons as demonstrated by Fern and his colleagues (Fern et al., 1996). However, we believe this is unlikely. The main reason is that the application of cadmium for 80 minutes did not show any significant functional impairment in uninjured guinea pig spinal cord white matter (current study). In addition, the dosage of cadmium used in this study was only half of what was demonstrated to be harmful to rat optic nerve (Fern et al., 1996). Interestingly, cadmium at a dosage that is 60% of that used in the current study and applied at a duration that is 20 minutes longer than that in this study did not cause significant reduction of CAP amplitude in rat optic nerve (Fern et al., 1996). Therefore, it is more than likely that the effect of cadmium in this study was mainly due to its ability to block VDCC, and not the non-specific toxicity.
It is well documented that prolonged increases in intracellular calcium lead to neuronal degeneration in trauma and diseases (Schlaepfer and Bunge, 1973; Schlaepfer, 1974; Schanne et al., 1979; George et al., 1995; Ueda et al., 1997; Cano-Abad et al., 2001). Because of this, it has been a continuous effort to design therapies for spinal cord injury and traumatic brain injury that either block calcium from entering the cell, or work to maintain a low level of calcium at the injury site. Our study, along with many others, offers a balancing view regarding the role of calcium in overall response to mechanical trauma. It argues a temporal and spatial differential role of calcium in the cellular response to mechanical trauma. More specifically, calcium may be beneficial, or even crucial for membrane resealing following mechanical insults.
Based on our previous studies, it takes a minimum of 30–60 minutes to complete the resealing process in guinea pig spinal cord axons following trauma in vitro (in 2 mM extracellular Ca2+) (Shi et al., 2000). However, considering the fact that extracellular Ca2+ drops below the level that supports effective membrane resealing for several hours following spinal cord trauma (Young et al., 1982; Stokes et al., 1983), it may be considerably longer than 60 minutes under in vivo conditions for effective membrane resealing to occur. Therefore, we suggest that treatments designed to lower extracellular calcium concentrations or aimed to block calcium entry into the cell may be better suited if used an hour post injury, when the membrane integrity is presumably reestablished. This will avoid interfering with the resealing process while preventing prolonged elevation of cytosolic calcium that is known to be detrimental (Schlaepfer and Bunge, 1973; Schlaepfer, 1974; Schanne et al., 1979; George et al., 1995; Ueda et al., 1997; Cano-Abad et al., 2001). It is our desire in the current study to first stress the notion of the differential role of calcium in the recovery process and second, point out that it is in the period immediately following the insult that intracellular calcium is most critical. This knowledge may be important in designing new approaches or optimizing the effectiveness of existing methods in the treatment of CNS trauma.
Acknowledgments
We thank Phyllis Zickmund and Gary Leung for their invaluable assistance during the course of this project. We also thank Kristin Hamann and Jennifer McBride for their critical reading of the manuscript. This study was supported by funding from National Institute of Health and the State of Indiana.
Comprehensive list of abbreviations
- VDCC
- voltage dependent calcium channels
- CNS
- central nervous system
- CAP
- compound action potential
Footnotes
Publisher's Disclaimer: This is a PDF file of an unedited manuscript that has been accepted for publication. As a service to our customers we are providing this early version of the manuscript. The manuscript will undergo copyediting, typesetting, and review of the resulting proof before it is published in its final citable form. Please note that during the production process errors may be discovered which could affect the content, and all legal disclaimers that apply to the journal pertain.
References
- Agrawal SK, Nashmi R, Fehlings MG. Role of L- and N-type calcium channels in the pathophysiology of traumatic spinal cord white matter injury. Neuroscience. 2000;99:179–188. [Abstract] [Google Scholar]
- Borgens RB, Shi R. Immediate recovery from spinal cord injury through molecular repair of nerve membranes with polyethylene glycol. FASEB J. 2000;14:27–35. [Abstract] [Google Scholar]
- Borgens RB, Jaffe LF, Cohen MJ. Large and persistent electrical currents enter the transected lamprey spinal cord. Proc Natl Acad Sci USA. 1980;77:1209–1213. [Europe PMC free article] [Abstract] [Google Scholar]
- Cano-Abad MF, Villarroya M, Garcia AG, Gabilan NH, Lopez MG. Calcium entry through L-type calcium channels causes mitochondrial disruption and chromaffin cell death. J Biol Chem. 2001;276:39695–39704. [Abstract] [Google Scholar]
- Cocucci E, Racchetti G, Podini P, Rupnik M, Meldolesi J. Enlargeosome, an exocytic vesicle resistant to nonionic detergents, undergoes endocytosis via a nonacidic route. Mol Biol Cell. 2004;15:5356–5368. [Europe PMC free article] [Abstract] [Google Scholar]
- Coorssen JR, Schmitt H, Almers W. Ca2+ triggers massive exocytosis in Chinese hamster ovary cells. Embo J. 1996;15:3787–3791. [Europe PMC free article] [Abstract] [Google Scholar]
- Detrait E, Eddleman CS, Yoo S, Fukuda M, Nguyen MP, Bittner GD, Fishman HM. Axolemmal repair requires proteins that mediate synaptic vesicle fusion. J Neurobiol. 2000;44:382–391. [Abstract] [Google Scholar]
- Eddleman CS, Bittner GD, Fishman HM. Barrier permeability at cut axonal ends progressively decreases until an ionic seal is formed. Biophys J. 2000;79:1883–1890. [Europe PMC free article] [Abstract] [Google Scholar]
- Eddleman CS, Ballinger ML, Smyers ME, Godell CM, Fishman HM, Bittner GD. Repair of plasmalemmal lesions by vesicles. Proc Natl Acad Sci USA. 1997;94:4745–4750. [Europe PMC free article] [Abstract] [Google Scholar]
- Fern R, Waxman SG, Ransom BR. Voltage-gated calcium channels in CNS white matter: Role in anoxic injury. J Neurophysiol. 1995;74:369–377. [Abstract] [Google Scholar]
- Fern R, Black JA, Ransom BR, Waxman SG. Cd(2+)-induced injury in CNS white matter. J Neurophysiol. 1996;76:3264–3273. [Abstract] [Google Scholar]
- Fiskum G. Mitochondrial participation in ischemic and traumatic neural cell death. J Neurotrauma. 2000;17:843–855. [Abstract] [Google Scholar]
- Fitzpatrick MO, Maxwell WL, Graham DI. The role of the axolemma in the initiation of traumatically induced axonal injury [editorial] J Neurol Neurosurg Psychiatry. 1998;64:285–287. [Europe PMC free article] [Abstract] [Google Scholar]
- George EB, Glass JD, Griffin JW. Axotomy-induced axonal degeneration is mediated by calcium influx through ion-specific channels. J Neurosci. 1995;15:6445–6452. [Europe PMC free article] [Abstract] [Google Scholar]
- Gross GW, Higgins ML. Cytoplasmic damage gradients in dendrites after transection lesion. Exp Brain res. 1987;67:52–61. [Abstract] [Google Scholar]
- Gross GW, Lucas JH, Higgins ML. Laser microbeam surgery: ultrastructural changes associated with neurite transection in culture. J Neurosci. 1983;3:1979–1993. [Europe PMC free article] [Abstract] [Google Scholar]
- Imaizumi T, Kocsis JD, Waxman SG. The role of voltage-gated Ca2+ channels in anoxic injury of spinal cord white matter. Brain Res. 1999;817:84–92. [Abstract] [Google Scholar]
- Krause TL, Fishman HM, Ballinger ML, Bittner GD. Extent and mechanism of sealing in transected giant axons of squid and earthworms. J Neurosci. 1994;14:6638–6651. [Europe PMC free article] [Abstract] [Google Scholar]
- Kulbatski I, Cook DJ, Tator CH. Calcium entry through L-type calcium channels is essential for neurite regeneration in cultured sympathetic neurons. J Neurotrauma. 2004;21:357–374. [Abstract] [Google Scholar]
- Lewen A, Matz P, Chan PH. Free radical pathways in CNS injury. J Neurotrauma. 2000;17:871–890. [Abstract] [Google Scholar]
- Lucas JH, Gross GW, Emery DG, Gardner CR. Neuronal survival or death after dendrite transection close to the perikaryon: correlation with electrophysiologic, morphologic, and ultrastructural changes. Cent Nerv Syst Trauma. 1985;2:231–255. [Abstract] [Google Scholar]
- Luo J, Li N, Robinson JP, Shi R. The increase of reactive oxygen species and their inhibition in an isolated guinea pig spinal cord compression model. Spinal Cord. 2002;40:656–665. [Abstract] [Google Scholar]
- Mason RP, Leeds PR, Jacob RF, Hough CJ, Zhang KG, Mason PE, Chuang DM. Inhibition of excessive neuronal apoptosis by the calcium antagonist amlodipine and antioxidants in cerebellar granule cells. J Neurochem. 1999;72:1448–1456. [Abstract] [Google Scholar]
- Maxwell WL, Kosanlavit R, McCreath BJ, Reid O, Graham DI. Freeze-fracture and cytochemical evidence for structural and functional alteration in the axolemma and myelin sheath of adult guinea pig optic nerve fibers after stretch injury. J Neurotrauma. 1999;16:273–284. [Abstract] [Google Scholar]
- McNeil PL, Terasaki M. Coping with the inevitable: how cells repair ea torn surface membrane. Nature Cell Biol. 2001;3:124–129. [Abstract] [Google Scholar]
- McNeil PL, Steinhardt RA. Plasma membrane disruption: repair, prevention, adaptation. Annu Rev Cell Dev Biol. 2003;19:697–731. [Abstract] [Google Scholar]
- Ouardouz M, Zamponi GW, Barr W, Kiedrowski L, Stys PK. Protection of ischemic rat spinal cord white matter: Dual action of KB-R7943 on Na+/Ca2+ exchange and L-type Ca2+ channels. Neuropharmacology. 2005;48:566–575. [Abstract] [Google Scholar]
- Ouardouz M, Nikolaeva MA, Coderre E, Zamponi GW, McRory JE, Trapp BD, Yin X, Wang W, Woulfe J, Stys PK. Depolarization-induced Ca2+ release in ischemic spinal cord white matter involves L-type Ca2+ channel activation of ryanodine receptors. Neuron. 2003;40:53–63. [Abstract] [Google Scholar]
- Peasley MA, Shi R. Resistance of isolated mammalian spinal cord white matter to oxygen-glucose deprivation. Am J Physiol Cell Physiol. 2002;283:C980–C989. [Abstract] [Google Scholar]
- Pettus EH, Christman CW, Giebel ML, Povlishock JT. Traumatically induced altered membrane permeability: its relationship to traumatically induced reactive axonal change. J Neurotrauma. 1994;11:507–522. [Abstract] [Google Scholar]
- Pointillart V, Gense D, Gross C, Bidabe AM, Gin AM, Rivel J, Caille JM, Senegas J. Effects of nimodipine on posttraumatic spinal cord ischemia in baboons. J Neurotrauma. 1993;10:201–213. [Abstract] [Google Scholar]
- Povlishock JT. Traumatically induced axonal injury: pathogenesis and pathobiological implications. Brain Pathology. 1992;2:1–12. [Abstract] [Google Scholar]
- Ray SK, Hogan EL, Banik NL. Calpain in the pathophysiology of spinal cord injury: neuroprotection with calpain inhibitors. Brain Res Brain Res Rev. 2003;42:169–185. [Abstract] [Google Scholar]
- Rhee RY, Gloviczki P, Cambria RA, Lowell RC, Okazaki H. The effects of nimodipine on ischemic injury of the spinal cord during thoracic aortic cross-clamping. Int Angiol. 1996;15:153–161. [Abstract] [Google Scholar]
- Rodriguez A, Webster P, Ortego J, Andrews NW. Lysosomes behave as Ca2+-regulated exocytic vesicles in fibroblasts and epithelial cells. J Cell Biol. 1997;137:93–104. [Europe PMC free article] [Abstract] [Google Scholar]
- Sattler R, Tymianski M, Feyaz I, Hafner M, Tator CH. Voltage-sensitive calcium channels mediate calcium entry into cultured mammalian sympathetic neurons following neurite transection. Brain Res. 1996;719:239–246. [Abstract] [Google Scholar]
- Schanne FAX, Kane AB, Young EE, Farber JL. Calcium dependence of toxic cell death: a final common pathway. Science. 1979;206:700–702. [Abstract] [Google Scholar]
- Schlaepfer WW. Calcium-induced degeneration of axoplasm in isolated segments of rat peripheral nerve. Brain Res. 1974;69:203–215. [Abstract] [Google Scholar]
- Schlaepfer WW, Bunge RP. Effects of calcium ion concentration on the degeneration of amputated axons in tissue culture. J Cell Biol. 1973;59:456–470. [Europe PMC free article] [Abstract] [Google Scholar]
- Shi R. In vivo evidence of membrane damage following compression of adult guinea pig spinal cord. J Neurotrauma. 2002;19:1394. [Google Scholar]
- Shi R. The dynamics of axolemmal disruption in guinea pig spinal cord following compression. J Neurocytol. 2004;33:203–211. [Abstract] [Google Scholar]
- Shi R, Blight AR. Compression injury of mammalian spinal cord in vitro and the dynamics of action potential conduction failure. J Neurophysiol. 1996;76:1572–1580. [Abstract] [Google Scholar]
- Shi R, Borgens RB. Acute repair of crushed guinea pig spinal cord by polyethylene glycol. J Neurophysiol. 1999;81:2406–2414. [Abstract] [Google Scholar]
- Shi R, Pryor JD. Temperature dependence of membrane sealing following transection in mammalian spinal cord axons. Neuroscince. 2000;98:157–166. [Abstract] [Google Scholar]
- Shi R, Borgens RB. Anatomical repair of nerve membranes in crushed mammalian spinal cord with polyethylene glycol. J Neurocytol. 2000;29:633–643. [Abstract] [Google Scholar]
- Shi R, Pryor JD. Pathological changes of isolated spinal cord axons in response to mechanical stretch. Neuroscience. 2002;110:765–777. [Abstract] [Google Scholar]
- Shi R, Asano T, Vining NC, Blight AR. Control of membrane sealing in injured mammalian spinal cord axons. J Neurophysiol. 2000;84:1763–1769. [Abstract] [Google Scholar]
- Shi R, Qiao X, Emerson N, Malcom A. Dimethylsulfoxide enhances CNS neuronal plasma membrane resealing after injury in low temperature or low calcium. J Neurocytol. 2001;30:829–839. [Abstract] [Google Scholar]
- Shi R, Lucas JH, Wolf A, Gross GW. Calcium antagonists fail to protect mammalian spinal neurons after physical injury. J Neurotrauma. 1989;6:261–276. [Abstract] [Google Scholar]
- Spira ME, Benbassat D, Dormann A. Resealing of the proximal and distal cut ends of transected axons: electrophysiological and ultrastructural analysis. J Neurobiol. 1993;24:300–316. [Abstract] [Google Scholar]
- Steinhardt RA, Bi G, Alderton JM. Cell membrane resealing by a vesicular mechanism similar to neurotransmitter release. Science. 1994;263:390–393. [Abstract] [Google Scholar]
- Stokes BT, Fox P, Hollinden G. Extracellular calcium activity in the injured spinal cord. Exp Neurol. 1983;80:561–572. [Abstract] [Google Scholar]
- Strautman AF, Cork RJ, Robinson KR. The distribution of free calcium in transected spinal axons and its modulation by applied electrical fields. J Neurosci. 1990;10:3564–3575. [Europe PMC free article] [Abstract] [Google Scholar]
- Terasaki M, Miyake K, McNeil PL. Large plasma membrane disruptions are rapidly resealed by Ca2+-dependent vesicle-vesicle fusion events. J Cell Biol. 1997;139:63–74. [Europe PMC free article] [Abstract] [Google Scholar]
- Ueda K, Shinohara S, Yagami T, Asakura K, Kawasaki K. Amyloid beta protein potentiates Ca2+ influx through L-type voltage-sensitive Ca2+ channels: a possible involvement of free radicals. J Neurochem. 1997;68:265–271. [Abstract] [Google Scholar]
- Xie X, Barrett JN. Membrane resealing in cultured rat septal neurons after neurite transection: evidence for enhancement by Ca2+-triggered protease activity and cytoskeletal disassembly. J Neurosci. 1991;11:3257–3267. [Europe PMC free article] [Abstract] [Google Scholar]
- Yawo H, Kuno M. How a nerve fiber repairs its cut end: involvement of phospholipase A2. Science. 1983;222:1351–1352. [Abstract] [Google Scholar]
- Yawo H, Kuno M. Calcium dependence of membrane sealing at the cut end of the cockroach giant axon. J Neurosci. 1985;5:1626–1632. [Europe PMC free article] [Abstract] [Google Scholar]
- Young W. Role of calcium in central nervous system injuries. J Neurotrauma. 1992;9:S9–S25. [Abstract] [Google Scholar]
- Young W, Yen V, Blight A. Extracellular calcium ionic activity in experimental spinal cord contusion. Brain Res. 1982;253:105–113. [Abstract] [Google Scholar]
- Young W, Koreh I. Potassium and calcium changes in injured spinal cords. Brain Res. 1986;365:42–53. [Abstract] [Google Scholar]
- Ziv NE, Spira ME. Spatiotemporal distribution of Ca2+ following axotomy and throughout the recovery process of cultured Aplysia neurons. Eur J Neurosci. 1993;5:657–668. [Abstract] [Google Scholar]
Full text links
Read article at publisher's site: https://doi.org/10.1016/j.neuroscience.2007.02.015
Read article for free, from open access legal sources, via Unpaywall:
https://europepmc.org/articles/pmc2701192?pdf=render
Citations & impact
Impact metrics
Citations of article over time
Smart citations by scite.ai
Explore citation contexts and check if this article has been
supported or disputed.
https://scite.ai/reports/10.1016/j.neuroscience.2007.02.015
Article citations
Intra-axonal mechanisms driving axon regeneration.
Brain Res, 1740:146864, 28 Apr 2020
Cited by: 19 articles | PMID: 32360100 | PMCID: PMC8166413
Review Free full text in Europe PMC
Cellular mechanisms and signals that coordinate plasma membrane repair.
Cell Mol Life Sci, 75(20):3751-3770, 26 Jul 2018
Cited by: 46 articles | PMID: 30051163 | PMCID: PMC6541445
Review Free full text in Europe PMC
Calpains, cleaved mini-dysferlinC72, and L-type channels underpin calcium-dependent muscle membrane repair.
J Neurosci, 33(12):5085-5094, 01 Mar 2013
Cited by: 75 articles | PMID: 23516275 | PMCID: PMC6704986
Role of TRPM2 cation channels in dorsal root ganglion of rats after experimental spinal cord injury.
Muscle Nerve, 48(6):945-950, 11 Sep 2013
Cited by: 14 articles | PMID: 23512594
Diffuse traumatic axonal injury in the optic nerve does not elicit retinal ganglion cell loss.
J Neuropathol Exp Neurol, 72(8):768-781, 01 Aug 2013
Cited by: 34 articles | PMID: 23860030 | PMCID: PMC3753119
Go to all (17) article citations
Similar Articles
To arrive at the top five similar articles we use a word-weighted algorithm to compare words from the Title and Abstract of each citation.
Polyethylene glycol enhances axolemmal resealing following transection in cultured cells and in ex vivo spinal cord.
J Neurotrauma, 27(1):151-161, 01 Jan 2010
Cited by: 20 articles | PMID: 19691421
Spinal cord regeneration induced by a voltage-gated calcium channel agonist.
Neurol Res, 24(7):639-642, 01 Oct 2002
Cited by: 5 articles | PMID: 12392197
Dimethylsulfoxide enhances CNS neuronal plasma membrane resealing after injury in low temperature or low calcium.
J Neurocytol, 30(9-10):829-839, 01 Sep 2001
Cited by: 20 articles | PMID: 12165673
Voltage-dependent calcium channels regulate GH4 pituitary cell proliferation at two stages of the cell cycle.
J Cell Physiol, 146(2):197-206, 01 Feb 1991
Cited by: 23 articles | PMID: 1705563
Funding
Funders who supported this work.
NICHD NIH HHS (3)
Grant ID: R03 HD041371-01A1
Grant ID: R03 HD041371-02
Grant ID: R03 HD041371