Abstract
Free full text

Increased Hepatic Myeloperoxidase Activity in Obese Subjects with Nonalcoholic Steatohepatitis
Abstract
Inflammation and oxidative stress are considered critical factors in the progression of nonalcoholic fatty liver disease. Myeloperoxidase (MPO) is an important neutrophil enzyme that can generate aggressive oxidants; therefore, we studied the association between MPO and nonalcoholic fatty liver disease. The distribution of inflammatory cells containing MPO in liver biopsies of 40 severely obese subjects with either nonalcoholic steatohepatitis (NASH) (n = 22) or simple steatosis (n = 18) was investigated by immunohistochemistry. MPO-derived oxidative protein modifications were identified by immunohistochemistry and correlated to hepatic gene expression of CXC chemokines and M1/M2 macrophage markers as determined by quantitative PCR. MPO plasma levels were determined by ELISA. The number of hepatic neutrophils and MPO-positive Kupffer cells was increased in NASH and was accompanied by accumulation of hypochlorite-modified and nitrated proteins, which can be generated by the MPO-H2O2 system. Liver CXC chemokine expression was higher in patients with accumulation of MPO-mediated oxidation products and correlated with hepatic neutrophil sequestration. Plasma MPO levels were elevated in NASH patients. Interestingly, neutrophils frequently surrounded steatotic hepatocytes, resembling the crown-like structures found in obese adipose tissue. Furthermore, hepatic M2 macrophage marker gene expression was increased in NASH. Our data indicate that accumulation of MPO-mediated oxidation products, partly derived from Kupffer cell MPO, is associated with induction of CXC chemokines and hepatic neutrophil infiltration and may contribute to the development of NASH.
The obesity-associated infiltration of neutrophils and macrophages into liver and adipose tissue is increasingly appreciated to be central to the development of chronic diseases such as nonalcoholic fatty liver disease (NAFLD) and insulin resistance.1 In both liver and adipose tissue, increased levels of free fatty acids are thought to initiate the activation of inflammatory pathways that are responsible for attraction and activation of immune cells.2,3 Furthermore, recruitment of neutrophils to the liver can be mediated by secretion of the CXC chemokines interleukin (IL)–8 and growth-related oncogene (GRO)-α.4,5 The inflammatory processes in the liver have been shown to play a particularly important role in the progression of the benign steatotic stage of NAFLD to the more advanced stages of nonalcoholic steatohepatitis (NASH), fibrosis, and cirrhosis.6,7
Next to inflammation, oxidative stress has been put forward as an important contributor to the progression of NAFLD.8,9 For example, oxidative stress leading to lipid peroxidation induces the progression of steatosis toward necroinflammation or fibrosis in both animal models of steatohepatitis and humans with steatotic liver disorders.10,11,12,13 Oxidative stress in the fatty liver can result from cytochrome P450 activity, peroxisomal β-oxidation, mitochondrial electron leak, as well as activities of recruited inflammatory cells.14
One of the principal molecules released after recruitment and activation of phagocytes is myeloperoxidase (MPO), an important enzyme involved in the generation of reactive oxygen species.15 MPO is highly expressed by neutrophils and as such widely used as a neutrophil marker. In the presence of physiological chloride concentrations, MPO reacts with hydrogen peroxide (H2O2, formed by the respiratory burst) to catalyze formation of hypochlorous acid/hypochlorite (HOCl/OCl−) and other oxidizing species.15 These oxidants may contribute to host tissue damage at sites of inflammation through reactions with a wide range of biological substrates, including DNA, lipids, and protein amino groups.16 In the absence of physiological chloride concentrations, the MPO-H2O2 system can also form reactive nitrogen species17 that may initiate lipid peroxidation or form protein tyrosine residues, another posttranslational modification found in many pathological conditions.18
Besides its abundant presence in neutrophils, where MPO makes up ~5% of the total cell protein content, lower levels of MPO have been found in monocytes, certain macrophages,19,20,21 and in a subpopulation of Kupffer cells.22 The presence of MPO in these cells may reflect their inflammatory status, which was recently shown to be modulated in obesity. For example, anti-inflammatory M2 macrophages with tissue-remodeling capacities were largely absent in obese adipose tissue in mice, whereas accumulation of proinflammatory M1 macrophages was associated with obesity and insulin resistance.23 Moreover, attenuation of the M2 differentiation program of Kupffer cells was associated with hepatic steatosis.24 Interestingly, M1 macrophages are known to generate high amounts of reactive oxygen and nitrogen species.25 Therefore, MPO-containing M1 macrophages/Kupffer cells could play a role in NAFLD.
To study the potential contribution of increased MPO activity to the progression of human NAFLD, we quantified inflammatory cells that express MPO and assessed hepatic accumulation of HOCl-modified proteins and nitrated proteins. Furthermore, we determined hepatic gene expression of IL-8 and GRO-α in relation to MPO-derived tissue damage and hepatic neutrophil sequestration to investigate a potential link between MPO activity and neutrophil accumulation. In addition, we analyzed for the first time hepatic gene expression of M1 and M2 macrophage phenotype markers in human NAFLD. Our results support an important role for Kupffer cell MPO-induced oxidative stress in the progression of NAFLD.
Materials and Methods
Liver Specimens
Human liver specimens were obtained during bariatric surgery from 40 severely obese patients (body mass index > 40). None of the patients had suffered from viral hepatitis or autoimmune-related disorders or reported excessive alcohol consumption (>20 g/day). Each liver specimen was divided into three pieces. One part was used for RNA isolation, another part was fixed in formalin, and a third part was embedded in Tissue-Tek optimal cutting temperature compound (Sakura Finetek Europe, Zoeterwoude, The Netherlands) and snap-frozen in 2-methylbutane. Biopsies were stained with H&E, periodic acid-Schiff with diastase, and Masson’s trichrome and contained a sufficient number of portal tracts to allow correct evaluation of histological features by an experienced pathologist who was blinded to the clinical context of the biopsy and laboratory parameters. Subjects were divided into two groups. The steatosis group comprised patients showing >5% steatotic hepatocytes without significant inflammation as observed after H&E staining (no intra-acinar inflammatory foci per ×20 with a ×20 ocular and no portal tract inflammation). The NASH group showed >5% steatotic hepatocytes together with lobular inflammation and hepatocellular ballooning. Grading and staging of NASH severity were performed according to the criteria of Brunt.26 Important patient characteristics are summarized in Table 1. This study was approved by the Medical Ethical Board of the Maastricht University Medical Centre in line with the ethical guidelines of the 1975 Declaration of Helsinki, and informed consent in writing was obtained from each subject.
Table 1
Clinical Characteristics of Patients Studied
Steatosis | NASH | |
---|---|---|
No. of patients | 18 | 22 |
Sex (female/male) | 13/5 | 16/6 |
Age (years) | 42.8 ± 2.3 | 43.6 ± 2.0 (P = 0.55) |
BMI (kg/m2) | 46.7 ± 1.4 | 50.0 ± 2.2 (P = 0.68) |
Fasting glucose (mmol/L) | 6.1 ± 0.3 | 6.2 ± 0.4 (P = 0.95) |
ALT (IU) | 22.5 ± 3.0 | 50.3 ± 11.0 (P < 0.01) |
AST (IU) | 18.3 ± 2.2 | 40.4 ± 6.4 (P < 0.01) |
NASH, nonalcoholic steatohepatitis; BMI, body mass index; ALT, alanine aminotransferase; AST, aspartate aminotransferase.
Immunohistochemistry
Formalin-fixed, paraffin-embedded liver biopsies were cut into 5-μm-thick tissue sections, deparaffinized, and rehydrated. Endogenous peroxidase activity was blocked by incubation in 0.6% H2O2 for 15 minutes. Primary antibodies were applied for 1 hour after blocking with 10% serum. The following murine monoclonal antibodies were used as primary antibodies: (i) anti-CD68 (clone Kp1, dilution 1/400; Dako, Glostrup, Denmark) specific for monocytes/macrophages; (ii) anti-human neutrophil peptide 1-3 (HNP1-3, clone D21, dilution 1:500; Hycult Biotechnology, Uden, The Netherlands) specific for neutrophils; (iii) anti-α-smooth muscle actin (clone 1A4, dilution 1:200; Sigma-Aldrich, Zwijndrecht, The Netherlands) specific for activated stellate cells; (iv) anti-HOCl-modified proteins (clone 2D10G9,27 dilution 1:10); and (v) anti-nitrotyrosine (clone HM11,28 dilution 1:50; Hycult Biotechnology). Polyclonal rabbit anti-human MPO antiserum (dilution 1:1000; Dako) was used as primary antibody to identify MPO. Horseradish peroxidase-labeled goat-anti-rabbit IgG (1:500; Jackson ImmunoResearch, Suffolk, UK) was applied as secondary antibody in the MPO staining and horseradish peroxidase-labeled goat-anti-mouse IgG (1:1000; Invitrogen, Carlsbad, CA) was applied during the HNP1-3, α-smooth muscle actin, and nitrotyrosine staining procedure. For CD68 and HOCl-modified protein stainings, endogenous biotin was blocked before primary antibody incubation with a biotin blocking system (Dako), biotinylated rabbit-anti-mouse IgG was applied as secondary antibody for 30 minutes at a 1:300 dilution, and the avidin-biotin-horseradish peroxidase system (Dako) was used. 3-Amino-9-ethylcarbazole or diaminobenzidine tetrahydrochloride was used as chromogen. Tissue sections were counterstained with Mayer’s hematoxylin. For the CD68/MPO double staining, the MPO staining protocol was performed using diaminobenzidine tetrahydrochloride as chromogen, followed by the CD68 staining protocol using HistoGreen (Linaris, Wertheim Bettingen, Germany) as chromogen.
For immunofluorescent double staining of MPO/CD68 and MPO/HNP1-3, 4-μm-thick frozen sections were fixed at −20°C with acetone, dried, and rehydrated. Endogenous peroxidase activity was blocked with 0.3% H2O2 in methanol, and nonspecific binding sites were blocked with goat serum in PBS. Sections were incubated with rabbit-anti-MPO/mouse-anti-CD68 or rabbit-anti-MPO/mouse-anti-HNP1-3, rinsed with PBS, and subsequently incubated with Alexa Fluor 594-conjugated goat anti-mouse IgG2a (4 μg/ml; Invitrogen Molecular Probes, Eugene, OR) and Tx red-conjugated goat anti-rabbit IgG (1:50; Jackson ImmunoResearch). Nuclei were stained with 4′,6-diamino-2-phenylindol, and sections were mounted with Fluorescent Mounting Medium (Dako).
Quantification of Immunohistochemical Stainings
For the HNP1-3, MPO, and CD68 stainings, four to six randomly selected different parts of stained sections were photographed using a Zeiss Axioscope (Zeiss, Göttingen, Germany) and a standard charge-coupled device camera (Stemmer Imaging, Puchheim, Germany) at ×200 magnification. Pictures were analyzed with Leica QWin image analysis software (Leica Microsystems, Cambridge, UK). Positive cell number was recorded as well as the extent of steatosis as defined by liver parenchyma area showing no hematoxylin staining (excluding vascular lumen). Furthermore, mean steatotic hepatocyte size was calculated by dividing the steatotic area by the number of hepatocytes showing steatosis. For the staining of HOCl-modified and nitrotyrosine epitopes, whole sections were observed and classified according to presence or absence of staining.
MPO ELISA
Blood samples were collected the day before surgery after at least 8 hours of fasting using evacuated tubes containing EDTA and processed as described previously.29 Plasma MPO concentrations were measured using a sandwich ELISA for human MPO according to the manufacturer’s protocol (Hycult Biotechnology). All plasma samples were analyzed in duplicate in the same run. The intra-assay coefficient of variance was <10%.
Quantitative Real-Time PCR
Total RNA was isolated from 50 mg liver tissue by homogenization in Tri-reagent (Sigma-Aldrich), according to the manufacturer’s instructions. RNA (750 ng) was converted to cDNA using the iScript cDNA synthesis kit (Bio-Rad, Hercules, CA). Quantitative real-time PCR was performed with the ABsolute SYBR Green Master Mix (ABgene, Leusden, The Netherlands) and the iQ5 iCycler (Bio-Rad). Relative expression was assessed in duplicate by the delta cycle threshold method after normalization for β-actin and β2-microglobulin expression. Sequences of the applied PCR primers are listed in Table 2.
Table 2
Primers Used for Quantitative Real-Time Reverse Transcription-PCR
Name | Forward | Reverse |
---|---|---|
GRO-α | 5′-CCACTGCGCCCAAACC-3′ | 5′-GCAGGATTGAGGCAAGCTTT-3′ |
IL-8 | 5′-CTGGCCGTGGCTCTCTTG-3′ | 5′-TTAGCACTCCTTGGCAAAACTG-3′ |
IL-1β | 5′-CTGAGCTCGCCAGTGAAATG-3′ | 5′-TTTAGGGCCATCAGCTTCAAA-3′ |
IL-6 | 5′-TCCAGGAGCCCAGCTATGAA-3′ | 5′-GAGCAGCCCCAGGGAGAA-3′ |
IL-10 | 5′-GGCGCTGTCATCGATTTCTT-3′ | 5′-TGGAGCTTATTAAAGGCATTCTTCA-3′ |
Dectin-1 | 5′-TCAGAACCACAGCTACCCAAGA-3′ | 5′-GCACACTACACAGTTGGTCATAAATG-3′ |
EMR-1 | 5′-GCATGACACTGGCATCTTTTTG-3′ | 5′-AATGTCTAAGTATTCCGTCCGAACA-3′ |
β-actin | 5′-GCTGTGCTACGTCGCCCTG-3′ | 5′-GGAGGAGCTGGAAGCAGCC-3′ |
β2-microglobulin | 5′-TCCATCCGACATTGAAGTTG-3′ | 5′-CGGCAGGCATACTCATCTT-3′ |
EMR-1, epidermal growth factor-like module containing mucin-like hormone receptor 1.
Statistical Analysis
Results are presented as mean ± SEM. Statistical analyses were conducted using SPSS software (version 15.0). The Mann-Whitney rank sum test, the Kruskal-Wallis one-way analysis of variance, and the χ2 analysis were applied to study differences among the study groups, and associations between parameters were determined through Spearman correlation analysis. Values of P <0.05 were considered statistically significant.
Results
Increased MPO-Positive Cell Number in NASH Is Associated with Extent of Steatosis
Forty-five percent (n = 18) of the 40 patients that were included in our study showed simple hepatic steatosis without significant inflammation, whereas 55% (n = 22) were diagnosed with NASH. Characteristics of the study groups with respect to the degree of steatosis, inflammation, and fibrosis are shown in Table 3. In comparison with the simple steatosis group, patients with NASH showed a significantly higher degree of steatosis as determined semiquantitatively by the pathologist (P < 0.001). Quantitative analyses also revealed that there was significantly more steatosis in patients with NASH as compared with patients with simple steatosis (P < 0.001) (Figure 1A). In addition, the mean size of steatotic hepatocytes was significantly higher in the NASH group (P < 0.001) (Figure 1A).
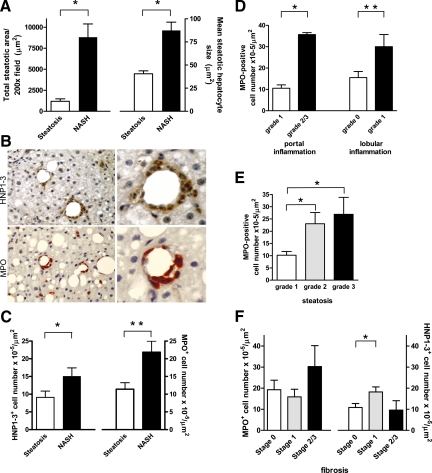
Increased MPO-positive cell number in NASH is associated with steatosis. A: The total amount of steatosis as well as the mean size of steatotic hepatocytes is significantly higher in subjects with NASH as compared with subjects with simple steatosis (*P < 0.001). B: Frequent organization of HNP1-3-positive neutrophils (red/brown, upper panel) and MPO-positive neutrophils (red/brown, lower panel) into crown-like structures surrounding steatotic hepatocytes (magnification, left panels, ×200, right panels, ×540). C: Subjects with NASH display more HNP1-3-positive neutrophils and more MPO-positive cells in the liver as compared with subjects with simple steatosis (*P = 0.02 and **P = 0.03, respectively). D: Higher grades of portal and lobular inflammation are associated with increased numbers of MPO-positive cells (*P < 0.01 and **P < 0.05, respectively). E: Significantly higher number of MPO-positive cells in subjects with grade 2 and grade 3 steatosis (*P < 0.05). F: The number of MPO-positive cells is not related to fibrosis stage in NASH as analyzed by one-way analysis of variance (*P = 0.75), whereas the number of HNP1-3-positive cells is higher in subjects with stage 1 fibrosis (*P = 0.05).
Table 3
Semiquantitative Analysis of the Degree of Steatosis, Inflammation, and Fibrosis in the Study Groups according to the Brunt Classification
Steatosis | NASH | |
---|---|---|
Steatosis | ||
![]() | 16 | 5 |
![]() | 1 | 8 |
![]() | 1 | 9 |
Inflammation | ||
![]() | ||
![]() ![]() | 0 | 14 |
![]() ![]() | 0 | 4 |
![]() ![]() | 0 | 4 |
![]() | ||
![]() ![]() | 0 | 11 |
![]() ![]() | 0 | 11 |
Fibrosis | ||
![]() | 0 | 7 |
![]() | 0 | 8 |
![]() | 0 | 4 |
![]() | 0 | 3 |
χ2 analysis for steatosis: P < 0.001.
To further characterize the cells contributing to inflammation, we first identified neutrophils by immunohistochemical detection of the specific marker HNP1-3 (also known as α-defensin30) and by detection of MPO. Interestingly, HNP1-3-positive and MPO-positive neutrophils were frequently associated with lipid-laden hepatocytes (Figure 1B), forming structures that strikingly resemble the so-called “crown-like structures” commonly found around necrotic adipocytes in obese adipose tissue.
The degree of neutrophil infiltration into the liver varied considerably among the patients. As expected, the number of HNP1-3-positive neutrophils as well as the number of MPO-positive cells were significantly higher in the NASH group compared with the steatosis group (Figure 1C). In addition, the number of MPO-positive cells was higher in patients with grade 2 or 3 portal inflammation as compared with patients showing grade 1 (P < 0.01; Figure 1D). Higher degrees of lobular inflammation were also associated with more MPO-positive cells (P < 0.05; Figure 1D). Furthermore, the number of MPO-positive cells correlated with both the extent of steatosis (rs = 0.57, P < 0.01) and mean steatotic hepatocyte size (rs = 0.52, P < 0.01). In line with this observation, the number of MPO-positive cells was significantly higher in patients with a higher grade of steatosis as scored by the pathologist (P = 0.03) (Figure 1E). On the other hand, the number of MPO-positive cells was unrelated to the degree of fibrosis (P = 0.75) (Figure 1F). Similarly, whereas the number of HNP1-3-positive cells was somewhat increased in the group of patients with stage 1 fibrosis (P = 0.05), patients with stage 2 or 3 fibrosis displayed a comparable number of HNP1-3-positive cells as patients without fibrosis (stage 0) (Figure 1F). Activation of hepatic stellate cells as reflected by immunohistochemical detection of α-smooth muscle actin was not associated with steatotic hepatocytes or NAFLD severity (data not shown).
Increased Prevalence of MPO-Positive Kupffer Cells in NASH
Interestingly, there were more MPO-positive cells than HNP1-3-positive cells, particularly in the NASH livers (Figure 1C). However, all HNP1-3-positive cells showed positivity for MPO (Figure 2A). This suggested that MPO-expressing cells other than neutrophils, most likely resident or infiltrating macrophages, were increased in NASH. Indeed, close examination of MPO-positive cells revealed that many of them lacked the multilobular nucleus characteristic of neutrophils. To further investigate the nature of these cells, double stainings using anti-MPO and anti-CD68 antibodies were performed. MPO/CD68-double-positive cells with a Kupffer cell-like morphology were frequently detected in patients with NASH (Figure 2B). Because the number of CD68-positive cells did not differ between the NASH group and patients with simple steatosis (P = 0.28; Figure 2C), this indicates that there is a selective increase in the population of MPO-expressing Kupffer cells in patients with NASH. Of note, many of these MPO-positive Kupffer cells were observed close to crown-like structures around steatotic hepatocytes (Figure 2B).
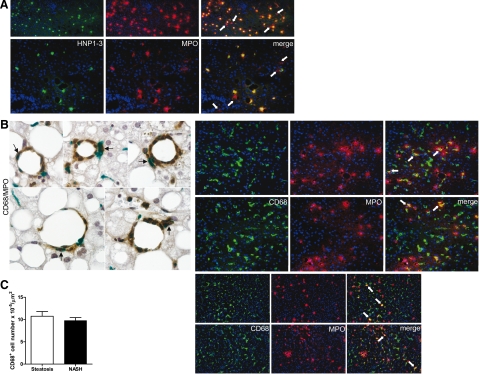
Increased prevalence of MPO-positive Kupffer cells in NASH. A: Representative immunofluorescent double stainings of HNP1-3 (green) and MPO (red) in the liver of different subjects with NASH. All neutrophils showing HNP1-3 staining are also MPO positive. Occasionally, MPO-containing cells negative for HNP1-3 can be observed (see white arrows; magnification, upper panel, ×100; lower panel, ×200). B: Left panel, Double staining for CD68 (green) and MPO (brown) in liver sections of five different subjects with NASH, showing colocalization of CD68 and MPO (see black arrows). The elongated morphology of double-labeled cells indicates that they represent Kupffer cells (magnification, ×600). Right panels, Immunofluorescent double stainings of CD68 (green) and MPO (red) indicate that subjects with NASH frequently exhibit Kupffer cells containing MPO (see white arrows; magnification, upper panel, ×200; lower panel, ×100). C: Comparable CD68-positive cell number in the liver of severely obese subjects with NASH and subjects with simple steatosis (P = 0.28).
Hepatic Accumulation of HOCl-Modified and Nitrated Proteins in NASH
We next investigated whether the increased staining for immunoreactive MPO in the liver of NASH patients translated into accumulation of MPO-derived oxidative modifications. HOCl-modified epitopes were detected in 77% (n = 17) of the subjects in the NASH group. In contrast, only 22% (n = 4) of patients with simple steatosis showed staining for HOCl-modified epitopes. The extent and intensity of the HOCl-staining varied between individuals (Figure 3A). In some cases, more pronounced staining was associated with steatotic hepatocytes. Omission of the primary antibody 2D10G9 or preabsorption with HOCl-modified (lipo)protein prevented antibody binding (data not shown), confirming that the staining was specific for HOCl-modified epitopes generated by the MPO-H2O2-chloride system. Importantly, the number of MPO-positive cells was significantly higher in patients showing staining for HOCl-modified proteins (P = 0.02), whereas the number of HNP1-3-positive cells was not significantly increased (P = 0.14; Figure 3B). This observation is consistent with a substantial contribution of Kupffer cells to MPO-mediated oxidative damage.
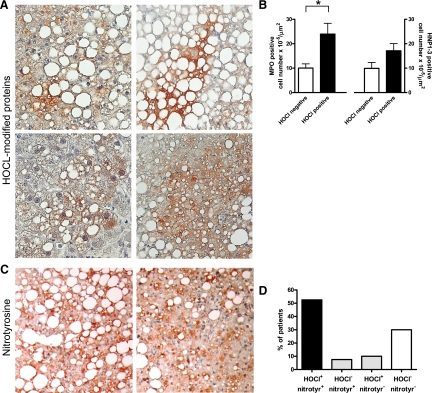
Accumulation of MPO-mediated HOCl-modified and nitrated proteins in NASH. A: Representative staining patterns for HOCl-modified proteins (red/brown) in livers of four different patients with NASH (magnification, ×200). More intense staining for HOCl-modified epitopes is frequently associated with steatotic hepatocytes. B: The number of hepatic MPO-positive cells is significantly higher in patients that show HOCl-modified protein staining (*P = 0.02). In contrast, HNP1-3-positive cell number is not significantly increased in this group (P = 0.14). C: Representative immunostainings of nitrotyrosine residues (red/brown) in livers of two patients with NASH (magnification, ×200). More intense staining is predominantly associated with steatotic hepatocytes. D: In most patients, HOCl-modified protein accumulation is coupled to nitrotyrosine accumulation.
Like HOCl-modified proteins, nitrated proteins were more frequently detected in the livers of patients with NASH. Seventy-two percent (n = 16) of the patients with NASH displayed staining for nitrotyrosine, as opposed to 33% (n = 6) of patients with simple steatosis. Again, the extent and intensity of staining for nitrotyrosine varied (Figure 3C). In a similar manner, as shown for HOCl-modified proteins, immunoreactive nitrotyrosine epitopes were sometimes detected around steatotic hepatocytes. As expected, the vast majority of patients showing staining for HOCl-modified proteins also showed pronounced staining for nitrated protein adducts (Figure 3D).
Increased Plasma MPO Levels in NASH Patients
Previously, we found elevated plasma MPO levels in severely obese patients compared with normal weight controls.31 On the basis of the remarkable increase of both MPO-positive cells and MPO-derived staining for HOCI-modified and nitrated epitopes in the liver of NASH patients, we hypothesized that plasma MPO levels could be higher in the subgroup of severely obese patients with NASH. Indeed, plasma MPO levels were significantly elevated in the NASH group compared with the patients with simple steatosis (59.5 ± 6.5 versus 41.1 ± 4.8 ng/ml, P = 0.03; Figure 4). However, there was no significant correlation between plasma MPO levels and the number of MPO-positive cells in the liver.
Expression of CXC Chemokines Correlates with Neutrophil Accumulation in NAFLD
Recruitment of neutrophils to inflammatory sites is potently induced by secretion of the CXC chemokines IL-8 and GRO-α, a process that occurs in response to cellular injury such as accumulation of chlorinated or nitrated macromolecules.4,5 Therefore, we investigated the expression of these chemokines in the liver of severely obese patients and studied their relation with MPO-derived oxidative modifications and neutrophil sequestration. Expression of both CXC chemokines was higher in patients that displayed simultaneous accumulation of HOCl-modified and nitrotyrosine epitopes when compared with patients without such accumulation (P = 0.05 and P = 0.04, respectively; Figure 5A). Moreover, expression of both GRO-α and IL-8 correlated with the number of HNP1-3-positive neutrophils (GRO-α, rs = 0.42, P < 0.01; IL-8, rs = 0.44, P < 0.01; Figure 5B). In line with our observation that many MPO-positive cells in the liver of NASH patients are not necessarily neutrophils, expression of these CXC chemokines did not correlate with the number of MPO-positive cells. As expected, expression of IL-8 and GRO-α was higher in the NASH group, although the difference with the simple steatosis group was not statistically significant (P = 0.76 and P = 0.64, respectively; Figure 5C).
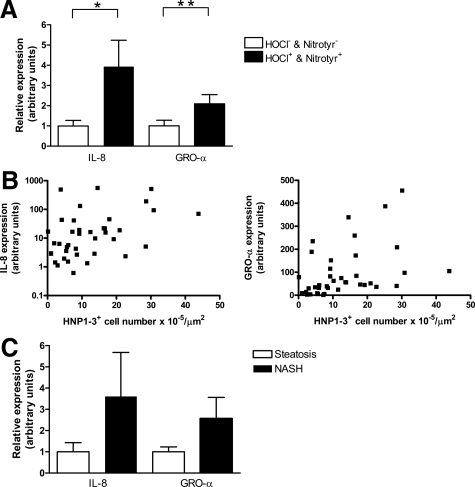
Hepatic expression of CXC chemokines is associated with MPO-mediated oxidative damage and neutrophil sequestration in NAFLD. A: Hepatic expression of IL-8 and GRO-α is higher in patients that show accumulation of HOCl-modified proteins and nitrotyrosine as compared with patients without accumulation of MPO-mediated oxidative products (*P = 0.04 and **P = 0.05, respectively). B: Significant correlation between hepatic IL-8 mRNA expression and hepatic neutrophil infiltration (rs = 0.44, P < 0.01) and GRO-α mRNA expression and hepatic neutrophil infiltration (rs = 0.42, P < 0.01). C: Gene expression of both IL-8 (P = 0.76) and GRO-α (P = 0.64) is elevated but not significantly increased in the liver of subjects with NASH in comparison with severely obese subjects with only steatosis.
M2 Macrophage Phenotype Markers Show Higher Hepatic Expression in NASH
The increased immunohistochemical staining for MPO in Kupffer cells in patients with NASH suggested that these cells might have a proinflammatory M1-like phenotype. To investigate this hypothesis, we performed mRNA expression analysis of several macrophage phenotype markers. Expression of IL-6, which is associated with the M1 phenotype, was markedly elevated in the NASH group compared with the simple steatosis group (Figure 6A), whereas expression of IL-1β, another M1 marker, was not altered (Figure 6A). This finding may suggest that the proinflammatory M1 phenotype is not dominant in NASH. In line with this, the expression of dectin-1, epidermal growth factor-like module containing mucin-like hormone receptor 1, and IL-10, all markers of anti-inflammatory M2 macrophages,32 was significantly higher in the liver of NASH patients (Figure 6B).
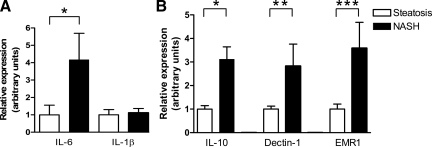
Increased hepatic expression of M2 macrophage phenotype markers in NASH. A: Increased hepatic expression of the proinflammatory M1 macrophage marker IL-6 (*P = 0.05) in NASH but comparable expression of IL-1β (P = 0.68) in obese subjects with NASH and subjects with simple steatosis. B: Increased mRNA expression of the anti-inflammatory M2 macrophage markers IL-10 (*P = 0.03), dectin-1 (**P = 0.07), and epidermal growth factor-like module containing mucin-like hormone receptor 1 (EMR1) (***P = 0.05) in the liver of subjects with NASH.
Discussion
Inflammation and oxidative stress are thought to be key elements in the progression of NAFLD. We have studied the potential contribution of MPO, an enzyme associated with oxygen/nitrogen species generation and inflammation, to the progression of human NAFLD. The data obtained in this study indicate that there are increased numbers of MPO-positive Kupffer cells in NASH. Furthermore, we show that accumulation of HOCl-modified and nitrated proteins is associated with CXC chemokine expression and infiltration of neutrophils into the liver. The potential role of MPO in NASH is further underscored by the fact that plasma MPO levels were elevated in NASH patients compared with patients with similar body mass index without NASH. Taken together, these results provide new evidence that increased expression and activity of MPO are associated with the perpetuating chronic inflammation that is observed in NASH.
MPO-positive neutrophils have previously been shown to be associated with the presence of oxidized phospholipids, in particular phosphatidylcholine, in steatotic hepatocytes.10 Importantly, oxidized phosphatidylcholine was also detected in Kupffer cells and shown to be more abundant in NASH than in normal livers. Our findings suggest that increased Kupffer cell MPO activity represents an additional source of oxidative damage in NASH. Also, intracellular MPO-mediated lipid peroxidation processes could represent an alternative route for formation of oxidized phospholipids in addition to the scavenger-receptor-mediated pathways that were previously proposed to be responsible.10
Although we have no direct evidence that MPO is involved in protein nitration in NASH, relevant nitration mechanisms via MPO are known to exist.18 The oxidation of nitrite by HOCl generated by the MPO-H2O2-halide system represents one route for nitrotyrosine formation.33,34 Alternatively, the MPO-H2O2 system may use nitrite to generate reactive nitrogen intermediates that promote nitration of proteins and lipid peroxidation.17 Most importantly, lysine-derived chloramines, formed by reaction of HOCl with protein amines, can generate nitrogen-centered radicals35; these radicals are also formed by HOCl-derived modification of phospholipids.36 Interestingly, staining for HOCl-modified epitopes was frequently paralleled by intense staining for nitrotyrosine in our study. Comparable data have been obtained in diseased kidney tissue where lipid peroxidation and HOCl-modified proteins may occur at similar locations.37
Fragmentation of the extracellular matrix by MPO-derived HOCl38,39 has previously been shown to cause activation of hepatic stellate cells, thereby promoting hepatic fibrosis.40 Moreover, lipid peroxidation via tyrosyl radicals and nitrogen dioxide radicals (both formed by the MPO-H2O2 system) increases extracellular matrix synthesis by hepatic stellate cells as well.41 However, the number of MPO-positive cells was not associated with the stage of fibrosis in the present study, suggesting that MPO-derived products play a minor role in the activation of stellate cells in NASH in younger patients. Nevertheless, increased MPO activity in the long run may contribute to the progression of fibrosis.
Like other macrophages, Kupffer cells can be differentially activated according to the inflammatory microenvironment, leading to different biological properties.42 The increased presence of MPO in Kupffer cells in NASH as observed in this study raised the intriguing possibility that Kupffer cells gain M1 macrophage-like proinflammatory properties in NASH, because this type of macrophage is known to generate high amounts of reactive oxygen species.25 The increased expression of the established M1 macrophage marker IL-6 was consistent with this concept. However, expression of IL-1β, another prototypical M1 marker, was not elevated. Moreover, all M2 markers measured in the present study showed substantial up-regulation in NASH, suggesting that part of the inflammatory response of Kupffer cells in NASH is directed toward tissue remodeling. Of note, M2 macrophages produce transforming growth factor-β and other proteins involved in tissue remodeling, such as metalloproteinases 1 and 12.43 As such, these cells may contribute to fibrosis in NASH. In addition, M2 macrophages express many types of scavenger receptors implicated in the uptake of lipids, lipoproteins, and apoptotic cells. Taken together, these characteristics suggest a role for Kupffer cells in liver remodeling and tissue repair after hepatocyte necrosis because of excessive lipid accumulation, although we cannot rule out that hepatic cells other than Kupffer cells contribute to the elevated M2 marker gene expression.
In the present study, we found that hepatic expression of IL-8 and GRO-α was elevated in patients with oxidative hepatocyte damage. Moreover, their expression significantly correlated with neutrophil sequestration. This suggests that these CXC chemokines provide a molecular link between oxidative hepatocyte injury and the recruitment of neutrophils to the liver in NASH. Furthermore, it was recently reported44 that accumulation of triglycerides in hepatocytes causes a dose-dependent increase in IL-8 secretion. Thus, triglyceride-induced IL-8 secretion may be a factor that directly links steatosis and infiltration of the liver by neutrophils. Interestingly, HOCl-modified (lipo)protein has also been reported to promote IL-8 expression in human monocytes.45 Moreover, IL-8 plasma levels are elevated in human NASH.46 Our results suggest that secretion of GRO-α might also be of interest in this respect, and putative effects of triglyceride accumulation on GRO-α secretion by hepatocytes merit further study.
The frequent organization of neutrophils in crown-like structures around steatotic hepatocytes that we observed might be significant because the majority of macrophages in obese adipose tissue are also organized into this type of structure, which is thought to prevent leakage of potentially cytotoxic lipid droplets into the interstitium.47,48 This suggests that excessive fat accumulation in adipose tissue and liver activates a common mechanism that drives infiltration and organization of inflammatory cells into crown-like structures. Because this inflammatory structure is associated with necrotic cell death in adipose tissue,47,48 necrosis may also be a major stimulus for liver inflammation in NASH. The emergence of a chronic inflammatory reaction in response to necrotically dying cells has recently been coupled to the release of so-called damage-associated molecular pattern molecules or alarmins, endogenous proteins that act extracellularly to induce inflammatory responses.49 Interestingly, it has been suggested that oxidative processes are involved in the eradication of damage-associated molecular patterns, thereby modulating the effects of chronic inflammation toward tissue remodeling and repair.50 It is tempting to speculate that MPO-mediated oxidation contributes to this process and complements the tissue repair that is exerted by Kupffer cells with M2 properties.
In conclusion, this is the first study to show that the inflammatory process in NASH is characterized by an increased prevalence of MPO-positive Kupffer cells accompanied by significant MPO-mediated oxidative hepatocyte damage. In view of the association of MPO-mediated hepatocyte damage with both CXC chemokine expression and hepatic neutrophil infiltration, increased MPO activity might be a driving factor underlying the progression of human NASH. MPO could therefore represent a novel therapeutic target51 in human NASH.
Acknowledgments
We thank Niels Mels for technical assistance and Froukje Verdam and Petra Niessen for critical evaluation of the manuscript.
Footnotes
Address reprint requests to Sander S. Rensen, Department of Surgery, Maastricht University Medical Centre, PO Box 616, 6200 MD Maastricht, The Netherlands. E-mail: [email protected].
Supported by Senter Novem Innovatiegerichte Onderzoeks Programmas’ Genomics grant IGE05012 and the Austrian Science Fund (FWF P19074-B05).
Conflict of interest statement: W.A.B. is shareholder of Hycult Biotechnology that provided the anti-HNP1-3 and anti-nitrotyrosine antibodies and the MPO ELISA kit used in this study. These materials are commercially available worldwide.
References
- Shoelson SE, Herrero L, Naaz A. Obesity, inflammation, and insulin resistance. Gastroenterology. 2007;132:2169–2180. [Abstract] [Google Scholar]
- Boden G. Obesity and free fatty acids. Endocrinol Metab Clin North Am. 2008;37:635–646. [Europe PMC free article] [Abstract] [Google Scholar]
- Yamaguchi K, Yang L, McCall S, Huang J, Yu XX, Pandey SK, Bhanot S, Monia BP, Li YX, Diehl AM. Inhibiting triglyceride synthesis improves hepatic steatosis but exacerbates liver damage and fibrosis in obese mice with nonalcoholic steatohepatitis. Hepatology. 2007;45:1366–1374. [Abstract] [Google Scholar]
- Lalor PF, Faint J, Aarbodem Y, Hubscher SG, Adams DH. The role of cytokines and chemokines in the development of steatohepatitis. Semin Liver Dis. 2007;27:173–193. [Abstract] [Google Scholar]
- Ramaiah SK, Jaeschke H. Role of neutrophils in the pathogenesis of acute inflammatory liver injury. Toxicol Pathol. 2007;35:757–766. [Abstract] [Google Scholar]
- Maher JJ, Leon P, Ryan JC. Beyond insulin resistance: innate immunity in nonalcoholic steatohepatitis. Hepatology. 2008;48:670–678. [Europe PMC free article] [Abstract] [Google Scholar]
- Tilg H, Hotamisligil GS. Nonalcoholic fatty liver disease: cytokine-adipokine interplay and regulation of insulin resistance. Gastroenterology. 2006;131:934–945. [Abstract] [Google Scholar]
- Matsuzawa N, Takamura T, Kurita S, Misu H, Ota T, Ando H, Yokoyama M, Honda M, Zen Y, Nakanuma Y, Miyamoto K, Kaneko S. Lipid-induced oxidative stress causes steatohepatitis in mice fed an atherogenic diet. Hepatology. 2007;46:1392–1403. [Abstract] [Google Scholar]
- Seki S, Kitada T, Yamada T, Sakaguchi H, Nakatani K, Wakasa K. In situ detection of lipid peroxidation and oxidative DNA damage in nonalcoholic fatty liver diseases. J Hepatol. 2002;37:56–62. [Abstract] [Google Scholar]
- Ikura Y, Ohsawa M, Suekane T, Fukushima H, Itabe H, Jomura H, Nishiguchi S, Inoue T, Naruko T, Ehara S, Kawada N, Arakawa T, Ueda M. Localization of oxidized phosphatidylcholine in nonalcoholic fatty liver disease: impact on disease progression. Hepatology. 2006;43:506–514. [Abstract] [Google Scholar]
- Edmison J, McCullough AJ. Pathogenesis of nonalcoholic steatohepatitis: human data. Clin Liver Dis. 2007;11:75–104. [Abstract] [Google Scholar]
- Berson A, De Beco V, Letteron P, Robin MA, Moreau C, El Kahwaji J, Verthier N, Feldmann G, Fromenty B, Pessayre D. Steatohepatitis-inducing drugs cause mitochondrial dysfunction and lipid peroxidation in rat hepatocytes. Gastroenterology. 1998;114:764–774. [Abstract] [Google Scholar]
- George J, Pera N, Phung N, Leclercq I, Yun Hou J, Farrell G. Lipid peroxidation, stellate cell activation and hepatic fibrogenesis in a rat model of chronic steatohepatitis. J Hepatol. 2003;39:756–764. [Abstract] [Google Scholar]
- Neuschwander-Tetri BA, Caldwell SH. Nonalcoholic steatohepatitis: summary of an AASLD Single Topic Conference. Hepatology. 2003;37:1202–1219. [Abstract] [Google Scholar]
- Klebanoff SJ. Myeloperoxidase: friend and foe. J Leukoc Biol. 2005;77:598–625. [Abstract] [Google Scholar]
- Davies MJ, Hawkins CL, Pattison DI, Rees MD. Mammalian heme peroxidases: from molecular mechanisms to health implications. Antioxid Redox Signal. 2008;10:1199–1234. [Abstract] [Google Scholar]
- Hazen SL, Zhang R, Shen Z, Wu W, Podrez EA, MacPherson JC, Schmitt D, Mitra SN, Mukhopadhyay C, Chen Y, Cohen PA, Hoff HF, Abu-Soud HM. Formation of nitric oxide-derived oxidants by myeloperoxidase in monocytes: pathways for monocyte-mediated protein nitration and lipid peroxidation in vivo. Circ Res. 1999;85:950–958. [Abstract] [Google Scholar]
- Souza JM, Peluffo G, Radi R. Protein tyrosine nitration—functional alteration or just a biomarker? Free Radic Biol Med. 2008;45:357–366. [Abstract] [Google Scholar]
- Malle E, Waeg G, Schreiber R, Grone EF, Sattler W, Grone HJ. Immunohistochemical evidence for the myeloperoxidase/H2O2/halide system in human atherosclerotic lesions: colocalization of myeloperoxidase and hypochlorite-modified proteins. Eur J Biochem. 2000;267:4495–4503. [Abstract] [Google Scholar]
- Nagra RM, Becher B, Tourtellotte WW, Antel JP, Gold D, Paladino T, Smith RA, Nelson JR, Reynolds WF. Immunohistochemical and genetic evidence of myeloperoxidase involvement in multiple sclerosis. J Neuroimmunol. 1997;78:97–107. [Abstract] [Google Scholar]
- Sugiyama S, Okada Y, Sukhova GK, Virmani R, Heinecke JW, Libby P. Macrophage myeloperoxidase regulation by granulocyte macrophage colony-stimulating factor in human atherosclerosis and implications in acute coronary syndromes. Am J Pathol. 2001;158:879–891. [Europe PMC free article] [Abstract] [Google Scholar]
- Brown KE, Brunt EM, Heinecke JW. Immunohistochemical detection of myeloperoxidase and its oxidation products in Kupffer cells of human liver. Am J Pathol. 2001;159:2081–2088. [Europe PMC free article] [Abstract] [Google Scholar]
- Lumeng CN, Bodzin JL, Saltiel AR. Obesity induces a phenotypic switch in adipose tissue macrophage polarization. J Clin Invest. 2007;117:175–184. [Europe PMC free article] [Abstract] [Google Scholar]
- Odegaard JI, Ricardo-Gonzalez RR, Red Eagle A, Vats D, Morel CR, Goforth MH, Subramanian V, Mukundan L, Ferrante AW, Chawla A. Alternative M2 activation of Kupffer cells by PPARδ ameliorates obesity-induced insulin resistance. Cell Metab. 2008;7:496–507. [Europe PMC free article] [Abstract] [Google Scholar]
- Mantovani A, Sica A, Locati M. New vistas on macrophage differentiation and activation. Eur J Immunol. 2007;37:14–16. [Abstract] [Google Scholar]
- Brunt EM, Janney CG, Di Bisceglie AM, Neuschwander-Tetri BA, Bacon BR. Nonalcoholic steatohepatitis: a proposal for grading and staging the histological lesions. Am J Gastroenterol. 1999;94:2467–2474. [Abstract] [Google Scholar]
- Malle E, Hazell L, Stocker R, Sattler W, Esterbauer H, Waeg G. Immunologic detection and measurement of hypochlorite-modified LDL with specific monoclonal antibodies. Arterioscler Thromb Vasc Biol. 1995;15:982–989. [Abstract] [Google Scholar]
- ter Steege JC, Koster-Kamphuis L, van Straaten EA, Forget PP, Buurman WA. Nitrotyrosine in plasma of celiac disease patients as detected by a new sandwich ELISA. Free Radic Biol Med. 1998;25:953–963. [Abstract] [Google Scholar]
- van Dielen FM, Buurman WA, Hadfoune M, Nijhuis J, Greve JW. Macrophage inhibitory factor, plasminogen activator inhibitor-1, other acute phase proteins, and inflammatory mediators normalize as a result of weight loss in morbidly obese subjects treated with gastric restrictive surgery. J Clin Endocrinol Metab. 2004;89:4062–4068. [Abstract] [Google Scholar]
- Schneider JJ, Unholzer A, Schaller M, Schafer-Korting M, Korting HC. Human defensins. J Mol Med. 2005;83:587–595. [Abstract] [Google Scholar]
- Nijhuis J, Rensen SS, Slaats Y, van Dielen FM, Buurman WA, Greve JW. Neutrophil activation in morbid obesity, chronic activation of acute inflammation. Obesity. 2009 DOI: 10.1038/oby.2009.113. [Abstract] [Google Scholar]
- Willment JA, Lin HH, Reid DM, Taylor PR, Williams DL, Wong SY, Gordon S, Brown GD. Dectin-1 expression and function are enhanced on alternatively activated and GM-CSF-treated macrophages and are negatively regulated by IL-10, dexamethasone, and lipopolysaccharide. J Immunol. 2003;171:4569–4573. [Abstract] [Google Scholar]
- Eiserich JP, Cross CE, Jones AD, Halliwell B, van der Vliet A. Formation of nitrating and chlorinating species by reaction of nitrite with hypochlorous acid: a novel mechanism for nitric oxide-mediated protein modification. J Biol Chem. 1996;271:19199–19208. [Abstract] [Google Scholar]
- Eiserich JP, Hristova M, Cross CE, Jones AD, Freeman BA, Halliwell B, van der Vliet A. Formation of nitric oxide-derived inflammatory oxidants by myeloperoxidase in neutrophils. Nature. 1998;391:393–397. [Abstract] [Google Scholar]
- Hawkins CL, Davies MJ. Hypochlorite-induced oxidation of proteins in plasma: formation of chloramines and nitrogen-centred radicals and their role in protein fragmentation. Biochem J. 1999;340(Pt. 2):539–548. [Europe PMC free article] [Abstract] [Google Scholar]
- Kawai Y, Kiyokawa H, Kimura Y, Kato Y, Tsuchiya K, Terao J. Hypochlorous acid-derived modification of phospholipids: characterization of aminophospholipids as regulatory molecules for lipid peroxidation. Biochemistry. 2006;45:14201–14211. [Abstract] [Google Scholar]
- Grone HJ, Grone EF, Malle E. Immunohistochemical detection of hypochlorite-modified proteins in glomeruli of human membranous glomerulonephritis. Lab Invest. 2002;82:5–14. [Abstract] [Google Scholar]
- Woods AA, Davies MJ. Fragmentation of extracellular matrix by hypochlorous acid. Biochem J. 2003;376:219–227. [Europe PMC free article] [Abstract] [Google Scholar]
- Rees MD, Kennett EC, Whitelock JM, Davies MJ. Oxidative damage to extracellular matrix and its role in human pathologies. Free Radic Biol Med. 2008;44:1973–2001. [Abstract] [Google Scholar]
- Winau F, Quack C, Darmoise A, Kaufmann SH. Starring stellate cells in liver immunology. Curr Opin Immunol. 2008;20:68–74. [Abstract] [Google Scholar]
- MacDonald GA, Bridle KR, Ward PJ, Walker NI, Houglum K, George DK, Smith JL, Powell LW, Crawford DH, Ramm GA. Lipid peroxidation in hepatic steatosis in humans is associated with hepatic fibrosis and occurs predominately in acinar zone 3. J Gastroenterol Hepatol. 2001;16:599–606. [Abstract] [Google Scholar]
- Hashimoto S, Yamada M, Yanai N, Kawashima T, Motoyoshi K. Phenotypic change and proliferation of murine Kupffer cells by colony-stimulating factors. J Interferon Cytokine Res. 1996;16:237–243. [Abstract] [Google Scholar]
- Zeyda M, Stulnig TM. Adipose tissue macrophages. Immunol Lett. 2007;112:61–67. [Abstract] [Google Scholar]
- Joshi-Barve S, Barve SS, Amancherla K, Gobejishvili L, Hill D, Cave M, Hote P, McClain CJ. Palmitic acid induces production of proinflammatory cytokine interleukin-8 from hepatocytes. Hepatology. 2007;46:823–830. [Abstract] [Google Scholar]
- Woenckhaus C, Kaufmann A, Bussfeld D, Gemsa D, Sprenger H, Grone HJ. Hypochlorite-modified LDL: chemotactic potential and chemokine induction in human monocytes. Clin Immunol Immunopathol. 1998;86:27–33. [Abstract] [Google Scholar]
- Kugelmas M, Hill DB, Vivian B, Marsano L, McClain CJ. Cytokines and NASH: a pilot study of the effects of lifestyle modification and vitamin E. Hepatology. 2003;38:413–419. [Abstract] [Google Scholar]
- Cinti S, Mitchell G, Barbatelli G, Murano I, Ceresi E, Faloia E, Wang S, Fortier M, Greenberg AS, Obin MS. Adipocyte death defines macrophage localization and function in adipose tissue of obese mice and humans. J Lipid Res. 2005;46:2347–2355. [Abstract] [Google Scholar]
- Strissel KJ, Stancheva Z, Miyoshi H, Perfield JW, 2nd, DeFuria J, Jick Z, Greenberg AS, Obin MS. Adipocyte death, adipose tissue remodeling, and obesity complications. Diabetes. 2007;56:2910–2918. [Abstract] [Google Scholar]
- Bianchi ME. DAMPs PAM: Ps and alarmins: all we need to know about danger. J Leukoc Biol. 2007;81:1–5. [Abstract] [Google Scholar]
- Lotze MT, Zeh HJ, Rubartelli A, Sparvero LJ, Amoscato AA, Washburn NR, Devera ME, Liang X, Tor M, Billiar T. The grateful dead: damage-associated molecular pattern molecules and reduction/oxidation regulate immunity. Immunol Rev. 2007;220:60–81. [Abstract] [Google Scholar]
- Malle E, Furtmuller PG, Sattler W, Obinger C. Myeloperoxidase: a target for new drug development?. Br J Pharmacol. 2007;152:838–854. [Europe PMC free article] [Abstract] [Google Scholar]
Articles from The American Journal of Pathology are provided here courtesy of American Society for Investigative Pathology
Full text links
Read article at publisher's site: https://doi.org/10.2353/ajpath.2009.080999
Read article for free, from open access legal sources, via Unpaywall:
http://ajp.amjpathol.org/article/S0002944010606591/pdf
Free after 12 months at ajp.amjpathol.org
http://ajp.amjpathol.org/cgi/reprint/175/4/1473.pdf
Free after 12 months at ajp.amjpathol.org
http://ajp.amjpathol.org/cgi/content/full/175/4/1473
Free to read at ajp.amjpathol.org
http://ajp.amjpathol.org/cgi/content/abstract/175/4/1473
Citations & impact
Impact metrics
Article citations
Chinese herbal formula in the treatment of metabolic dysfunction-associated steatotic liver disease: current evidence and practice.
Front Med (Lausanne), 11:1476419, 08 Oct 2024
Cited by: 0 articles | PMID: 39440040 | PMCID: PMC11493624
Review Free full text in Europe PMC
Targeting hepatic macrophages for non-alcoholic fatty liver disease therapy.
Front Cell Dev Biol, 12:1444198, 05 Sep 2024
Cited by: 0 articles | PMID: 39300994 | PMCID: PMC11410645
Review Free full text in Europe PMC
Understanding Macrophage Complexity in Metabolic Dysfunction-Associated Steatotic Liver Disease: Transitioning from the M1/M2 Paradigm to Spatial Dynamics.
Livers, 4(3):455-478, 13 Sep 2024
Cited by: 0 articles | PMID: 39328386 | PMCID: PMC11426415
The mitochondrial TSPO ligand Atriol mitigates metabolic-associated steatohepatitis by downregulating CXCL1.
Metabolism, 159:155942, 12 Jun 2024
Cited by: 0 articles | PMID: 38871077
Novel Mouse Model of Myocardial Infarction, Plaque Rupture, and Stroke Shows Improved Survival With Myeloperoxidase Inhibition.
Circulation, 150(9):687-705, 17 Jun 2024
Cited by: 0 articles | PMID: 38881440
Go to all (164) article citations
Similar Articles
To arrive at the top five similar articles we use a word-weighted algorithm to compare words from the Title and Abstract of each citation.
Neutrophil-derived myeloperoxidase aggravates non-alcoholic steatohepatitis in low-density lipoprotein receptor-deficient mice.
PLoS One, 7(12):e52411, 20 Dec 2012
Cited by: 75 articles | PMID: 23285030 | PMCID: PMC3527496
Decreased nucleotide excision repair in steatotic livers associates with myeloperoxidase-immunoreactivity.
Mutat Res, 736(1-2):75-81, 07 Nov 2011
Cited by: 19 articles | PMID: 22100520
Myeloperoxidase-Hepatocyte-Stellate Cell Cross Talk Promotes Hepatocyte Injury and Fibrosis in Experimental Nonalcoholic Steatohepatitis.
Antioxid Redox Signal, 23(16):1255-1269, 24 Jun 2015
Cited by: 69 articles | PMID: 26058518 | PMCID: PMC4677570
The role of neutrophils in innate immunity-driven nonalcoholic steatohepatitis: lessons learned and future promise.
Hepatol Int, 14(5):652-666, 02 Sep 2020
Cited by: 27 articles | PMID: 32880077
Review
Funding
Funders who supported this work.
Austrian Science Fund FWF (1)
Grant ID: P19074-B05