Abstract
Free full text

Chemokines and the pathophysiology of neuropathic pain
Abstract
Chemokines and chemokine receptors are widely expressed by cells of the immune and nervous systems. This review focuses on our current knowledge concerning the role of chemokines in the pathophysiology of chronic pain syndromes. Injury- or disease-induced changes in the expression of diverse chemokines and their receptors have been demonstrated in the neural and nonneural elements of pain pathways. Under these circumstances, chemokines have been shown to modulate the electrical activity of neurons by multiple regulatory pathways including increases in neurotransmitter release through Ca-dependent mechanisms and transactivation of transient receptor channels. Either of these mechanisms alone, or in combination, may contribute to sustained excitability of primary afferent and secondary neurons within spinal pain pathways. Another manner in which chemokines may influence sustained neuronal excitability may be their ability to function as excitatory neurotransmitters within the peripheral and central nervous system. As is the case for traditional neurotransmitters, injury-induced up-regulated chemokines are found within synaptic vesicles. Chemokines released after depolarization of the cell membrane can then act on other chemokine receptor-bearing neurons, glia, or immune cells. Because up-regulation of chemokines and their receptors may be one of the mechanisms that directly or indirectly contribute to the development and maintenance of chronic pain, these molecules may then represent novel targets for therapeutic intervention in chronic pain states.
In his landmark treatise Sir Charles Scott Sherrington proposed the concept that pain is the evolved response to a potentially harmful, noxious stimulus (1). An example would be placing your hand on a hot iron and the resulting sensation of pain, which produces almost immediate withdrawal, thereby preventing tissue damage. This conscious perception of pain is the result of activity in a set of well defined neural pathways that start with the primary afferent neurons. These pseudounipolar neurons are responsible for the transmission of pain (“nociceptors”) and have unmyelinated or lightly myelinated axons. The cell bodies of nociceptors are found in the dorsal root ganglia (DRG). Nociceptors have both a peripheral connection that innervates potentially diseased or traumatized nerves, muscles, tendons, organs, and epithelia, and a centrally projecting axon that enters the central nervous system. This central axon conveys “nociceptive” information to second-order neurons in the dorsal horn of the spinal cord. Neural connections from the dorsal horn to the thalamus, and from there to the cortex, relay this noxious information to higher centers of conscious experience (Fig. 1). The central axons of primary afferent nociceptive neurons also provide information to polysynaptic spinal cord interneurons, which are essential for the initiation of the nociceptive withdrawal reflex. These neurons trigger motor reflexes that are important in the avoidance of potentially harmful painful stimuli (e.g., that hot iron). Descending pathways originating in the cortex and/or midbrain provide modulatory feedback signals at the level of the spinal cord that also regulate the nociceptive experience, thereby providing a closed-loop feedback control of this behavior. Additionally, impulses can travel back along the peripheral axon of the nociceptive sensory neuron toward the distal nerve endings, resulting in the local release of neuropeptides in the injury environment. This neuropeptide release produces vasodilatation, venule permeability, plasma extravasation, edema, and leukocyte influx, a process termed “neurogenic inflammation.”
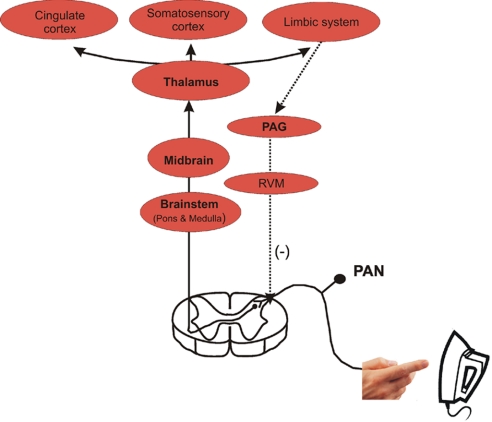
The neural pathway of nociception from primary afferent neurons (PANs) to the superficial lamina in the dorsal horn of the spinal cord. Second-order neurons in the dorsal horn convey the noxious signal to the brainstem, midbrain, and thalamus. Finally, third-order neurons relay the electrical signal to the somatosensory/cingulate cortex and limbic system. Descending modulatory influences arrive in the spinal cord dorsal horn (dashed lines) and are derived from the midbrain periaqueductal gray (PAG), the locus ceruleus, and the rostral ventromedial medulla (RVM).
Although pain clearly plays an important survival role in safeguarding the individual from potential sources of tissue destruction, the perception of pain can also be the result of a dysfunctioning nervous system. Typically, the local response to various types of injury or infection involves the release of peripheral chemical mediators. These injury-associated factors produce two effects. One role is to attract leukocytes to the point of injury as part of the inflammatory response (2) and the other is to sensitize nociceptors, enhancing their responses to pain (3). This increased excitatory activity produces transmitter release in the spinal cord enhancing neuronal activity in pain pathways in the central nervous system, a phenomenon known as spinal sensitization (4). Under some circumstances, nociceptor-driven electrical activity in the spinal cord becomes divorced from normal physiology, so that pain is produced in the absence of any appropriate stimulus (5, 6). This is now known as pathological or “neuropathic” pain.
Neuropathic pain is experienced in association with many types of injury to the nervous system or as a consequence of diabetes, cancer, infectious agents (e.g., HIV-1), or the toxic side effects of diverse drug regimens. From the behavioral point of view, neuropathic pain is associated with different types of hypersensitive pain behavior, including allodynia (pain evoked by a normally innocuous stimulus) and hyperalgesia (enhanced pain evoked by a noxious stimulus). In states of neuropathic pain, abnormal activity occurs in nociceptive neurons throughout the neuraxis. This activity is thought to result from the increased neuronal expression and activation of ion channels and receptors that initiate and mediate the abnormal generation of action potentials and synaptic transmission in pain pathways. But what causes these changes to occur? Presumably something provokes nociceptive neurons to express different sets of genes, resulting in a new and abnormal chronically hyperexcitable “pain” phenotype.
From the therapeutic point of view, neuropathic pain is an extremely intractable problem. Once established, pain of this type is not readily susceptible to treatment with nonsteroidal anti-inflammatory drugs (NSAIDs). Moreover, although opiates may be used for acute or for chronic pain states (e.g., terminal cancer), alleviation of neuropathic pain is more problematic because high doses are often required, narrowing the therapeutic index (7). The remaining available drugs used to treat these syndromes (tricyclic antidepressants, antiepileptics) are not particularly effective and are also associated with a number of negative side effects (8). Hence, a complete understanding of the cellular and molecular processes involved in the development of neuropathic pain is essential for the development of novel therapies.
It has been shown that peripheral nerve injury (trauma-, disease-, or drug-induced) can trigger a wide variety of cellular changes in sensory neurons and, as we have discussed, neuropathic pain following peripheral nerve injury is a consequence of enhanced excitability associated with the chronic sensitization of nociceptive neurons in the peripheral and central nervous systems. Interestingly, after a peripheral nerve injury, not only a subset of injured (9–12), but also neighboring noninjured peripheral sensory neurons exhibit spontaneous, ectopic discharges (13–18). Abnormal excitability of pain neurons may even extend to the spinal cord dorsal horn contralateral to the nerve injury (19–23, 69). Although it is clear that molecular changes in the sensory ganglia and spinal cord dorsal horn are responsible for chronic pain, it remains a mystery as to what event(s) are critical for its development and maintenance.
Peripheral Nerve Injury and Inflammation
One important development in our understanding of the cellular and molecular processes that produce neuropathic pain concerns the role of the immune system. Immunity can be dissociated into two different phases: innate and acquired. Acquired immunity involves the phenomenon of immunological memory and includes the antibody and lymphocyte responses to specific antigens. The forerunner to acquired immunity is the innate immune response. This more basic type of immunity involves a generalized immune cell response to a variety of toxic or pathological intrusions into physiological homeostasis. Molecules such as Toll-like receptors expressed by numerous types of cells, including leukocytes, Schwann cells, neurons, astrocytes, and microglia, can recognize generalized molecular patterns of infectious agents, cell debris, or other cellular detritus initiating a cascade of cytokine synthesis that orchestrates a general cellular response to these potential problems (27–30). As noted above, this response is inflammatory in nature and involves the recruitment of leukocytes to areas of tissue damage. The activation of innate immune inflammatory responses is also frequently linked to the development of pathology. In the present context, it is believed that the innate immune response to injury plays a prominent role in the establishment of chronic pain states, extending beyond its role in promoting the influx and activation of leukocytes. Although inflammatory and neuropathic pain syndromes are often considered distinct entities, emerging evidence suggests that proinflammatory substances produced in association with the innate immune response are clearly implicated in the actual development and maintenance of neuropathic pain and may be a necessary prelude to its development. As such, both neuroinflammatory and associated immune responses following nerve damage may contribute as much to the development and maintenance of neuropathic pain as the initial nerve damage itself. The traditional view has been that the influx of leukocytes associated with inflammation was responsible for secreting the chemical mediators that produced pain. However, as we will discuss, current evidence suggests that the role of the inflammatory response in the generation of pain is not limited to effects produced by the influx of leukocytes per se. Thus, it is currently believed that the proinflammatory cytokines that drive chronic pain behavior may be derived from the cellular elements of the nervous system itself, and that these molecules can act directly on receptors expressed by neurons and other cells of the nervous system. The effects produced by these factors may lead to chronic hyperexcitability and alterations in gene expression by nociceptors, abnormal processing of pain signals, and enhanced pain states. In this way, signaling pathways designed to facilitate a protective response to tissue injury become sources of chronic pathological pain.
The question then arises as to exactly which inflammatory cytokines are concerned in the establishment of chronic pain and the molecular processes that underlie pain hypersensitivity. The innate immune response is associated with the development of a complex cascade of cytokine expression in which many inflammatory mediators are synthesized in a mutually dependent manner. It is likely that several of these inflammatory mediators are concerned in the establishment of the “pain” phenotype that characterizes neuropathic pain.
One family of cytokines that has recently come to light as playing a central role in the induction and maintenance of chronic pain are the CHEMOtactic cytoKINES (chemokines). Chemokines are small, secreted proteins that exert all of their known effects through the activation of a family of related G protein-coupled receptors (GPCRs). Chemokines were originally identified because of their chemoattractant effects that control the migration of different classes of leukocytes in association with the development of inflammation (2). Several subfamilies of chemokines and their receptors are known to exist (Table 1). Most chemokines are not constitutively expressed at high levels, their production and secretion being normally associated with activation of the inflammatory response (Fig. 2). An exception to this rule is the chemokine, stromal cell-derived factor-1 (SDF-1/CXCL12). SDF-1 is the most evolutionarily ancient member of the chemokine family; it existed phylogenetically before the development of an immune system, indicating that chemokine signaling originally played a role other than the regulation of leukocyte chemotaxis (31, 32). Still, chemotaxis appears to be one ancient function of this chemokine as well. In mammals, SDF1 signaling through its major receptor, CXCR4, has been shown to be important for the development of the embryo, where SDF-1 regulates the migration of the stem/progenitor cells that form numerous tissues (33, 34). Postnatally, this signaling system is still used in this way to retain hematopoietic stem cells in the bone marrow (35).
Table 1.
Chemokine receptor selectivity
Receptor | Chemokines, systematic name (functional name) |
---|---|
CC chemokine receptors | |
![]() ![]() ![]() ![]() | CCL3 (MIP-1α), CCL5 (RANTES), CCL7 (MCP-3), CCL13 (MCP-4) |
![]() ![]() ![]() ![]() | CCL2 (MCP-1), CCL7 (MCP-3), CCL8 (MCP-2), CCL12 (MCP-5), CCL13 (MCP-4) |
![]() ![]() ![]() ![]() | CCL5 (RANTES), CCL7 (MCP-3), CCL8 (MCP-2), CCL11 (Eotaxin), CCL13 (MCP-4), CCL15 (MIP-5), CCL24 (Eotaxin-2), CCL26 (Eotaxin-3) |
![]() ![]() ![]() ![]() | CCL2 (MCP-1), CCL3 (MIP-1α), CCL5 (RANTES), CCL17 (TARC), CCL22 (MDC) |
![]() ![]() ![]() ![]() | CCL3 (MIP-1α), CCL5 (RANTES), CCL8 (MCP-2), CCL14 (MIP-1β) |
![]() ![]() ![]() ![]() | CCL20 (Exodus-1/LARC/MIP-3α) |
![]() ![]() ![]() ![]() | CCL19 (Exodus-3/ELC/MIP-3β), CCL21 (Exodus-2/SLC/6Ckine) |
![]() ![]() ![]() ![]() | CCL1 (I-309), CCL17 (TARC), CCL19 (Exodus-3/ELC/MIP-3β) |
![]() ![]() ![]() ![]() | CCL2 (MCP-1), CCL3 (MIP-1α), CCL5 (RANTES), CCL8 (MCP-2), CCL11 (Eotaxin), CCL13 (MCP-4), CCL14 (MIP-1β), CCL25 (TECK) |
![]() ![]() ![]() ![]() | CCL27 (CTACK), CCL28 (MEC) |
![]() ![]() ![]() ![]() | CCL2 (MCP-1), CCL19 (Exodus-3/ELC/MIP-3β), CCL21 (Exodus-2/SLC/6Ckine), CCL25 (TECK) |
CXC chemokine receptors | |
![]() ![]() ![]() ![]() | CXCL6 (GCP-2), CXCL8 (IL-8) |
![]() ![]() ![]() ![]() | CXCL1 (GRO-α) CXCL2 (GRO-β), CXCL3 (GRO-γ), CXCL5 (ENA-78), CXCL6 (GCP-2), CXCL7 (NAP-2), CXCL8 (IL-8) |
![]() ![]() ![]() ![]() | CXCL10 (IP-10), CXCL9 (MIG), CXCL11 (ITAC) |
![]() ![]() ![]() ![]() | CXCL12 (SDF-1) |
![]() ![]() ![]() ![]() | CXCL13 (BLC) |
![]() ![]() ![]() ![]() | CXCL16 |
![]() ![]() ![]() ![]() | CXCL11 (ITAC), CXCL12 (SDF-1) |
CX3C chemokine receptor | |
![]() ![]() ![]() ![]() | CX3CL1 (Fractalkine) |
C chemokine receptor | |
![]() ![]() ![]() ![]() | XCL1 (Lymphotactin), XCL2 (SCM-1b) |
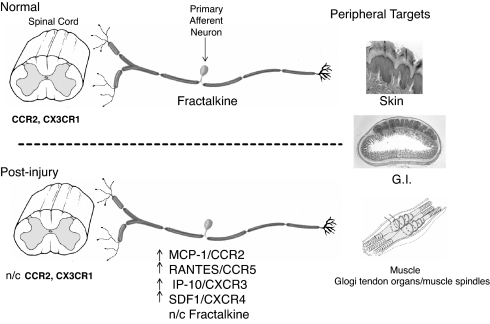
Peripheral and central targets of primary afferent neurons. (Upper) The somas of nociceptive neurons are housed together with myelinating Schwann cells and nonmyelinating Schwann cells (satellite cells) in the dorsal root ganglia (DRG). These pseudounipolar neurons communicate with both peripheral target tissue (epithelia, muscle, and visceral organs) and neuronal/nonneuronal elements of the spinal cord dorsal horn (neurons, microglia, and astrocytes). Some chemokine/receptors are present in both CNS and PNS cellular elements. (Lower) Following injury, compromised sensory neurons and adjacent nonneuronal cells produce chemokines and their receptors within the DRG (n/c indicates no change in mRNA or protein expression). These chemokines can be released within the DRG as well as from axons in peripheral target tissue and spinal cord dorsal horn. Increased cellular signaling between chemokines and receptors may be responsible for changes in neuronal excitability.
Although chemokines clearly have a central role in orchestrating the normal inflammatory response, the pathogenesis of many chronic inflammatory conditions such as atherosclerosis, arthritis, and inflammatory bowel disease has been shown to be mediated in large part by the actions of chemokines (2). In addition, numerous neurological conditions accompanied by activation of the innate immune response during their onset or progression appear to involve the action of chemokines in their pathogenesis. These include autoimmune disorders (e.g., multiple sclerosis), neurodegenerative disorders (e.g., cerebral ischemic injury, Parkinson's, Huntington's, and Alzheimer's diseases), as well as virus-based diseases (e.g., HIV-1 and herpes simplex) (36–38). This chemokine-mediated component is also likely to extend to the pathogenesis and maintenance of chronic pain in both disease-related conditions (e.g., multiple sclerosis, HIV-1, and herpes simplex) and after trauma, all of which are associated with innate immune responses and prolonged expression of chemokines and their receptors by the cellular elements of the nervous system (39). This being the case, interference with chemokine function represents a promising approach for the development of both novel anti-inflammatory medication and the treatment of chronic pain conditions.
Chemokines and Their Receptors in Acute and Chronic Pain
There is now a large amount of data indicating that, as with other cytokines, chemokines and their receptors can influence both the acute and chronic phases of pain. However, why is chemokine function of particular interest in this regard? As indicated above, it has become apparent that the cellular elements of the nervous system (e.g., neurons, glia, and microglia) are able to both synthesize and respond to chemokines, something that is quite independent of their role in the regulation of leukocyte chemotaxis and function. Oh et al. (40) first demonstrated that the simple injection of the chemokines SDF-1, Regulated upon Activation, Normal T cell Expressed, and Secreted (RANTES/CCL5) or macrophage inflammatory protein-1α (MIP-1α/CCL3) into the adult rat hind paw produced dose-dependent tactile allodynia. Indeed, these authors demonstrated that cultured DRG neurons expressed numerous types of chemokine receptors, indicating that the observed pain behavior might result from a direct action of chemokines on these neurons. In support of this possibility, chemokines were found to strongly excite DRG neurons in culture (41, 42) and chemokine-induced excitation was associated with the release of pain related neurotransmitters such as Substance P and calcitonin gene-related peptide (CGRP) (43, 44). The cellular mechanism underlying chemokine-induced excitation of sensory neurons in culture has been shown to have at least two components. The first of these components is the transactivation of transient receptor potential cation channels (TRP), such as TRPV1 and TRPA1, which are also expressed by populations of nociceptive neurons (44–46) (Fig. 3). The second component is the inhibition of K+ conductances that normally regulate neuronal excitability. MIP-1α, for example, can enhance the thermal sensitivity of TRPV1 (47). The receptor for MIP-1α, CCR1, is expressed by >85% of cultured DRG neurons, which also express TRPV1 (47). Activation of other chemokine receptors, such as CCR2 expressed by cultured DRG neurons (see below), also produces excitation through transactivation of both TRPV1 and TRPA1 (44) (Fig. 3). In the former instance the mechanism of activation appears to be due to phospholipase C-induced removal of tonic PIP2-mediated channel block (48), whereas in the second instance the transactivation appears to involve a PKC-mediated event (49–51). Importantly, TRPA1 activation is central to acute pain, neuropeptide release, and neurogenic inflammation (52, 53). These data suggest that chemokine-induced excitation involving TRP channel activation may be of key importance in driving the increased excitation observed in chronic pain states.
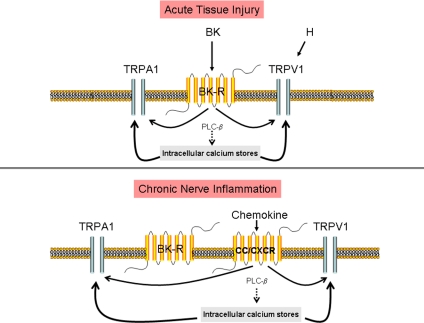
Crosstalk among chemokine receptors, bradykinin, and transient receptor potential channels (TRP). (Upper) In sensory neurons, treatment with the proinflammatory bradykinin peptide results in hyperalgesia. Bradykinin also enhances the sensitivity of TRPV1 and TRPA1, through a signal transduction cascade involving Gi protein, PLCβ, and PKC. PLCβ hydrolyzes phosphoinositol 4,5-biphosphate (PIP2), an endogenous inhibitor of TRPV1, thereby sensitizing the TRPV1 pain receptor. Activation of PKC can simultaneously produce activation of TRPA1 receptors. (Lower) Nerve injury up-regulates the chemokine receptor, CCR2. Activation of CCR2 by MCP-1 can now sensitize nociceptors via transactivation of the transient receptor potential channels, TRPV1 and TRPA1. Injury-induced up-regulation of MCP-1/CCR2 signaling by sensory neurons may participate sustained excitability of primary afferent neurons.
A significant question is whether such data, mostly obtained in cell culture studies, has relevance to the situation prevailing in chronic pain states in vivo. More recent evidence supporting a key role for chemokines and their receptors in chronic pain has come from the results of experiments using several accepted models of neuropathic pain in rodents. These models include sciatic nerve transaction (54, 55), partial ligation of the sciatic nerve (26, 56, 57), chronic constriction injury of the sciatic nerve (58–60), chronic compression of the L4L5 DRG (CCD), a rodent model of spinal stenosis (41, 42), lysophosphati dylcholine-induced focal nerve demyelination (ref. 44;), bone cancer pain (61, 62), and zymosan-induced inflammatory pain (58, 63, 64). Each of these models resulted in up-regulation of one or more chemokine receptors by DRG neurons associated with, or in close proximity to, the injury. Moreover, in several instances it has also been demonstrated that sensory neurons will actually up-regulate the synthesis of chemokines in addition to their cognate receptors (Fig. 4) (41, 42, 44, 65). Thus, in association with chronic pain the same DRG neuron may up-regulate both a chemokine and its receptor, suggesting some form of cell autologous regulation of DRG excitability by these molecules may occur. For example, it might be imagined that under these circumstances DRG neurons could release chemokines that would then activate receptors expressed by the same neuron or others in the vicinity. Because chemokines can excite DRG neurons, this process might contribute to the neuronal hyperexcitability observed under these conditions. As chemokines are also of central importance in the recruitment of leukocytes, they would have a unique role in simultaneously coordinating inflammation and neuronal excitability.
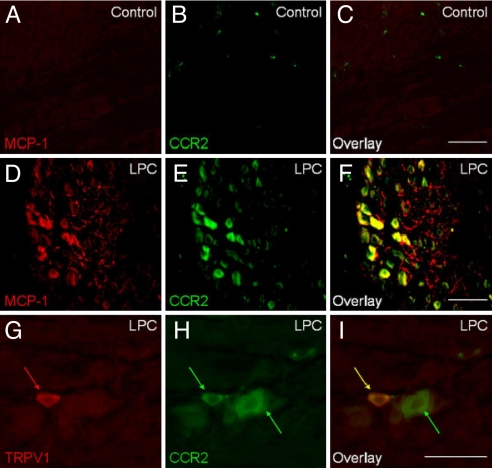
Primary afferent neurons express MCP-1 and CCR2 in association with peripheral neuropathy. Sciatic nerve demyelination injury was induced by lysophosphatidylcholine (LPC) in CCR2-EGFP BAC transgenic mice. DRG were isolated at postoperation day (POD) 14, cryosectioned, and subjected to immunohistochemistry by using a polyclonal anti-MCP-1 antibody. (A–C) Sham-operated control. (D–F) LPC-treated group. Note that many neuronal cell bodies express both MCP-1 and CCR2. In addition, MCP-1 is also observed in numerous axon processes throughout the ganglion. (G–I) TRPV1-expressing nociceptors (red arrows) up-regulated CCR2 expression (yellow arrow). Some of larger neurons that do not express TRPV1 also expressed CCR2 (green arrow). (Scale bars, 100 μm.) [Reproduced with permission from ref. 44. (Copyright 2007, Blackwell).]
One good example of the validity of this type of model concerns the potential role of the chemokine MCP-1 and its receptor CCR2 in the genesis of neuropathic pain. The role of MCP-1/CCR2 signaling was first intimated in neuropathic pain states following peripheral nerve injury in genetically engineered mice lacking CCR2 receptors. These receptor knockout mice failed to display mechanical hyperalgesia after partial ligation of the sciatic nerve without a detectable change in acute pain behavior (56), whereas transgenic mice overexpressing glial MCP-1 exhibited enhanced nociceptive responses (66).
In keeping with these results, exogenous administration of MCP-1 produced both membrane threshold depolarization and action potentials in a subpopulation of small, medium, and large neurons in the intact DRG from animals with neuropathic pain resulting from chronic compression of the DRG (CCD) (41, 42). Examination of the expression pattern for CCR2 receptors in the DRG demonstrated that they were not normally expressed by DRG neurons in vivo, but were extensively up-regulated in models of neuropathic pain where they were usually present in the same neurons that up-regulated the expression of MCP-1 under the same circumstances (42) (Fig. 4). Appropriately, the excitatory electrophysiological effects of MCP-1 were not observed in control animals. Subsequently, two ionic mechanisms contributing to the excitatory effects of MCP-1 were identified in acutely dissociated small-diameter DRG neurons isolated from animals previously subjected to CCD. MCP-1 activated a non-voltage-dependent, depolarizing current with properties of a nonselective cation conductance, quite possibly a TRP channel consistent with the data obtained in cell culture studies (44), and it also inhibited a voltage-dependent outward current (41).
Such chemokine-induced excitatory effects on sensory neurons may further facilitate the axonal transport and the release of excitatory neuropeptides, such as CGRP (43) and Substance P from the terminals of DRG neurons in the spinal cord. Zhang and De Koninck recently demonstrated that MCP-1 is also present in central afferent fibers in the spinal cord (59). Thus, electrical activity due to peripheral nerve injury may also stimulate central afferent release of MCP-1 into the spinal cord dorsal horn, further activating CCR2-expressing glial cells or central neurons (56, 59, 67). Neurons from the dorsal horn express CCR2 receptors and MCP-1/CCR2 signaling reduces the inhibitory effects of GABA on these cells. Hence, release of MCP-1 may mediate excitatory effects both at the level of the DRG and spinal cord.
Overall, these results suggest that MCP-1 may function as an up-regulatable neurotransmitter in DRG neurons, whose expression is associated with the development of states of chronic pain hypersensitivity. Examination of the distribution of MCP-1 at a subcellular level after its synthesis in cultured DRG neurons has revealed that it is initially processed by the trans-Golgi network and packaged into the same synaptic vesicles as the peptide neurotransmitter CGRP (44). Vesicles that contain both proteins can be observed in the neuronal soma and after transport to nerve terminals. Depolarization of these neurons results in Ca-dependent release of MCP-1 either from the soma or nerve terminals (44). Presumably, release of the chemokine from the cell soma within the DRG would have the effect of depolarizing neighboring CCR2-expressing neurons, eliciting excitation and promoting further MCP-1 release within the DRG or within the dorsal horn or the spinal cord where it could interact with CCR2-expressing neurons and glia. In this way up-regulation of MCP-1 and CCR2 might be an important trigger for the up-regulation of DRG hyperexcitability and maintenance of chronic pain. Note that in the brain the chemokine CCL21/exodus has also been shown to be up-regulated by neurons after excitotoxic stimulation and to be packaged into secretory vesicles and released on neuronal depolarization (68). Thus, it appears that when chemokines are expressed in neurons under different circumstances they may generally play a novel role as neurotransmitters. MCP-1 and CCR2, as well as certain other chemokines and chemokine receptors, exhibit an exceptionally prolonged up-regulation in the injury-associated DRG (42, 59) and the trigeminal ganglion following peripheral nerve injury§ or herpes simplex virus infection (70–72), supporting the possibility that this type of signaling could contribute to the chronic nature of neuropathic pain.
Overall, the evidence suggests that prolonged chemokine and chemokine receptor expression in sensory ganglia may well be a significant contributor to many injury-induced and virus-associated neuropathic pain syndromes. This being the case, it is also of interest to define the signaling pathways in DRG neurons that result in the up-regulation of chemokine and chemokine receptor expression because they may represent novel targets for intervention in the treatment of chronic pain. In the case of CCR2 receptors some information on this issue has been obtained. Analysis of the structure of the mouse and human CCR2 genes revealed several upstream regulatory elements that might potentially mediate the action of different transcription factors. Included in these is a conserved binding site for the transcription factor, nuclear factor of activated T cells (NFAT) (73). Members of the NFAT family of proteins are expressed by DRG neurons, and expression of constitutively active NFAT derivatives produced up-regulation of CCR2 receptors in these neurons (73). NFAT is activated by being dephosphorylated by the Ca-dependent phosphatase calcineurin. When [Ca]i is increased in DRG neurons after depolarizing and Ca influx by voltage-dependent Ca channels, for example, calcineurin and NFAT are activated, resulting in the up-regulation of CCR2 expression (73). This then provides a possible pathway for the induction of CCR2 in the context of neuropathic pain. An injury-induced upstream mediator is envisaged as initially depolarizing DRG neurons, leading to Ca influx and CCR2 up-regulation. Once up-regulated, signaling by CCR2 could increase DRG excitation further and potentiate ongoing excitability. Interestingly, although MCP-1 is up-regulated by DRG neurons together with CCR2 in chronic pain (42), MCP-1 is not a target gene for NFAT regulation. However, we have observed that MCP-1 is up-regulated in DRG neurons by the action of the cytokine TNF-α (73). Indeed, TNF-α is known to increase the excitability of DRG neurons (74, 75). Potential sources of TNF-α release include the cells associated with the peripheral injury site and glial cells adjacent to primary afferent neuron central projections within the spinal cord (microglia and astrocytes) (84, 105). Thus, TNF-α may be the upstream regulator of chemokine signaling in these cells. Such a possibility would also help to explain why increased DRG excitability extends to neurons that are both ipsilateral and contralateral to the injured neurons, observations that suggest an important role for a diffusible mediator in triggering these events.
Although most of the data obtained on chemokine signaling in the DRG in the context of neuropathic pain concerns the role of MCP-1/CCR2, it is clear that other types of chemokine signaling may also be dynamically regulated under similar conditions. Up-regulated expression of the CXCR3, CXCR4, and CCR5 receptors, and their chemokine ligands, has also been observed in populations of DRG neurons in chronic pain models (69). As with CCR2, up-regulation of some of these chemokine receptors appears to be NFAT-dependent (e.g., CCR5), whereas others do not (e.g., CXCR4) (73). The precise expression patterns and time course of up-regulation of these diverse chemokine signaling systems differs in each case, and so the details of how they each participate in pain behavior may vary according to the circumstances and will require further clarification. Nevertheless, in so far as they have been investigated, it appears that all of these chemokines are packaged into neurotransmitter secretory vesicles in DRG neurons (44, 68). It is interesting that, in the case of SDF-1 and MCP-1, these vesicle populations are clearly different (unpublished observations), indicating that even when two chemokines are secreted by the same neuron they may subserve somewhat different functions.
As an example of the role of chemokine signaling in chronic pain one might consider PNS and CNS inflammatory demyelinating diseases such as Guillain–Barré syndrome, Charcot–Marie–Tooth disease types 1 and 4, and multiple sclerosis, which are frequently accompanied by a neuropathic pain syndrome (76, 77). Epidemiological studies suggest that chronic pain syndromes afflict 50–80% of patients with multiple sclerosis and 70–90% of individuals with Guillain–Barré syndrome (78). Disease-related components that may be central to this neuropathic pain symptomology include axon and Wallerian degeneration (79), which may act as a trigger for the cytokine cascades that result in the up-regulation and chronic expression of chemokines and their cognate receptors (2, 80).
Studies of several rodent models of demyelinating diseases known to elicit neuropathic pain behavior include late-developing peripheral axon demyelination in periaxin knockout mice (81), lysophosphatidylcholine-induced transient focal demyelination of the sciatic nerve in mice and rats (44, 69, 82), and the late, acute clinical phase of experimental autoimmune neuritis (83), and indicate a possible role of chemokine-influenced chronic pain in these models. Recent studies on rats and mice subjected to transient focal demyelination of the sciatic nerve revealed chronic up-regulation of MCP-1 and IFN-γ-inducing protein-10 (IP-10/CXCL10) and the chemokine receptors, CCR2, CCR5, and CXCR4 in primary sensory neurons (69). Application of these same chemokines to neurons isolated from DRG of animals after demyelination produced an increase in excitation. Thus, up-regulation of these chemokines and their receptors may effectively drive the chronic excitability and pain behavior in demyelinating diseases of this type. It is also of interest that administration of small molecule CCR2 receptor antagonists to these animals afforded some relief from ongoing pain, further indicating the role of chemokine signaling and the potential therapeutic effectiveness of inhibiting these events (69).
Chemokines, Glia and Chronic Pain
The preceding discussion has focused on the role of chemokines and their receptors expressed by neurons, in particular. However, it is clear that these molecules are expressed by other types of cells in the DRG and CNS which may also participate in the development and maintenance of neuropathic pain. Some chemokine and chemokine receptor signaling events after peripheral injury or infection appear to be primarily mediated by molecular and/or morphological remodeling of glial cells that, in turn, become a source of inflammatory mediators. It has been proposed that such “activated” Schwann cells, astrocytes, and microglia also play an essential role in the development of chronic pain hypersensitivity (84). Indeed, drugs that inhibit the activation of these cells also seem to interfere with the development of chronic pain behavior, presumably by suppressing the release of inflammatory mediators such as chemokines associated with their activation.
One of the recent breakthroughs in pain research has been recognition that reciprocal communication between neurons and microglia is important in regulating the quiescent and reactive states of glial cells. In addition to the glial receptors for ATP, neuropeptides, neurotransmitters, and neurotrophic factors, chemokines and their receptors appear to also contribute to these events. Activated astrocytes and microglia are also rich sources of chemokine production that could then interact with pain-related mechanisms in the dorsal horn and higher up the neuraxis (29). A clear example of the central role for chemokine signaling in mediating communication between DRG neurons and microglia in the spinal cord involves the chemokine fractalkine/CX3CL1 and its receptor, CX3CR1. Fractalkine has an unusual structure in that it is tethered to the membrane by means of a transmembrane mucin-like stalk. Normally, fractalkine is expressed by neurons and its receptor is particularly highly expressed by microglia (64). Fractalkine can signal to its receptor on target cells in a “tethered” state or it can be released after proteolytic cleavage producing a soluble form of the chemokine that can act at a distance (58). Injection of fractalkine into the spinal cord produces pain hypersensitivity and it has been observed that soluble fractalkine is produced in some chronic pain models (57, 58, 85, 86). Recent studies have revealed how fractalkine may act in vivo. It has been demonstrated that the enzyme cathepsin S, which can cleave tethered membrane-bound fractalkine to its soluble form, can itself be released from activated microglia (87). The released fractalkine can then act on microglia to up-regulate the release of proallodynic inflammatory mediators. Thus, fractalkine may act as a neuronal to glial messenger that amplifies ongoing pain producing mechanisms. This model also illustrates the fact that neurons and glia can interact in a variety of complex ways to elaborate ongoing pain stimuli, producing the mediators that may then initiate transcriptional changes resulting in chronic neuronal hyperexcitability and pain. In addition to the example of fractalkine, activated spinal microglia also express CCR2 and CXCR3 receptors, making them potential targets for MCP-1 or IP-10 up-regulated and released from DRG neurons (56, 88, 89).
Glia in the DRG and peripheral nerve may also represent a source of, and a target for, the action of chemokines. For example, in response to nerve injury MCP-1 is up-regulated in Schwann cells (55, 90, 91). Thus, these cells might also represent a source of chemokine release for the activation of CCR2 chemokine receptors up-regulated in adjacent DRG neurons.
The key involvement of chemokine signaling between glia and DRG neurons appears to be likely in certain chronic pain states such as those experienced in association with HIV-1 infection. This has particular relevance for the present discussion because the cellular receptors for gp120, the HIV-1 coat protein, are the CXCR4 and CCR5 chemokine receptors. One example of an HIV-1-associated pain syndrome is distal symmetrical polyneuropathy (DSP), which affects as many as one third of all HIV-1-infected individuals (92). This painful sensory neuropathy frequently begins with paresthesias in the fingers and toes progressing over weeks to months, followed by the development of pain, often of a burning and lancinating nature, which can make walking very difficult. Measurements of pain hypersensitivity have demonstrated allodynia and hyperalgesia in HIV-1-infected individuals. Interestingly, as is the case of HIV-1-associated effects on the CNS, there is no productive infection of peripheral neurons by the virus. Thus, indirect effects of HIV-1 must lead to the development of this pain state.
In addition to the effects of inflammatory mediators (including chemokines) released by virally infected leukocytes, there are at least two ways in which HIV-1-induced DSP may involve the direct effects of HIV-1 gp120 on chemokine receptors in the DRG: (i) viral protein shedding in the peripheral nervous system might enable gp120 to produce painful neuropathy via glial to neuronal signaling in the DRG and/or spinal cord (93, 94) or (2) by the direct activation of CCR5/CXCR4-bearing sensory neurons by gp120 (40, 95, 96). Indeed, Keswani and colleagues have presented a model in which gp120 can act in both these ways (93, 97). In the first instance these authors have demonstrated that binding of gp120 to CXCR4 receptors expressed by DRG satellite glial cells up-regulates the release of the chemokine RANTES which can then activate CCR5 receptors expressed by DRG neurons. In the second instance, gp120 can directly bind to and activate CXCR4 receptors expressed by DRG neurons (40). Moreover, this initial excitation of DRG neurons by gp120 and/or glial mediators might produce Ca2+-dependent up-regulation of CCR2 expression by these neurons by the mechanisms discussed above (73), leading to a second level of chemokine-mediated excitation. In support of such a model we have observed that treatment of the sciatic nerve with a T-tropic gp120 subsequently leads to MCP-1 and CCR2 up-regulation (unpublished observations), which would also be expected to promote excitation of DRG neurons.
Complicating matters further, AIDS patients who are treated by highly active, antiretroviral therapeutical (HAART) agents can also develop a painful sensory neuropathy. Intriguingly, the symptoms of this syndrome are clinically indistinguishable from those of HIV-1-induced DSP, including a burning sensation in the hands and feet and hypersensitivity to pain (92, 98, 99). The fact that the two syndromes are usually seen in association with one another makes diagnosis more difficult.
Recent studies have shed new light on the mechanisms of HAART-induced DSP and the role of chemokine signaling, in particular. It was observed that the drug, 2′,3′-dideoxycytidine (zalcitabine or ddC), produced neuropathic pain behavior together with up-regulated expression of both SDF-1 and CXCR4 in DRG satellite glial cells and some neurons. This suggests that SDF-1 release from DRG glia might be involved in the autologous regulation of excitatory substances from these same cells and that released SDF-1 might also directly excite DRG neurons. Significantly, zalcitabine-induced pain was completely blocked by the CXCR4 antagonist AMD3100, illustrating the key role of CXCR4 signaling in this behavior (65). Hence, the proallodynic actions of both HIV-1 and of zalcitabine depend on chemokine signaling between DRG glia and neurons.
Chemokine Interactions with Other Neurotransmitters
As we have discussed, chemokines and their receptors expressed by DRG neurons in chronic pain conditions may contribute to nociceptor hyperexcitability in many instances by directly exciting these neurons. However, other consequences of chemokine signaling may also indirectly contribute to pain behavior. One important example of this concerns chemokine interactions with the endogenous opioid system. Generally speaking, activation of μ-opioid receptors in the DRG and dorsal horn reduces neuronal excitability and synaptic transmission at synapses between nociceptors and first-order neurons in the spinal cord. This is believed to be one of the mechanisms by which opiates reduce pain behavior. However, it has been demonstrated that up-regulation of chemokine signaling consistently down-regulates μ-opioid receptor function (47, 100). One mechanism through which this may occur is by heterologous desensitization resulting from the effects of chemokine receptor activation on μ-receptor function (101, 102). In addition, FRET studies have demonstrated that some chemokine receptors can directly dimerize with μ-opioid receptors, raising the possibility that this interaction may also alter μ-receptor signaling (103). Interestingly, the opposite may also be true as the μ-opiate receptor agonist [d-Ala2,N-MePhe4,Gly5-ol]enkephalin can down-regulate chemokine activation of chemokine receptors, something that may account for opiate drug-induced immune suppression (104). As we have discussed, chemokines expressed by DRG neurons may also be responsible for chemoattractant effects resulting in leukocyte influx into the ganglia. Some leukocytes can actively secrete opioid peptides, an action that is potentially analgesic (24, 25). Thus, chemokines may be potentially proalgesic by directly exciting DRG neurons and down-regulating opioid signaling as well as potentially analgesic owing to their effects on endorphin release from leukocytes. How these diverse effects play out in the context of chronic pain behavior is incompletely understood and may have different degrees of importance depending on the precise type of pain syndrome under consideration. Clearly, however, there are numerous cellular mechanisms through which up-regulated chemokine signaling might result in pain hypersensitivity or related phenomena.
Conclusions
Recent research has made it clear that inflammatory processes are critical for the development of states of chronic pain and for the changes in behavior of pain neurons that accompany these syndromes. The development of such behavior may involve reciprocal signaling interactions between the different cellular elements of the central and peripheral nervous systems. As we have discussed here, chemokines seem to be one set of molecules that play a key role in coordinating injury associated nociceptive events as they serve to regulate inflammatory responses and can simultaneously act on elements of the nervous system. Importantly, chemokines in DRG neurons seem to act as up-regulatable neurotransmitters that produce excitatory effects in the DRG and spinal cord through a variety of mechanisms. The ability of small molecule antagonists of CCR2 and CXCR4 receptors to ameliorate ongoing pain hypersensitivity in animal models clearly indicates the importance of chemokine signaling in this behavior. We therefore conclude that targeting the chemokine system may provide a novel form of therapeutic intervention into states of chronic pain.
ACKNOWLEDGMENTS.
This work was supported by National Institutes of Health Grant NS049136, a National Multiple Sclerosis Society Pilot Award, and Illinois Excellence in Medicine, State of Illinois (to F.A.W.); and National Institutes of Health Grants NS043095, DA013141, and MH040165 (to R.J.M.).
Footnotes
The authors declare no conflict of interest.
§White F, Bauer W, Jellish W, Chan D, LaMotte R, Miller R, 25th Annual Scientific Meeting of the American Pain Society, May 3–6, 2006, San Antonio, TX, poster 682.
References
Articles from Proceedings of the National Academy of Sciences of the United States of America are provided here courtesy of National Academy of Sciences
Full text links
Read article at publisher's site: https://doi.org/10.1073/pnas.0709250104
Read article for free, from open access legal sources, via Unpaywall:
https://europepmc.org/articles/pmc2154400?pdf=render
Citations & impact
Impact metrics
Article citations
Update on Biomarkers of Chronic Inflammatory Processes Underlying Diabetic Neuropathy.
Int J Mol Sci, 25(19):10395, 27 Sep 2024
Cited by: 0 articles | PMID: 39408723 | PMCID: PMC11476795
Review Free full text in Europe PMC
Exploring the role of spinal astrocytes in the onset of hyperalgesic priming signals in acid-induced chronic muscle pain.
PNAS Nexus, 3(9):pgae362, 30 Aug 2024
Cited by: 0 articles | PMID: 39228816 | PMCID: PMC11370897
Causal association between circulating inflammatory markers and sciatica development: a Mendelian randomization study.
Front Neurol, 15:1380719, 02 Jul 2024
Cited by: 0 articles | PMID: 39015317 | PMCID: PMC11250389
A New Application for Cenicriviroc, a Dual CCR2/CCR5 Antagonist, in the Treatment of Painful Diabetic Neuropathy in a Mouse Model.
Int J Mol Sci, 25(13):7410, 05 Jul 2024
Cited by: 1 article | PMID: 39000516
CC Chemokine Family Members' Modulation as a Novel Approach for Treating Central Nervous System and Peripheral Nervous System Injury-A Review of Clinical and Experimental Findings.
Int J Mol Sci, 25(7):3788, 28 Mar 2024
Cited by: 2 articles | PMID: 38612597 | PMCID: PMC11011591
Review Free full text in Europe PMC
Go to all (217) article citations
Other citations
Wikipedia
Similar Articles
To arrive at the top five similar articles we use a word-weighted algorithm to compare words from the Title and Abstract of each citation.
Chemokines as pain mediators and modulators.
Curr Opin Anaesthesiol, 21(5):580-585, 01 Oct 2008
Cited by: 52 articles | PMID: 18784482 | PMCID: PMC2702665
Review Free full text in Europe PMC
Chemokines in neuron-glial cell interaction and pathogenesis of neuropathic pain.
Cell Mol Life Sci, 74(18):3275-3291, 07 Apr 2017
Cited by: 147 articles | PMID: 28389721 | PMCID: PMC11107618
Review Free full text in Europe PMC
Role of the immune system in neuropathic pain.
Scand J Pain, 20(1):33-37, 01 Dec 2019
Cited by: 92 articles | PMID: 31730538
Review
Chemokines and pain mechanisms.
Brain Res Rev, 60(1):125-134, 25 Dec 2008
Cited by: 169 articles | PMID: 19146875 | PMCID: PMC2691997
Review Free full text in Europe PMC
Funding
Funders who supported this work.
NIDA NIH HHS (2)
Grant ID: R01 DA013141
Grant ID: DA013141
NIMH NIH HHS (2)
Grant ID: MH040165
Grant ID: R37 MH040165
NINDS NIH HHS (5)
Grant ID: NS043095
Grant ID: NS049136
Grant ID: R01 NS049136
Grant ID: R01 NS049136-02
Grant ID: R01 NS043095