Abstract
Free full text

Exocytosis of post-Golgi vesicles is regulated by components of the endocytic machinery
Abstract
Post-Golgi vesicles target and deliver most biosynthetic cargoes to the cell surface. However, the molecules and mechanism involved in fusion of these vesicles is not well understood in mammalian cells. We have employed a system to simultaneously monitor release of luminal and membrane biosynthetic cargoes from individual post-Golgi vesicles. Exocytosis of these vesicles is not calcium-triggered and can result in complete, partial or no release of membrane cargo. Partial or no release of membrane cargo of a fusing vesicle are fates associated with kiss-and-run exocytosis and are the predominant mode of post-Golgi vesicle exocytosis. Partial cargo release by post-Golgi vesicles occurs due to premature closure of the fusion pore and is modulated by the activity of clathrin, actin and dynamin. Our results demonstrate that the components of the endocytic machinery modulate the nature and extent of secretion of biosynthetic cargo by affecting fusion of post-Golgi vesicles to the cell membrane.
Introduction
Eukaryotic cells use vesicles to carry newly synthesized lipids and proteins to and across the cell membrane. Secretory biosynthetic cargo is made in the ER, traffic through the Golgi and are packaged in vesicles that are transported to and fuse with the cell membrane, delivering their contents (Palade, 1975). Some of these vesicles (post-Golgi vesicles) fuse shortly after arriving at the cell surface (constitutive exocytosis) and other vesicles (synaptic and dense core vesicles) remain there until a transient rise in calcium triggers their fusion (regulated exocytosis). Regulated secretion involves control over formation and expansion of the fusion pore by regulators such as Ca2+ level (Ales et al., 1999; Elhamdani et al., 2006; Katz, 1971), synaptotagmin (Wang et al., 2001; Wang et al., 2006; Jaiswal et al., 2004), complexin (Archer et al., 2002; Barclay et al., 2005), Munc18 (Barclay et al., 2004; Barclay et al., 2005) and PIP kinase Iγ (Gong et al., 2005).
It is known that a rapid influx of Ca2+ is not needed to trigger constitutive exocytosis (Miller and Moore, 1991; Edwardson and Daniels-Holgate, 1992; Lew and Simon, 1991). Thus it is believed that release of cargo by post-Golgi vesicle exocytosis is not regulated by controlled formation and expansion of the fusion pore. However, while rapid Ca2+ influx does not trigger post-Golgi vesicle exocytosis, it is possible that efflux of calcium from the lumen of the post-Golgi vesicle may control formation and expansion of the fusion pore trigger during post-Golgi vesicle fusion. This has been observed in endosomes (Mayorga et al., 1994; Holroyd et al., 1999), yeast vacuoles (Peters and Mayer, 1998), ER to Golgi and intra-Golgi carriers (Chen et al., 2002; Porat and Elazar, 2000). Moreover, mechanisms other than calcium increase, such as spontaneous reversal of fusion (Stevens and Williams, 2000) and the action of endocytic machinery (Holroyd et al., 2002; Newton et al., 2006; Graham et al., 2002) could regulate fusion pore of exocytic post-Golgi vesicles. Thus there is a need to investigate if Ca2+-dependent or independent mechanisms controls formation and expansion of the post-Golgi vesicle fusion pore.
Two features that have enabled studying the regulation of cargo release by formation and expansion of fusion pore during regulated exocytosis are 1) the ability to synchronize fusion by Ca2+ increase and 2) use of fluorescently tagged luminal and membrane cargoes. As no triggering mechanism is known for post-Golgi vesicle exocytosis, in mammalian cells this synchrony has been achieved by altering growth temperature to block biosynthetic cargo traffic and then reverting to normal temperature to release the block. However, temperature not only affects fusion pore formation and expansion (Zhang and Jackson, 2008) it also affects distribution of lipids and dynamics of transport throughout the secretory pathway (Patterson et al., 2008). While constitutive secretion of biosynthetic membrane cargo has been monitored, secretion of luminal cargo from individual post-Golgi vesicle has not been monitored. To study formation and expansion of fusion pore during post-Golgi cargo secretion other approaches to synchronize, label and monitor exocytosis of post-Golgi vesicles are needed.
We had previously developed an approach to synchronize secretion of constitutive biosynthetic cargo by using a cell-permeable pharmacological regulator, instead of temperature shift (Rivera et al., 2000). This approach allows controlling trafficking of only the desired biosynthetic cargo (membrane or luminal) with no effect on any other cargo. It is thus well suited to study formation and expansion of fusion pore during post-Golgi cargo secretion. Here we have used this approach together with total internal reflection fluorescence microscopy (TIRFM) to simultaneously image secretion of luminal and membrane cargo by individual post-Golgi vesicles in live cells. We found that post-Golgi vesicles can undergo partial (kiss-and-run) exocytosis, resulting in incomplete release of luminal cargo and partial or no release of membrane proteins. Ca2+ had no detectable effect on triggering post-Golgi vesicle fusion nor does it affect the nature of post-Golgi vesicle exocytosis. Instead, clathrin, dynamin and actin - known regulators of endocytosis, control the nature and extent of post-Golgi vesicle exocytosis. Our results identify kiss-and-run exocytosis as a significant regulator of the extent of biosynthetic cargo secretion by post-Golgi vesicles. It also provides evidence that this control is achieved via the activity of the endocytic machinery.
Results
Synchronization of biosynthetic cargo secretion
To synchronize the delivery of biosynthetic cargo to the surface, we tagged the cargo of interest with mutant version of protein FKBP12 (F36M) (Rivera et al., 2000; Rollins et al., 2000). This protein domain homo-dimerizes, which is reversible by a small cell-permeant molecule AP21988 and is thus termed conditional aggregation domain (CAD). Following synthesis in the ER, proteins with multiple CADs self-aggregate and are retained there until ligand addition causes the cargo to disaggregate and traffic through the secretory pathway (Fig. 1A) (Rivera et al., 2000). As a marker for luminal cargo we expressed human growth hormone signal sequence (hGHss) fused to GFP, 4 CADs and hGH itself. Our membrane marker was nerve growth factor receptor (NGFR), which we have previously used to mark the post-Golgi vesicle membrane (Kreitzer et al., 2003), expressed with a fluorescent protein and CADs (Fig. 1B).

(A) Schematic showing two pathways for cell-surface delivery of biosynthetic cargo. In each of these pathways places where cargo trafficking can be regulated by calcium are marked. Misfolding or aggregation of proteins or chelating cytosolic calcium blocks cargo traffic at this step. During regulated-exocytosis, calcium increase due to influx from across the plasma membrane channels and efflux from the vesicle triggers exocytosis. In constitutive exocytosis of post-Golgi vesicles, entry of calcium through plasma membrane channels is not required for exocytosis but, it is not known if the calcium released from docked post-Golgi vesicle triggers its exocytosis. (B) Schematic showing different domains of the luminal and membrane cargoes used in this study. (C) Epi-fluorescence (left) and phase contrast images (right) of a cell with the luminal cargo (hGHss-GFP) at various time points following the addition of AP21988.
Within a few minutes of adding the CAD-disaggregating molecule AP21988 to the culture media, the fluorescence of the luminal cargo moved from the reticular distribution of the ER to a perinuclear distribution of the Golgi and then moved peripherally in small vesicles (Rivera et al., 2000) (Fig. 1C; video 1). Within 15 minutes of ligand addition we detected individual fluorescent vesicles at the cell membrane by total internal reflection fluorescence microscopy (TIRFM) (Data not shown). To determine if the fluorescent marker was in post-Golgi vesicles we simultaneously expressed either the LDL-receptor or VSVGts, as fusions to YFP (Schmoranzer et al., 2000). In cells at 20°C LDLR is retained in the TGN while at 40°C, VSVGts is retained in the ER. Both proteins traffic via post-Golgi vesicles upon shifting cells to 37°C (Schmoranzer et al., 2000). The CFP-tagged luminal or membrane marker co-localized with the vesicles carrying YFP-tagged LDLR or VSVGts (correlation coefficient 0.79 and 0.80 respectively; Fig. S1A, B, E, G). This indicated that the cell membrane-proximal vesicles carrying the CAD-containing fluorescent proteins were bona-fide post-Golgi vesicles.
Post-Golgi vesicles undergo kiss-and-run exocytosis
To monitor secretion of cargo from these post-Golgi vesicles, cells expressing luminal marker (hGHss-GFP-CAD-hGH) were imaged by TIRFM. During transit through Golgi, this protein is cleaved by the Golgi specific protease furin (Fig. 1B). Thus, the fluorescent vesicle luminal marker is hGH signal sequence fused to GFP-CAD (hGHss-GFP; the hGH itself remains non-fluorescent) (Rivera et al., 2000). The fluorescence of these vesicles increase as they entered the evanescent field, and then drops rapidly to background level as it spreads laterally, indicating cargo exocytosis (Fig 2A). We also observed exocytic events where vesicle fluorescence decreased, but remained above the background (Fig 2B). This observation is consistent with the vesicle transiently opening its fusion pore to the outside, releasing only a part of its cargo. Notwithstanding the fact that most of these vesicles do not move following fusion, the term “kiss-and-run fusion” is used to describe such partial fusion fates (Harata et al., 2006). We will thus refer to these fusion fates as kiss-and-run fusion. To test if these partially fusing vesicles open a transient fusion pore we used FM 4–64 dye, which can access the vesicle membrane only when it contacts the extracellular media and has been used to test kiss-and-run fusion of synaptic and other vesicles (Harata et al., 2006). Indeed, FM 4–64 labeled many GFP containing post-Golgi vesicles (Fig. 2C). Similarly, vesicles got labeled with the fluid phase marker Alexa fluor 546-hydrazine (not shown).
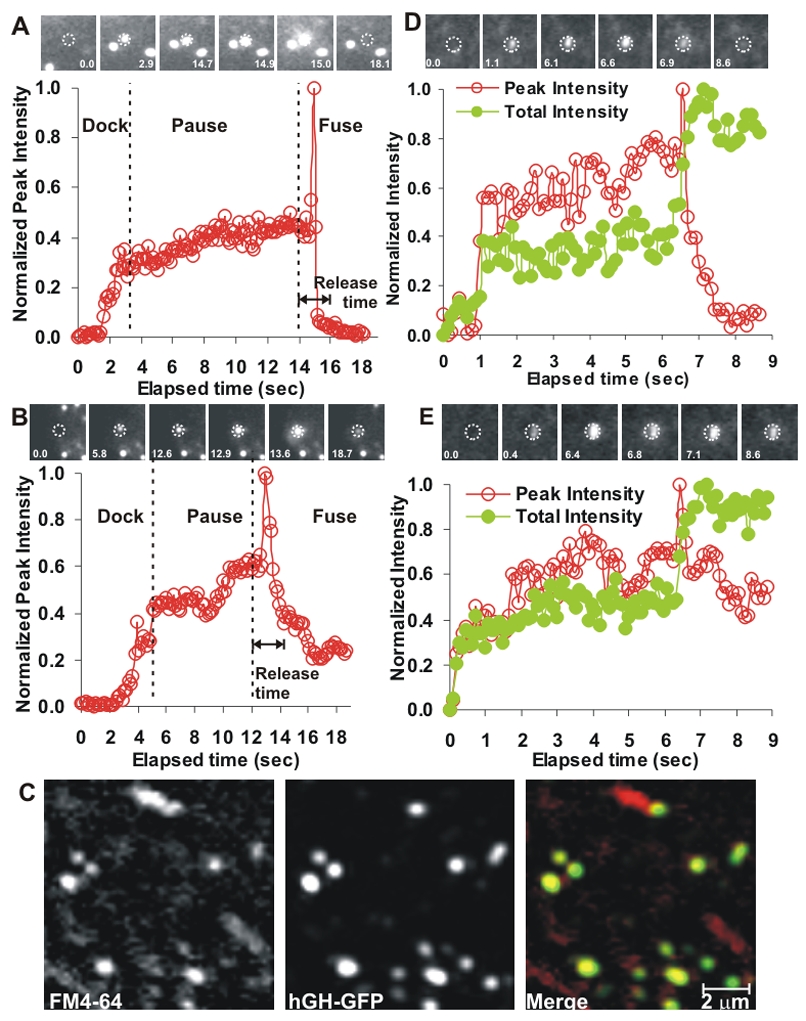
Exocytic vesicles are monitored using (A–C) luminal marker hGHss-GFP and (D, E) membrane marker NGFR-GFP. (A, D) Complete release and (B, E) partial (kiss-and-run) release of the cargoes. The dotted circle marks pixels whose intensity was unchanged as the vesicle docked. The plots show fluorescence within the dotted circle (open symbols) and of the entire field (filled symbols). Intensity is normalized after subtracting background prior to the appearance of the vesicle. Based on the measurement of vesicle fluorescence, vesicle was considered to be tethered/docked at the point after appearing in the evanescent field when its fluorescence was relatively stable. Priming marks the period between docking and initiation of fusion, which is indicated by rapid increase in vesicle fluorescence followed by the cargo release. As total fluorescence of the membrane cargo in D and E remain unchanged after release of the cargo from the vesicles, it indicates that the proteins from the vesicle membrane are delivered to the cell membrane and not lost in the cytosol (as would occur if the vesicle had lysed). (C) A cell expressing hGHss-GFP (green) was treated for 20 minutes with AP21988, followed by 10 minutes in FM4–64 (red). Image is of a part of this cell showing many vesicles labeled with both these markers.
The ability of extracellular markers to enter the lumen of the vesicle indicates that partial release of vesicle luminal marker is due to a transient opening to the outside. However, it does not rule out the possibility that this may involve photodamage to the cell and vesicle membrane causing leakage and mixing of luminal content. To resolve between fusion, leakage and lysis we monitored the fate of vesicle membrane proteins during exocytosis. Only when a vesicle exocytoses will its membrane protein be delivered to the cell surface where it diffuses in two dimensions, thus any decrease in vesicle fluorescence will cause a concomitant increase in cell membrane fluorescence resulting in no change in integrated fluorescence. In case of leakage the membrane marker would remain with the vesicle and not diffuse in the cell membrane, while in case of lysis it will diffuse in the cytoplasm in three dimensions (Jaiswal and Simon, 2007; Jaiswal et al., 2007; Schmoranzer et al., 2000). Following ligand addition, CFP tagged CAD-NGFR trafficked to the post-Golgi vesicles together with YFP tagged CAD-hGH (Fig. S1C, D). Based on the fluorescence of the vesicle membrane marker we observed two types of exocytic behaviors. In some cases the fluorescence of the vesicular membrane protein reduced to the pre-docking background (Fig. 2D red symbol), indicating complete release. In other cases the fluorescence of the vesicular membrane protein decreased, but remained above the pre-docking background (Fig. 2E red symbol), indicating partial release. In each of the observed fates of the vesicle (Fig. 2D, E), the integrated (sum of vesicular and cell membrane) fluorescence of the fusing vesicle’s membrane protein remained high (green symbols) even when there was a decrease in the luminal fluorescence (red symbols). This confirms that these events involve merger of the vesicle and cell membrane and thus indicate exocytosis, not leakage or lysis.
To quantify the number of post-Golgi vesicles undergoing partial or complete fusion, we monitored the release of the luminal cargo or membrane cargo following the addition of CAD disaggregating ligand. Based on luminal contents (hGHss-GFP), we observed 32.7±8.0 fusions/cell in a minute of which 73% (23.8±7.1) were kiss-and-run fusion and the rest 8.9±2.9 were complete fusions (Fig. 3A gray bars). However, using membrane cargo (LNGFR-GFP) we observed 12.2±2.2 fusions/cell in a minute of which 17% (2.1±0.8) were kiss-and-run fusions and the rest 10.1±2 were complete fusions (Fig. 3A black bars). While the difference in number of complete fusions reported by luminal and membrane markers is not statistically significant (p value = 0.4), there is 11-fold difference in the number of vesicles that partially released their content (p value = 10−6).
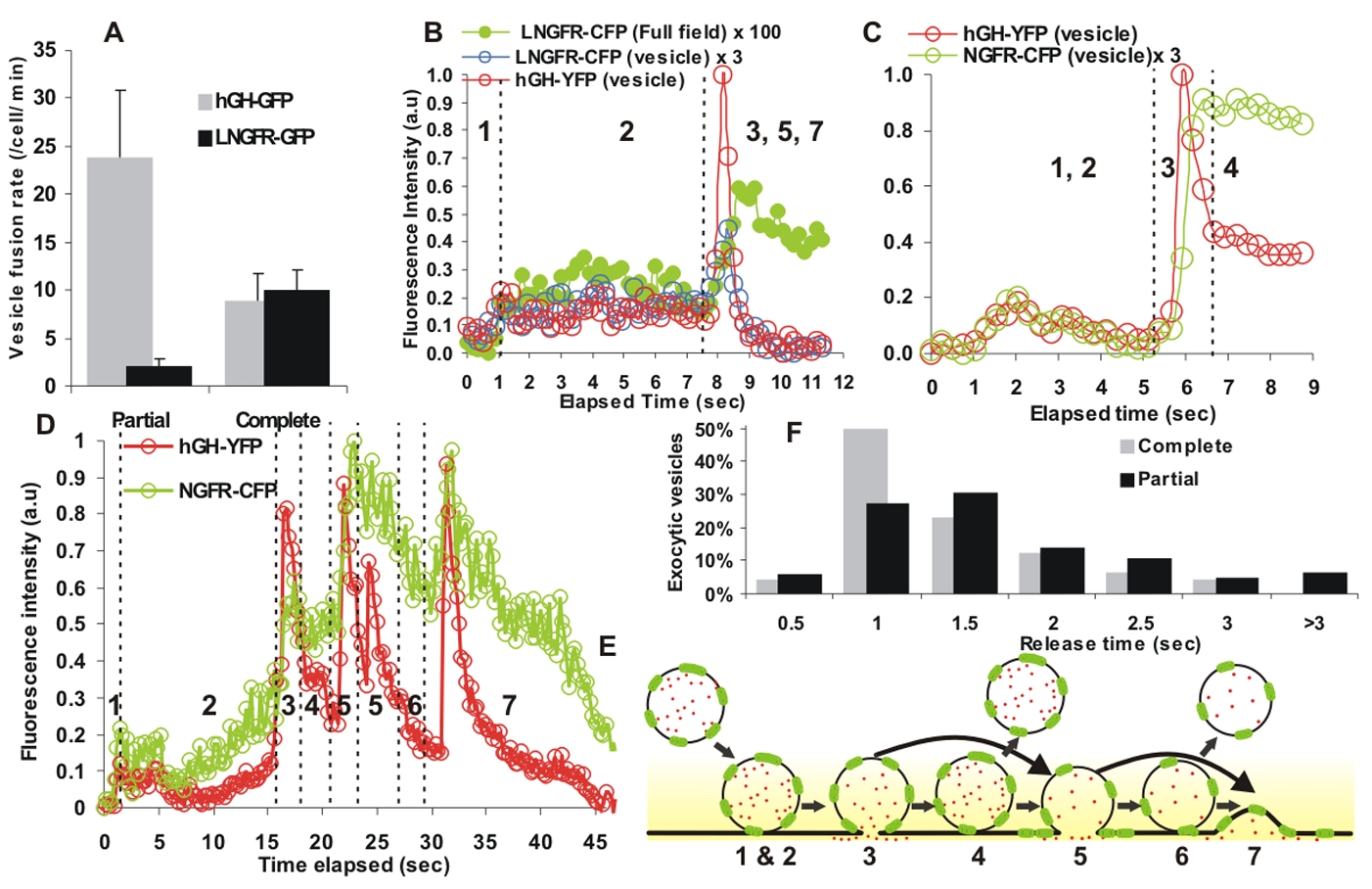
(A) Vesicles undergoing partial (kiss-and-run) or complete exocytosis were measured for one minute in cells expressing only luminal (n = 378 vesicles from 9 cells) or only membrane (n = 135 vesicles from 6 cells) markers. The error bars represent standard deviation. (B) The vesicle labeled with both, luminal (hGH-YFP) as well as membrane (NGFR-CFP) marker (supplementary figure 2A) that undergoes complete fusion. Open symbol shows vesicle-associated fluorescence and filled symbol shows fluorescence of the entire field of view. (C) A dual labeled vesicle that exocytoses to partially release the luminal cargo (red symbol), but does not deliver any membrane cargo to the cell surface (green symbol). Vesicle is shown in figure S2B. (D) Total fluorescence of luminal and membrane cargo for a vesicle that undergoes multiple partial fusions prior to complete fusion. This vesicle is shown in figure S2C and a schematic for the various fusion stages are marked by numbers as described in figure E. (E) Schematic showing states of the vesicle imaged in D – 1&2: docking and priming. 3: first fusion with the cell membrane resulting in partial release of luminal marker (rapid increase and decrease in YFP fluorescence) and no release of the membrane marker (increase and no subsequent decrease in the vesicle associated CFP fluorescence). 4: A period where none of the two markers were released from the vesicle. 5: Fusion where the luminal and membrane markers are released partially albeit to different extents. 6: A period when both the markers are retained by the vesicle. 7: Complete release of luminal and membrane cargoes resulting in return of their vesicle associated fluorescence to pre-docking background. Membrane cargo is marked in green and luminal cargo in red, the shaded yellow region depicts the evanescent field. (F) Release time for hGHss-GFP was quantified for vesicles that underwent partial or complete fusion in five cells (n = 169 vesicles). Release time denotes the period during which vesicle fluorescence increased and reached a post-fusion plateau (see Fig. 2).
Above results are consistent with a population of vesicles that undergo kiss-and-run fusion resulting in partial release of their luminal cargo but no release of the membrane cargo. To test this prediction we co-expressed the membrane and luminal markers as CFP and YFP tagged proteins. Both these cargoes were sequestered in the ER and AP21988 addition caused both cargoes to package in the same post-Golgi vesicles (Fig. S1C, D; Fig. S2 A–C).
Quantification of the vesicle and cell membrane-associated fluorescence indicates vesicles that fully released their YFP-tagged luminal cargo (Fig 3B, red line) also fully released their CFP-tagged membrane marker cargo (Fig. 3B open blue circles) and the released membrane cargo is delivered to the cell membrane: The integrated fluorescence of the vesicle and cell membrane remained relatively constant after exocytosis (Fig. 3B green line, filled symbols). Thus vesicle luminal and membrane cargoes behaved similarly when co-expressed or expressed independently – their fluorescence returned to pre-fusion level (Figs. 2A and 2D). Vesicles partially releasing their luminal content delivered either little or no membrane protein (Fig. 3C–E, Fig. S2B–C). The fusion events where luminal cargo is partially released and membrane cargo is not released (state 3 in Fig. 3E) account for the reduced number of partial fusions when only the vesicle membrane protein was used as exocytic marker (Fig. 3A).
Vesicles that fuse partially without releasing membrane cargo can subsequently fuse with partial or complete release of the membrane cargo (Fig. 3D). We confirmed that the fluorescence signals we observed originated from an individual fusing vesicle (Fig. S2 D–E). Above results indicate that the nature of vesicle fusion is regulated dynamically. To examine if restriction of fusion pore (during kiss-and-run fusion) slows release of luminal content, we compared the time between start and end of luminal cargo release by individual exocytic vesicles from five cells. Cargo released faster during complete fusions (1.11 ± 0.30 s) compared to kiss-and-run fusions (1.49 ± 0.36 s) (p value = 0.01; Fig. 3F).
It has been previously reported that dense core vesicles (DCVs) can partially release their luminal contents together with release of some but not other membrane proteins (Tsuboi et al., 2004). Thus, incomplete release of membrane proteins could reflect a limitation of the reporter protein used and not the nature of the fusion. However, our vesicle membrane marker (NGFR-GFP) can be retained or fully released by the same vesicle (Fig. 3D) and our observation that ~ 17% (2/12; Fig. 3A) post-Golgi vesicles labeled by this marker undergo kiss-and-run fusion is in agreement with the results of 14% previously reported using LDLR-GFP (Schmoranzer and Simon, 2003). Together, these results suggest that the incomplete release we observe is not due to a limitation of our reporter. Instead, it is consistent with post-Golgi vesicles partially releasing their contents similar to the kiss-and-run fusion proposed for synaptic, dense core and other regulated exocytic vesicles (Harata et al., 2006).
Ca2+ does not trigger nor regulate the nature of post-Golgi vesicle exocytosis
Unlike regulated exocytic vesicles, exocytosis of post-Golgi vesicles is unaffected when extracellular Ca2+ is chelated using EGTA (Lew and Simon, 1991). This indicates that Ca2+ influx is not required for post-Golgi vesicle exocytosis, but does not rule out that transient localized Ca2+ microdomains formed by the efflux from the docked post-Golgi vesicle may trigger its exocytosis. Such a mode of Ca2+-triggering has been identified for other membrane fusion reactions (Peters and Mayer, 1998). To test this possibility we used two reagents - ionomycin to rapidly discharge vesicular Ca2+ (Williams et al., 1985) and BAPTA-AM to rapidly chelate cytosolic Ca2+ and prevent the formation of Ca2+ microdomains (Peters and Mayer, 1998). Increasing Ca2+ by ionomycin triggered lysosomal exocytosis in HT1080 cells as we have previously observed for other cells (Jaiswal et al., 2002). BAPTA-AM prevents Ca2+-microdomain formation which triggers yeast vacuole fusion (Peters and Mayer, 1998). Using the Ca2+-sensitive ratiometric dye pair fura-red and fluo-3 we found that both these agents stably altered the cytosolic Ca2+ within 10 sec (Fig. 4A). Calcium is required for trafficking of biosynthetic cargo from ER to Golgi (Beckers and Balch, 1989) and this treatment reduced the number of fluorescently-labeled post-Golgi vesicles that newly appeared in the TIR field (video 2). Thus, assaying total cargo released from cells with altered cytosolic calcium cannot distinguish between effects of Ca2+ on vesicle exocytosis and effects on transport through the biosynthetic pathway.
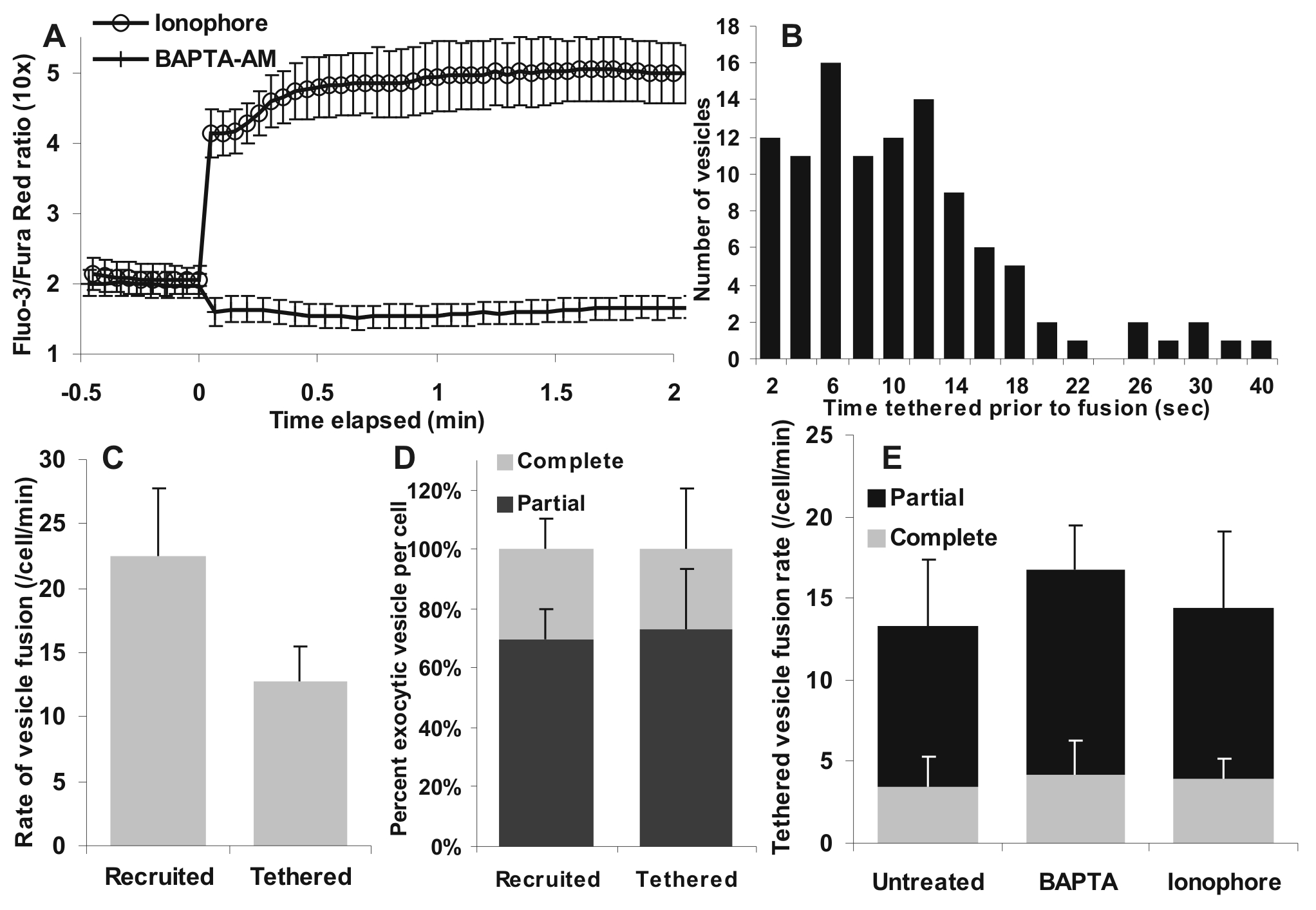
(A) Six HT1080 cells labeled with Fura-red and Fluo-3 and were imaged using epifluorescence excitation starting 30s prior to the addition of calcium altering agents and their averaged fluorecen is plotted. (B) Cells expressing luminal cargo hGHss-GFP were imaged by TIR-FM and the time for which the exocytic vesicles remained tethered at the cell surface prior to fusion was measured for 107 vesicles. (C) Rate of exocytosis of 450 hGHss-GFP vesicles from 10 cells was quantified, those present in the TIR field at the start of imaging were termed as tethered/docked, rest were classified as recruited. (D) Exocytic fate of the tethered and newly recruited vesicles. (E) Tethered post-Golgi vesicles that underwent exocytosis were quantified in nine cells each that were treated for 20–40 minutes with AP21988 only (untreated) or with AP21988 followed by 100μM BAPTA-AM (for 5 min) or 10μM ionomycin.
To resolve if calcium affected steps after transport, we took advantage of the observation that most post-Golgi vesicles pause prior to fusion, as if briefly tethered to the cell membrane (step 2 in Fig. 3E; Fig. 4B n = 107) (Schmoranzer et al., 2000). Exocytosis of these cargo carrying post-Golgi vesicles is independent of effects at the step of cargo packaging. Thus, we monitored the exocytosis of membrane tethered post-Golgi vesicles to test for effects of calcium release from their lumen on exocytosis. In cells where calcium has not been altered, over one-third of all vesicles that exocytose during a two minute imaging period were tethered at the cell surface at the start of imaging (Fig. 4C). Vesicles that were tethered or freshly recruited were equally likely to partially (or completely) release their contents (Fig. 4D). We next quantified the effect of transient treatment of ionomycin or BAPTA-AM on exocytosis of tethered vesicles (Fig. 4E). Neither chelating the cytosolic Ca2+ (with BAPTA-AM) nor increasing it (with ionomycin) affected the rate of exocytosis of post-Golgi vesicles (Fig. 4E p value = >0.4 for both treatments). To test if the acidic lumen of secretory vesicles was hindering the ionomycin-induced release of calcium, we treated cells with ionomycin in the presence of 25 mM NH4Cl or 100 μM of the Na+/H+ exchanger monensin. Neither agent alone or together with ionomycin had any affect on the rates of post-Golgi vesicle fusion (data not shown). These results indicated that post-Golgi vesicle exocytosis is insensitive to calcium influx and insensitive to any local Ca2+ efflux from the secreting vesicle. To test if Ca2+ affects the nature of post-Golgi vesicle fusion we monitored post-Golgi vesicle exocytosis in BAPTA and ionomycin treated cells. We detected no changes in the rate of partial or complete release of cargo by either treatment (Fig. 4E; p values between 0.2 – 0.8). This ruled out a role of Ca2+ or Ca2+-dependent exocytic machinery in regulating the choice between kiss-and-run and complete fusion of post-Golgi vesicles.
Endocytic machinery affects the nature of Post-Golgi vesicle exocytosis
We examined if clathrin, actin and dynamin, proteins that control membrane curvature, bending and fission during endocytosis, affect kiss-and-run fusion of post-Golgi vesicles. We immunostained the cells treated with AP21988 (to allow the hGHss-GFP to traffic into the post-Golgi vesicles) and for endogenous clathrin light chain. Half of all the vesicles analyzed (n=245) from four cells, contained a detectable endogenous clathrin signal (Fig. 5A, Fig. S3A). To determine if this co-localization was functionally associated with the nature of vesicle fusion we simultaneously imaged luminal post-Golgi cargo (hGHss-GFP) and dsRed-clathrin light chain (CLC) in live cells (video 3). Expression of dsRed-CLC had no effect on the nature of the secretion of luminal cargo hGHss-GFP (data not shown). A majority (71 out of 118) of the exocytic post-Golgi vesicles containing hGHss-GFP were also labeled with dsRed-CLC (vesicle marked with circle in video 3, Fig. 5B, C) and 79% of such clathrin labeled vesicles underwent kiss-and-run fusion (Fig. 5D). Clathrin was present on the vesicles prior to docking. In some of the vesicles CLC-dsRed did not dissociate even after fusion (Fig. 5B), in others it dissociated following fusion (Fig. 5C). Of the vesicles with no detectable dsRed-CLC (vesicle marked with square in video 3; Fig. 5D), 83% completely released their contents (Fig. 5E). To test if clathrin affects post-Golgi vesicle exocytosis, we reduced the expression of clathrin heavy chain (CHC) and CLC by over 80% (Fig. 5F) using a previously described siRNA (Hinrichsen et al., 2003).. With this siRNA, the cells had very little punctate CLC staining and some residual perinuclear staining (Fig. 5F, lower panel), consistent with previous reports (Hinrichsen et al., 2003). The cells showed a nearly two-fold increase (16 ± 4 v/s 8.9 ± 2.9; p value = 0.001) in complete fusions and increase in total rate of fusion from 32.7 ± 8 to 44.2 ± 10.1(p value = 0.02) (Fig. 5G). However, there was no change in the rate of kiss-and-run fusion (p value = 0.3). As there is a broad distribution (from <20% to <100%) of the amount of luminal cargo released by vesicles undergoing kiss-and-run fusion, we tested the effect of CHC knockdown on this distribution. In CHC siRNA treated cells there are more vesicles in all bins where cargo released is < 80% (Fig. 5H). However, the number of vesicles that release between 80% to <100% of their cargo decreased ~3-fold. This shows that knockdown of CHC affects vesicles undergoing partial fusion such that some vesicles with no detectable release of their content (non-exocytic vesicles) in untreated cells now release between 20–80% of their content, causing an increase in these bins. But, vesicles that release between 80% to <100% of their content now release all of their content (complete release), causing a decrease in <100% bin. Thus through its effect on vesicles that normally do not fuse and fuse partially, CHC knockdown causes an increase in complete and total fusion.
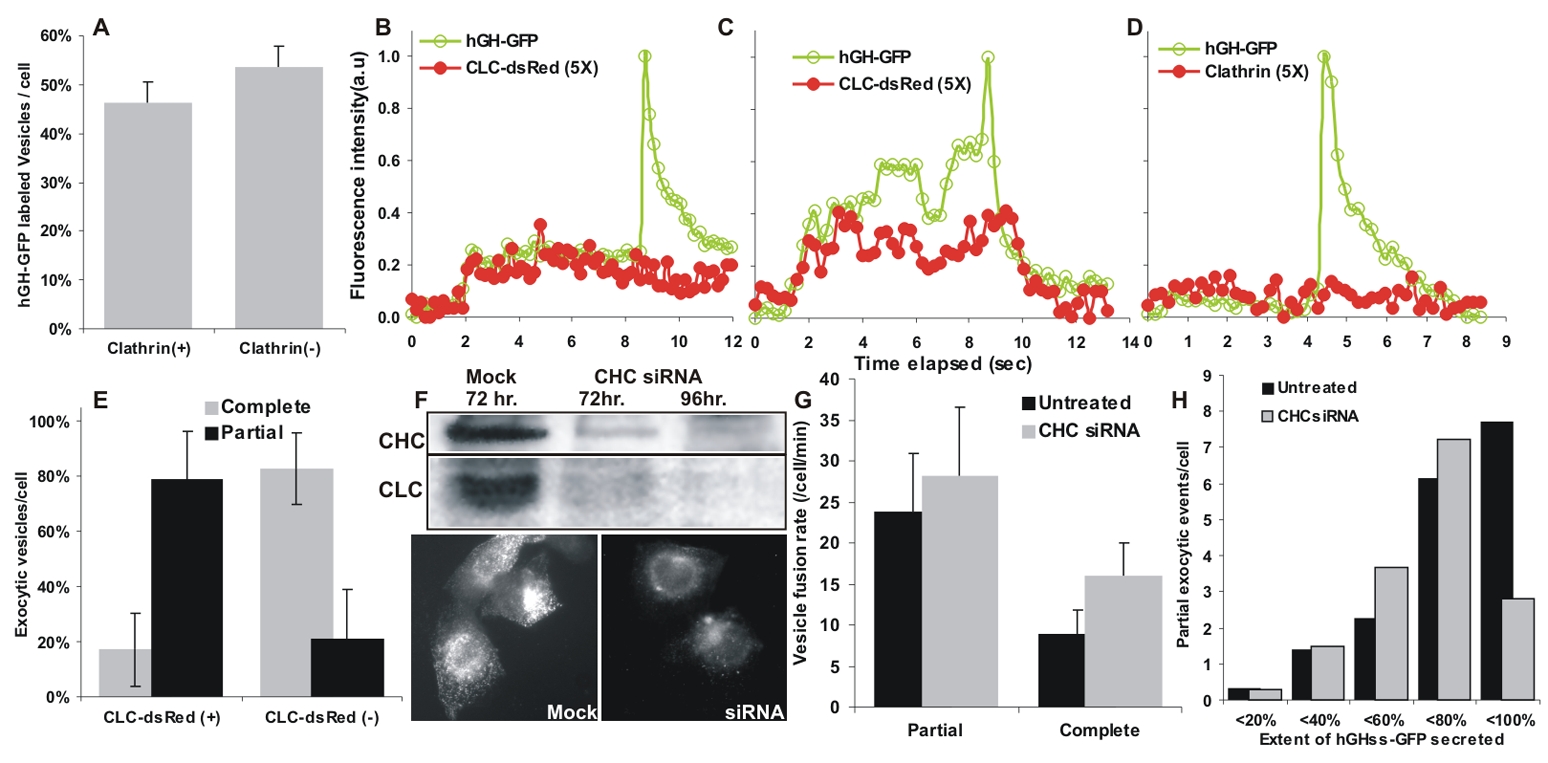
(A) Cells treated with AP21988 to label vesicles with hGHss-GFP were fixed and immunostained for CLC and all (245) GFP-labeled vesicles were scored for the presence of clathrin. (B–D) 118 hGHss-GFP labeled vesicles from 4 cells expressing dsRed-CLC were imaged to assess the presence of dsRed-CLC and the extent of GFP they secreted. (B–D) Change in dsRed-CLC signal associated with vesicles that undergo (B, C) kiss-and-run or (D) complete fusion (E) A plot showing secretory fates of exocytic vesicles that are (+) or are not (−) labeled with dsRed-CLC. (F) Efficacy of siRNA-mediated knockdown of clathrin examined by immunoblotting and immunofluorescence. (G) Effect of clathrin knockdown on the exocytic fate of 543 and 565 hGHss-GFP vesicles respectively from nine each of untreated and CHC siRNA treated cells. (H) Extent of hGHss-GFP secreted by vesicles undergoing kiss-and-run fusion in untreated and CHC-siRNA treated cells.
We next examined the association of dynamin with post-Golgi vesicles. Thirty minutes after the release of the luminal cargo from the ER, cells were immunostained for endogenous dynamin. Dynamin was only occasionally present on the post-Golgi vesicles at the cell surface (Fig. S3B). To test if dynamin associates with vesicles at the time of fusion we expressed dynamin2(aa)-mCherry and monitored its distribution in live cells. The signal/noise for dynamin was weaker than for dynamin at endocytic vesicles, so we averaged signals from multiple events. A rapid (<1 sec) burst of dynamin occured at the site of fusion of vesicles that released their cargo partially (Fig. 6A, 27 events) and no detectable increase in dynamin occurred for vesicles that released their cargo completely (Fig. 6B, 29 events). To test for a functional consequence on exocytosis, we blocked dynamin activity. Dynamin activity is required for budding of biosynthetic cargo carrying vesicles from the TGN (Cao et al., 2000; Kreitzer et al., 2000). This prevented the use of agents that chronically block dynamin activity (dynamin siRNA, dominant negative dynamin mutants or function-blocking antibodies) to test the role of dynamin in regulating the fusion step for post-Golgi vesicles. This requires transient and rapid block of dynamin activity, for which we used dynasore – a cell-permeant small molecule inhibitor of dynamin’s GTPase activity (Macia et al., 2006). Consistent with previously reported effects of a chronic block in dynamin function, treatment of cells expressing CAD-tagged hGHss-GFP with 80μM dynasore for 10 minutes fully blocked endocytosis of transferrin (Fig. 6C) and prevented release of cargo from the Golgi (Fig. 6D). At the concentrations and duration we used, dynasore had no detectable effect on cell viability based on trypan blue dye exclusion (Bess and Simon unpublished). Moreover, the effect of dynasore was reversible: following dynasore washout, luminal cargo containing post-Golgi vesicles reappeared at the cell surface and fused in a manner that was indistinguishable from its behavior prior to dynasore treatment (video 4). To assess the role of dynamin in regulating the post-Golgi vesicle fusion pore, we allowed the luminal cargo (hGHss-GFP) to be packaged into post-Golgi vesicles for 20 minutes and then 10 minutes after adding dynasore we analyzed the fate of cell membrane tethered vesicles. Cells treated with dynasore had a three-fold increase in the number of fusions that resulted in complete release of cargo (9.9 ± 4 v/s 3.4 ± 1.9; p value = 0.001) and a similar decrease in the number of partial release events (3.4 ± 2 v/s 9.8 ± 4.1; p value = 0.0005) (Fig. 6E), indicating that the GTPase activity of dynamin regulates kiss-and-run fusion of post-Golgi vesicles.
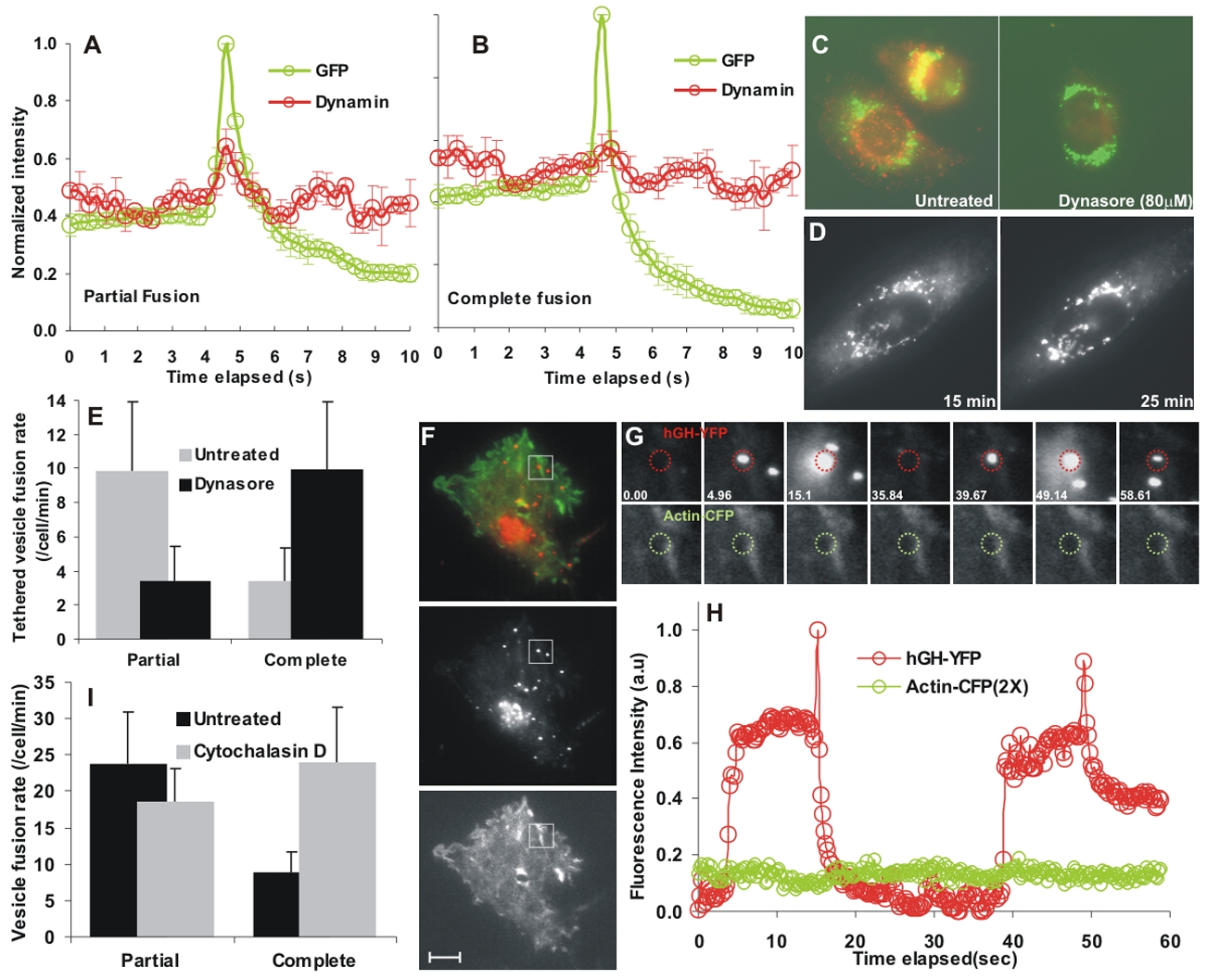
(A, B) Luminal cargo hGHss-GFP and dynamin2(aa)-mCherry were simultaneously imaged by TIRFM in AP21988-treated cells. Averaged intensity traces for GFP and mCherry for (A) 29 vesicles undergoing kiss-and-run fusion and (B) 27 vesicles undergoing complete fusion. Traces were aligned to the point of secretion (peak of hGHss-GFP fluorescence) and the error bars represent SEM. (C) CAD-hGHss-GFP expressing cells treated or not treated for 10 minutes with dynasore were labeled with Tfn and extracellular Tfn was acid washed prior to fixing. Only untreated cells (left) show Tfn-containing vesicles (red). (D) Cells were treated for 20 minutes with 80μM dynasore and AP21988-induced release of hGHss-GFP from the Golgi was monitored continuously; two of these time points are shown. (E) Rate of exocytosis of 121 hGHss-GFP labeled vesicles (from seven cells) that were tethered to the cell surface at the start of imaging was monitored following treatment of cells with AP21988 alone (untreated) or an additional 10 minute treatment with 80μM dynasore. (F) Cell expressing the luminal cargo hGHss-YFP and actin-CFP were imaged by TIRFM following treatment with AP21988. Scale bar -10 μm. (G) The boxed region in F is magnified. Lower panel shows actin and the upper panel shows hGHss-YFP images at the time points indicated. (H) Quantification of YFP and CFP fluorescence from the region in G marked by dotted circle. (I) Rate of luminal cargo exocytosis of in nine cells treated with AP21988 alone (untreated) and with 2μM cytochalasin D.
F-actin mediates dynamin and clathrin-dependent vesicle fission and has been implicated in post-fusion retrieval of Xenopus egg granules (Sokac and Bement, 2006). To investigate if actin assembly contributes to premature closure of the post-Golgi vesicle fusion pore we transiently expressed actin-CFP in cells expressing the luminal cargo (hGHss-YFP) (Fig. 6F). We observed no change in F-actin at the site of fusion irrespective of the nature of release - complete or partial (Fig. 6G, H). Thus, within our detection limits, F-actin buildup at the site of fusion is not required for pinching off and closure of fusion pore during partial fusion. To test if F-actin affects the kiss-and-run fusion of post-Golgi vesicles we treated cells simultaneously with AP21988 and 2μM cytochalasin D for 30 minutes and then imaged exocytosis of hGHss-GFP. Cytochalasin D treatment resulted in over twofold increase in the number of fusions that completely released their cargo (24.0 ± 7.5 v/s 8.9 ± 2.9; p value = 10−5) while the rate of partial fusion remained unchanged (18.5 ± 4.5 v/s 23.8 ± 7.1; p value = 0.2). This caused a small increase in the total rate of fusions (42.6 ± 9.7 v/s 32.7 ± 8.0; p value = 0.03).
Discussion
Imaging the secretion of membrane and luminal cargo simultaneously from individual post-Golgi vesicle has yielded several unexpected observations of the regulation of the secretion of post-Golgi cargo.
We obtained unambiguous evidence for kiss-and-run exocytosis of post-Golgi vesicles and found it is the dominant mode of biosynthetic cargo secretion. The fusing vesicles had various fates including: a) Incomplete release of luminal and membrane contents, b) Incomplete release of luminal and no release of membrane contents, and c) complete release of the luminal and membrane contents.
Kiss-and-run fusion has previously been observed in Ca2+-regulated exocytosis and the molecules implicated in determining this fusion fate regulate the Ca2+-sensitivity of regulated fusion. However, the exocytosis of post-Golgi vesicles is insensitive to both influx of extracellular Ca2+ and efflux of Ca2+ from the vesicle lumen (Fig. 4). These results suggest that either the post-Golgi vesicle exocytic SNAREs are not sensitive to Ca2+ increase or accessory factors make them Ca2+-insensitive. Regardless of the mechanism, our results indicate that Ca2+ does not regulate formation or expansion of the fusion pore during all forms of exocytosis and kiss-and-run fusion of post-Golgi vesicles is not regulated by calcium.
Interfering with the synthesis or activity of clathrin, dynamin and actin, proteins that regulate curvature and fission of vesicle membrane during, affects the nature of post-Golgi vesicle fusion (Figs. 5 and and6).6). Each of these proteins is involved in clathrin mediated endocytosis. However, based on the following observations we conclude that effects of these proteins on fusion is not through their role in clathrin mediated endocytosis:
- Clathrin builds up gradually at the endocytic spot (Merrifield et al., 2002; Rappoport and Simon, 2003), which is not observed at the sites of kiss-and-run fusions. All the clathrin on these vesicles is present before the vesicle appears at the cell surface (Fig. 5B, C, video 3).
- There is no burst of actin around the time of vesicle pinching (Fig 6E–G), as has been shown for endocytic vesicles (Merrifield et al., 2002).
-Following the detachment from the cell membrane, endocytic vesicles rapidly move out of the evanescent field (Merrifield et al., 2005). In contrast, post-Golgi cargo containing vesicles remained at the cell surface, often fusing again, sometimes multiple times, until their cargo is released (Fig. 3D, E).
Thus, the modulation of fusion fate of post-Golgi vesicles appears to be a result of premature termination of fusion due to a direct role of clathrin, dynamin and actin in regulating the exocytic machinery rather than a competition between exo- and endocytosis. Direct regulation of fusion by clathrin and dynamin could involve binding and sequestering SNAREs, resulting in inefficient fusion (Antonny, 2004). Such a role has been demonstrated for the dynamin homolog (Vps1p) in yeast (Peters et al., 2004). However, regulation by only binding and sequestering SNAREs cannot explain the observed change in the nature of fusions caused by a rapid block in the dynamin GTPase activity (Fig. 6E). A mechanochemical mode of action of dynamin in regulating fusion pore has been suggested for DCVs according to which kiss-and-run fusion of DCVs is regulated by dynamin but not clathrin (Tsuboi et al., 2004). However, our results demonstrate that clathrin and dynamin both play a role in restricting the expansion of the post-Golgi vesicle fusion pore. Similarly, clathrin may have a mechanical or mechanochemical role to play, in maintaining vesicle curvature, inhibiting flattening or recruiting molecules that cause scission. Clathrin found on a partially releasing vesicle is present before the vesicle arrives at the cell membrane (Fig. 5B, C). Thus, as has been shown for TGN derived vesicles (Puertollano et al., 2003) post-Golgi vesicles may obtain their clathrin at the TGN.
Disruption of F-actin also increases the rate of complete and total release. This suggests that disruption of clathrin or actin allows those vesicles to fully fuse that are normally unable to fuse or fuse partially. As no assembly of F-actin is detected at the time of fusion, the mechanism by which F-actin disassembly increases complete fusion is plausibly through the effect on disassembly of cortical F-actin. Disassembly of cortical actin could reduce steric hindrance on vesicle fusion and flattening or allow components of the fusion machinery to diffuse more readily in the plasma membrane and better assemble causing increased complete fusions. This is consistent with our previous observations that disrupting actin shortens the time between when a vesicle arrives at the plasma membrane and releases its cargo (Schmoranzer and Simon, 2003).
Our observations are consistent with a model for regulation of post-Golgi vesicle fusion pore expansion involving clathrin, actin and dynamin (Fig. 7). According to this model, clathrin and dynamin prevent complete release by either restricting the expansion of the fusion pore, severing the neck of the fusion pore or both (Fig. 7A). We suggest that restrictions imposed on partially fusing vesicles slow down vesicle flattening such that the fusion pore neck stays open for longer periods. This is consistent with the observation that compared to vesicles that undergo kiss-and-run fusion luminal cargo is released faster from vesicles that undergo complete fusion (Fig 3F). Additionally, clathrin on the vesicle and cortical F-actin act as barriers restricting the ability of a fusion pore to form, thus their absence should increase the total number of fusion events, which is what we observed (Fig. 5F, 6E and I). A longer life of the neck of the fusion pore allows a short burst of dynamin assembly that further restricts the expansion of the fusion pore and/or causes its scission resulting in partial fusion (Fig. 6A, ,7A).7A). However, in case of vesicles where dynamin does not assemble at the neck or when the dynamin activity is blocked release of cargo goes to completion (Fig. 6B, E, ,7C7C).

(A) Presence of clathrin on the vesicle, actin on the cell membrane and dynamin on the neck of the fusing vesicle restricts fusion pore expansion and severing the neck of the vesicle causing partial fusion. Lack of (B) clathrin, (C) functional dynamin or (D) cortical F-actin relieves the restriction on the fusion pore expansion causing an increase in complete fusion. Lack of clathrin and cortical F-actin also minimizes physical barrier to the fusion machinery resulting in increased number of total fusions.
These results raise the question of whether there is a physiological relevance of kiss-and-run fusion of post-Golgi vesicles. One possible role may be to allow rapid retrieval of the Golgi-resident proteins that traffic to the cell surface. Another related role could be allowing the cell membrane to locally regulate the extent of membrane and luminal cargo secreted by a post-Golgi vesicle. As such these results are important for understanding the control over secretion of post-Golgi cargo. Additionally, in view of the ongoing controversy regarding whether kiss-and-run fusion does (Gandhi and Stevens, 2003; Aravanis et al., 2003) or does not (Dickman et al., 2005; Ryan et al., 1996) exist, our work provides a novel system where the regulation of this process can be studied. The interplay we demonstrate between the basal exocytic and endocytic machineries suggests that these processes may have evolved as one instead of two distinct processes for controlling intracellular membrane traffic.
Experimental Procedures
Cell culture and treatments
Human fibrosarcoma cells HT1080 were cultured in DMEM media supplemented with 10% FBS (Invitrogen, Carlsbad, CA) in 5% CO2 at 37° C. For imaging, cells were plated onto glass coverslips (Fisher Scientific, Pittsburgh, PA) or on glass bottom dishes (MatTek, Ashland, MA) and imaged in OptiMEM (Invitrogen, Carlsbad, CA). Cells were transfected using effectene (Qiagen, Valencia, CA) or Lipofectamine 2000 (Invitrogen, Carlsbad CA). Transiently transfected cells were imaged within 72 hours of transfection. To release the cargo from ER aqueous stock solution of AP21988 (1 mM, ARIAD Gene Therapeutics, MA) was diluted in OptiMEM and added to the cells at the final concentration of 2 μM for the desired period prior to imaging. Stocks for FM4–64, BAPTA-AM (Invitrogen) and ionomycin (Sigma Aldrich, St. Louis, MO) were prepared in DMSO and diluted appropriately in OptiMEM for use. For immunodetection we used goat anti-dynamin II polyclonal Antibody (SantaCruz Biotech., Santa Cruz, CA), mouse anti-CLC monoclonal antibody clone CON.1 (Covance Research Products, Denver, PA) and mouse anti-CHC monoclonal antibody clone 23 (BD Biosciences Pharmingen, San Diego, CA). For clathrin knockdown siRNA – AACCUGCGGUCUGGAGUCAAC, described for CHC knockdown in human cells (Hinrichsen et al., 2003) and the siGLO Red transfection control siRNA were obtained from Dharmacon (Chicago, IL). Cells were co-transfected with both these RNAs using Oligofectamine (Invitrogen) and 72 to 96 hours post transfection siGLO labeling was used to identify siRNA transfected cells.
Microscopy and Data Analysis
Through-the-objective TIR-FM and epifluorescence microscopy were performed with an inverted Olympus IX-70 microscope with an APO 60x N.A. 1.45 TIR objective (Olympus Scientific, Melville, NY) equipped with a 12-bit cooled CCD camera (ORCA-ER, Hamamatsu Photonics, Hamamatsu, Japan). The depth of the evanescent field was kept between 100–150 nm. The camera, the Mutech MV1500 image acquisition card (Billerica, MA) and the mechanical shutters (Uniblitz, Vincent Associates, Rochester, NY) were controlled by MetaMorph (Molecular Devices, Downingtown, PA). The microscope was enclosed in a home-built chamber for temperature control and all imaging was performed at 37° C. For TIR-FM, fluors were excited using 442 nm He-Cd, 543 nm He-Ne laser and tunable Argon laser (Melles Griot, Carlsbad, CA). For epifluorescence, Xenon short arc lamp (Ushio Inc., Japan) was used. Emission filters used include 480/40, 515/30 or 525/50, 550/50, 580lp. Dual-color TIR-FM was carried out by simultaneously exciting both fluors and spectrally separated their emission using an emission splitter (Dual-View, Optical Insights, Santa Fe, NM) equipped with band pass filters mentioned above. All filters, dichroics and polychroics were from Chroma Technologies, Brattleboro, VT. Data analysis was performed using MetaMorph software (Molecular Devices, Downingtown, PA). Satistical significance was measured using Students’ T-test and the significance is reported as p values.
Supplementary Material
01
02
03
04
05
06
07
08
Acknowledgments
Thomas Kirschhausen provided us dynasore and DsRed-CLC and Claire Atkinson generated Dynamin2(aa)-mCherry. We thank lab members and others who offered helpful suggestions during the course of this work and Patrick Bhola for comments on the manuscript. Knut Wittkowski helped us with some of the statistical analysis and the work was funded by grants from NSF (BES-0620813) and NIH (P20 GM072015 to SMS and R01AR055686 to JKJ).
Footnotes
Author contributions
JKJ designed the research, performed the experiments, analyzed data and wrote the paper, all with help from SMS. VMR contributed new reagents.Publisher's Disclaimer: This is a PDF file of an unedited manuscript that has been accepted for publication. As a service to our customers we are providing this early version of the manuscript. The manuscript will undergo copyediting, typesetting, and review of the resulting proof before it is published in its final citable form. Please note that during the production process errors may be discovered which could affect the content, and all legal disclaimers that apply to the journal pertain.
References
- Ales E, Tabares L, Poyato JM, Valero V, Lindau M, Alvarez dT. High calcium concentrations shift the mode of exocytosis to the kiss-and-run mechanism. Nat Cell Biol. 1999;1:40–44. [Abstract] [Google Scholar]
- Antonny B. SNARE filtering by dynamin. Cell. 2004;119:581–582. [Abstract] [Google Scholar]
- Aravanis AM, Pyle JL, Tsien RW. Single synaptic vesicles fusing transiently and successively without loss of identity. Nature. 2003;423:643–647. [Abstract] [Google Scholar]
- Archer DA, Graham ME, Burgoyne RD. Complexin regulates the closure of the fusion pore during regulated vesicle exocytosis. J Biol Chem. 2002;277:18249–18252. [Abstract] [Google Scholar]
- Barclay JW, Aldea M, Craig TJ, Morgan A, Burgoyne RD. Regulation of the fusion pore conductance during exocytosis by cyclin-dependent kinase 5. J Biol Chem. 2004;279:41495–41503. [Abstract] [Google Scholar]
- Barclay JW, Morgan A, Burgoyne RD. Calcium-dependent regulation of exocytosis. Cell Calcium. 2005;38:343–353. [Abstract] [Google Scholar]
- Beckers CJM, Balch WE. Calcium and GTP: Essential components in vesicular trafficking between the endoplasmic reticulum and Golgi apparatus. J Cell Biol. 1989;108:1245–1256. [Europe PMC free article] [Abstract] [Google Scholar]
- Cao H, Thompson HM, Krueger EW, McNiven MA. Disruption of Golgi structure and function in mammalian cells expressing a mutant dynamin. J Cell Sci. 2000;113(Pt 11):1993–2002. [Abstract] [Google Scholar]
- Chen JL, Ahluwalia JP, Stamnes M. Selective effects of calcium chelators on anterograde and retrograde protein transport in the cell. J Biol Chem. 2002;277:35682–35687. [Abstract] [Google Scholar]
- Dickman DK, Horne JA, Meinertzhagen IA, Schwarz TL. A slowed classical pathway rather than kiss-and-run mediates endocytosis at synapses lacking synaptojanin and endophilin. Cell. 2005;123:521–533. [Abstract] [Google Scholar]
- Edwardson JM, Daniels-Holgate PU. Reconstitution in vitro of a membrane-fusion event involved in constitutive exocytosis. A role for cytosolic proteins and a GTP-binding protein, but not for Ca2+ Biochem J. 1992;285(Pt 2):383–385. [Europe PMC free article] [Abstract] [Google Scholar]
- Elhamdani A, Azizi F, Artalejo CR. Double patch clamp reveals that transient fusion (kiss-and-run) is a major mechanism of secretion in calf adrenal chromaffin cells: high calcium shifts the mechanism from kiss-and-run to complete fusion. J Neurosci. 2006;26:3030–3036. [Abstract] [Google Scholar]
- Gandhi SP, Stevens CF. Three modes of synaptic vesicular recycling revealed by single-vesicle imaging. Nature. 2003;423:607–613. [Abstract] [Google Scholar]
- Gong LW, Di Paolo G, Diaz E, Cestra G, Diaz ME, Lindau M, De Camilli P, Toomre D. Phosphatidylinositol phosphate kinase type I gamma regulates dynamics of large dense-core vesicle fusion. Proc Natl Acad Sci U S A. 2005;102:5204–5209. [Abstract] [Google Scholar]
- Graham ME, O’Callaghan DW, McMahon HT, Burgoyne RD. Dynamin-dependent and dynamin-independent processes contribute to the regulation of single vesicle release kinetics and quantal size. Proc Natl Acad Sci U S A. 2002;99:7124–7129. [Europe PMC free article] [Abstract] [Google Scholar]
- Harata NC, Aravanis AM, Tsien RW. Kiss-and-run and full-collapse fusion as modes of exo-endocytosis in neurosecretion. J Neurochem. 2006;97:1546–1570. [Abstract] [Google Scholar]
- Hinrichsen L, Harborth J, Andrees L, Weber K, Ungewickell EJ. Effect of clathrin heavy chain- and alpha-adaptin-specific small inhibitory RNAs on endocytic accessory proteins and receptor trafficking in HeLa cells. J Biol Chem. 2003;278:45160–45170. [Abstract] [Google Scholar]
- Holroyd C, Kistner U, Annaert W, Jahn R. Fusion of endosomes involved in synaptic vesicle recycling. Mol Biol Cell. 1999;10:3035–3044. [Europe PMC free article] [Abstract] [Google Scholar]
- Holroyd P, Lang T, Wenzel D, De Camilli P, Jahn R. Imaging direct, dynamin-dependent recapture of fusing secretory granules on plasma membrane lawns from PC12 cells. Proc Natl Acad Sci U S A. 2002;99:16806–16811. [Europe PMC free article] [Abstract] [Google Scholar]
- Jaiswal JK, Andrews NW, Simon SM. Membrane proximal lysosomes are the major vesicles responsible for calcium-dependent exocytosis in nonsecretory cells. J Cell Biol. 2002;159:625–635. [Europe PMC free article] [Abstract] [Google Scholar]
- Jaiswal JK, Chakrabarti S, Andrews NW, Simon SM. Synaptotagmin VII restricts fusion pore expansion during lysosomal exocytosis. Plos Biology. 2004;2:1224–1232. [Europe PMC free article] [Abstract] [Google Scholar]
- Jaiswal JK, Fix M, Takano T, Nedergaard M, Simon SM. Resolving vesicle fusion from lysis to monitor calcium-triggered lysosomal exocytosis in astrocytes. Proc Natl Acad Sci U S A. 2007;104:14151–14156. [Europe PMC free article] [Abstract] [Google Scholar]
- Jaiswal JK, Simon SM. Imaging single events at the cell membrane. Nat Chem Biol. 2007;3:92–98. [Abstract] [Google Scholar]
- Katz B. Quantal mechanism of neural transmitter release. Science. 1971;173:123–126. [Abstract] [Google Scholar]
- Kreitzer G, Marmorstein A, Okamoto P, Vallee R, Rodriguez-Boulan E. Kinesin and dynamin are required for post-Golgi transport of a plasma-membrane protein. Nat Cell Biol. 2000;2:125–127. [Abstract] [Google Scholar]
- Kreitzer G, Schmoranzer J, Low SH, Li X, Gan Y, Weimbs T, Simon SM, Rodriguez-Boulan E. Three-dimensional analysis of post-Golgi carrier exocytosis in epithelial cells. Nat Cell Biol. 2003;5:126–136. [Abstract] [Google Scholar]
- Lew DJ, Simon SM. Characterization of constitutive exocytosis in the yeast Saccharomyces cerevisiae. J Membr Biol. 1991;123:261–268. [Abstract] [Google Scholar]
- Macia E, Ehrlich M, Massol R, Boucrot E, Brunner C, Kirchhausen T. Dynasore, a cell-permeable inhibitor of dynamin. Dev Cell. 2006;10:839–850. [Abstract] [Google Scholar]
- Mayorga LS, Beron W, Sarrouf MN, Colombo MI, Creutz C, Stahl PD. Calcium-dependent fusion among endosomes. J Biol Chem. 1994;269:30927–30934. [Abstract] [Google Scholar]
- Merrifield CJ, Feldman ME, Wan L, Almers W. Imaging actin and dynamin recruitment during invagination of single clathrin-coated pits. Nat Cell Biol. 2002;4:691–698. [Abstract] [Google Scholar]
- Merrifield CJ, Perrais D, Zenisek D. Coupling between clathrin-coated-pit invagination, cortactin recruitment, and membrane scission observed in live cells. Cell. 2005;121:593–606. [Abstract] [Google Scholar]
- Miller SG, Moore HP. Reconstitution of constitutive secretion using semi-intact cells: regulation by GTP but not calcium. J Cell Biol. 1991;112:39–54. [Europe PMC free article] [Abstract] [Google Scholar]
- Newton AJ, Kirchhausen T, Murthy VN. Inhibition of dynamin completely blocks compensatory synaptic vesicle endocytosis. Proc Natl Acad Sci U S A. 2006;103:17955–17960. [Europe PMC free article] [Abstract] [Google Scholar]
- Palade G. Intracellular Aspects of the Process of Protein Synthesis. Science. 1975;189:867. [Abstract] [Google Scholar]
- Patterson GH, Hirschberg K, Polishchuk RS, Gerlich D, Phair RD, Lippincott-Schwartz J. Transport through the Golgi apparatus by rapid partitioning within a two-phase membrane system. Cell. 2008;133:1055–1067. [Europe PMC free article] [Abstract] [Google Scholar]
- Peters C, Baars TL, Buhler S, Mayer A. Mutual control of membrane fission and fusion proteins. Cell. 2004;119:667–678. [Abstract] [Google Scholar]
- Peters C, Mayer A. Ca2+/calmodulin signals the completion of docking and triggers a late step of vacuole fusion. Nature. 1998;396:575–580. [Abstract] [Google Scholar]
- Porat A, Elazar Z. Regulation of intra-Golgi membrane transport by calcium. J Biol Chem. 2000;275:29233–29237. [Abstract] [Google Scholar]
- Puertollano R, van der Wel NN, Greene LE, Eisenberg E, Peters PJ, Bonifacino JS. Morphology and dynamics of clathrin/GGA1-coated carriers budding from the trans-Golgi network. Mol Biol Cell. 2003;14:1545–1557. [Europe PMC free article] [Abstract] [Google Scholar]
- Rappoport JZ, Simon SM. Real-time analysis of clathrin-mediated endocytosis during cell migration. J Cell Sci. 2003;116:847–855. [Abstract] [Google Scholar]
- Rivera VM, Wang X, Wardwell S, Courage NL, Volchuk A, Keenan T, Holt DA, Gilman M, Orci L, Cerasoli F, Jr, Rothman JE, Clackson T. Regulation of protein secretion through controlled aggregation in the endoplasmic reticulum. Science. 2000;287:826–830. [Abstract] [Google Scholar]
- Rollins CT, Rivera VM, Woolfson DN, Keenan T, Hatada M, Adams SE, Andrade LJ, Yaeger D, van Schravendijk MR, Holt DA, Gilman M, Clackson T. A ligand-reversible dimerization system for controlling protein-protein interactions. Proc Natl Acad Sci U S A. 2000;97:7096–7101. [Europe PMC free article] [Abstract] [Google Scholar]
- Ryan TA, Smith SJ, Reuter H. The timing of synaptic vesicle endocytosis. Proc Natl Acad Sci U S A. 1996;93:5567–5571. [Europe PMC free article] [Abstract] [Google Scholar]
- Schmoranzer J, Goulian M, Axelrod D, Simon SM. Imaging constitutive exocytosis with total internal reflection fluorescence microscopy. J Cell Biol. 2000;149:23–32. [Europe PMC free article] [Abstract] [Google Scholar]
- Schmoranzer J, Simon SM. Role of microtubules in fusion of post-Golgi vesicles to the plasma membrane. Mol Biol Cell. 2003;14:1558–1569. [Europe PMC free article] [Abstract] [Google Scholar]
- Sokac AM, Bement WM. Kiss-and-coat and compartment mixing: coupling exocytosis to signal generation and local actin assembly. Mol Biol Cell. 2006;17:1495–1502. [Europe PMC free article] [Abstract] [Google Scholar]
- Stevens CF, Williams JH. “Kiss and run” exocytosis at hippocampal synapses. Proc Natl Acad Sci U S A. 2000;97:12828–12833. [Europe PMC free article] [Abstract] [Google Scholar]
- Tsuboi T, McMahon HT, Rutter GA. Mechanisms of dense core vesicle recapture following “kiss and run” (“cavicapture”) exocytosis in insulin-secreting cells. J Biol Chem. 2004;279:47115–47124. [Abstract] [Google Scholar]
- Wang CT, Bai J, Chang PY, Chapman ER, Jackson MB. Synaptotagmin-Ca2+ triggers two sequential steps in regulated exocytosis in rat PC12 cells: fusion pore opening and fusion pore dilation. J Physiol. 2006;570:295–307. [Abstract] [Google Scholar]
- Wang CT, Grishanin R, Earles CA, Chang PY, Martin TF, Chapman ER, Jackson MB. Synaptotagmin modulation of fusion pore kinetics in regulated exocytosis of dense-core vesicles. Science. 2001;294:1111–1115. [Abstract] [Google Scholar]
- Williams DA, Fogarty KE, Tsien RY, Fay FS. Calcium gradients in single smooth muscle cells revealed by the digital imaging microscope using Fura-2. Nature. 1985;318:558–561. [Abstract] [Google Scholar]
- Zhang Z, Jackson MB. Temperature Dependence of Fusion Kinetics and Fusion Pores in Ca2+-triggered Exocytosis from PC12 Cells. J Gen Physiol. 2008;131:117–124. [Europe PMC free article] [Abstract] [Google Scholar]
Full text links
Read article at publisher's site: https://doi.org/10.1016/j.cell.2009.04.064
Read article for free, from open access legal sources, via Unpaywall:
http://www.cell.com/article/S0092867409005819/pdf
Subscription required at www.cell.com
http://www.cell.com/cgi/content/reprint/137/7/1308
Citations & impact
Impact metrics
Citations of article over time
Alternative metrics
Smart citations by scite.ai
Explore citation contexts and check if this article has been
supported or disputed.
https://scite.ai/reports/10.1016/j.cell.2009.04.064
Article citations
Single molecule dynamics in a virtual cell combining a 3-dimensional matrix model with random walks.
Sci Rep, 14(1):20032, 28 Aug 2024
Cited by: 0 articles | PMID: 39198682 | PMCID: PMC11358523
Clathrin-associated carriers enable recycling through a kiss-and-run mechanism.
Nat Cell Biol, 26(10):1652-1668, 19 Sep 2024
Cited by: 0 articles | PMID: 39300312
Molecular identification of bulbospinal ON neurons by GPER, which drives pain and morphine tolerance.
J Clin Invest, 133(1):e154588, 03 Jan 2023
Cited by: 11 articles | PMID: 36346677 | PMCID: PMC9797334
Combined nanometric and phylogenetic analysis of unique endocytic compartments in Giardia lamblia sheds light on the evolution of endocytosis in Metamonada.
BMC Biol, 20(1):206, 21 Sep 2022
Cited by: 5 articles | PMID: 36127707 | PMCID: PMC9490929
Caveolin-initiated macropinocytosis is required for efficient silica nanoparticles' transcytosis across the alveolar epithelial barrier.
Sci Rep, 12(1):9474, 08 Jun 2022
Cited by: 8 articles | PMID: 35676405 | PMCID: PMC9178038
Go to all (82) article citations
Data
Data behind the article
This data has been text mined from the article, or deposited into data resources.
BioStudies: supplemental material and supporting data
Similar Articles
To arrive at the top five similar articles we use a word-weighted algorithm to compare words from the Title and Abstract of each citation.
Role of microtubules in fusion of post-Golgi vesicles to the plasma membrane.
Mol Biol Cell, 14(4):1558-1569, 01 Apr 2003
Cited by: 36 articles | PMID: 12686609 | PMCID: PMC153122
Imaging the recruitment and loss of proteins and lipids at single sites of calcium-triggered exocytosis.
Mol Biol Cell, 27(15):2423-2434, 15 Jun 2016
Cited by: 31 articles | PMID: 27307587 | PMCID: PMC4966983
Actin controls the vesicular fraction of dopamine released during extended kiss and run exocytosis.
ACS Chem Biol, 9(3):812-820, 21 Jan 2014
Cited by: 34 articles | PMID: 24400601 | PMCID: PMC3985473
TRP Channel Trafficking
CRC Press/Taylor & Francis, Boca Raton (FL), 05 Jan 2011
Cited by: 0 articles | PMID: 21204515
ReviewBooks & documents Free full text in Europe PMC
Funding
Funders who supported this work.
NIAMS NIH HHS (4)
Grant ID: R01 AR055686-02
Grant ID: R01 AR055686
Grant ID: R01AR055686
Grant ID: R01 AR055686-01A2
NIGMS NIH HHS (4)
Grant ID: P20 GM072015-04
Grant ID: P20 GM072015-03
Grant ID: P20 GM072015
Grant ID: R01 GM087977