Abstract
Free full text

Distinct populations of metastases-enabling myeloid cells expand in the liver of mice harboring invasive and preinvasive intra-abdominal tumor
Abstract
The liver is the most common site of adenocarcinoma metastases, even in patients who initially present with early disease. We postulated that immune-suppressive cells in the liver of tumor-bearing hosts inhibit anti-tumor T cells, thereby accelerating the growth of liver metastases. Using models of early preinvasive pancreatic neoplasia and advanced colorectal cancer, aims of this study were to determine immune phenotype, stimulus for recruitment, inhibitory effects, and tumor-enabling function of immune-suppressive cells in the liver of tumor-bearing hosts. We found that in mice with intra-abdominal malignancies, two distinct CD11b+Gr1+ populations with divergent phenotypic and functional properties accumulate in the liver, becoming the dominant hepatic leukocytes. Their expansion is contingent on tumor expression of KC. These cells are distinct from CD11b+Gr1+ populations in other tissues of tumor-bearing hosts in terms of cellular phenotype and cytokine and chemokine profile. Liver CD11b+Gr1+ cells are highly suppressive of T cell activation, proliferation, and cytotoxicity and induce the development of Tregs. Moreover, liver myeloid-derived suppressor cells accelerate the development of hepatic metastases by inactivation of cytotoxic T cells. These findings may explain the propensity of patients with intra-abdominal cancers to develop liver metastases and suggest a promising target for experimental therapeutics.
Introduction
The immune function of the liver is associated primarily with tolerance induction. Several observations link the liver to immunologic anergy. For example, the liver is a frequent site of autoimmune disease, such as autoimmune hepatitis; the liver mediates tolerance to orally ingested antigens; and hepatic allografts—unlike renal, pancreatic, cardiac, or intestinal allografts—are accepted with minimal or no exogenous immune suppression [1]. Recently, the scientific basis for hepatic tolerance has been examined, largely focusing on DC function. For example, tolerogenic DC have been shown to play a role in T cell anergy to liver allografts [2]. Xia et al. [3] reported that the unique hepatic microenvironment programs Lin–CD117+ hematopoietic progenitor differentiation into regulatory DC, responsible for maintaining liver tolerance. We have reported that liver DC, as a result of their immaturity, are poorly immunogenic when compared with spleen DC in a variety of in vitro and in vivo contexts [4]. Further, oral tolerance has been linked to liver sinusoidal endothelial cells and resident hepatic plasmacytoid DC. Using transgenic mice expressing H-2Kb exclusively on endothelial cells, Limmer et al. [5] demonstrated that oral antigen administration leads to induction of anergy in H-2Kb-restricted CD8+ T cells via scavenger liver sinusoidal endothelial cells. Conversely, Goubier et al. [6] reported that liver plasmacytoid DC induce tolerance to orally ingested antigen by active T cell deletion.
One of the most striking clinical observations possibly related to hepatic tolerance is the propensity of intra-abdominal tumors to preferentially metastasize to the liver. In fact, for reasons that are uncertain, tumors originating in the colon, small bowel, pancreas, stomach, or bile ducts metastasize more commonly to the liver than all other organs combined [7]. The liver is the site of “first-pass” for blood-borne cancer cells from the gastrointestinal tract, as the mesenteric blood flow traverses the liver via the portal vein prior to joining the systemic venous return via the hepatic veins. However, within moments after leaving the mesenteric circulation, neoplastic cells are dispersed throughout the general circulation [8]. It has also been suggested that the complex network of the liver sinusoidal endothelial system “traps” blood-borne micrometastases for nonspecific mechanical reasons [9, 10]. However, this theory has neither been proven definitively nor debunked. Furthermore, the fact that lymphatic metastases, in addition to hematogenous spread, also populate disproportionately to the liver suggests that this phenomenon is unlikely governed entirely by anatomic constraints.
We found that even in animals with early PanIN lesions, a phenotypically distinct population of immune-suppressive CD11b+Gr1+ cells expands in the liver. In animals with advanced i.p. tumor, CD11b+Gr1+ cells expand from a baseline of 1–2% to become >50% of hepatic NPC. Compared with MDSC in lymphoid organs and tumor-infiltrating MDSC, liver CD11b+Gr1+ cells are phenotypically and functionally distinct. They contain a greater CD11b+Gr1inter fraction, exhibiting monocyte morphology, express higher Ly6C and CD31 but lower Ly6G and MHC II, and produce robust levels of proinflammatory and regulatory cytokines. Liver CD11b+Gr1+ cells are highly suppressive of T cell proliferation in vitro and in vivo, inhibit T cell cytotoxicity, and induce Treg development. Moreover, in adoptive transfer and in situ models, liver CD11b+Gr1+ cells from animals with intra-abdominal cancer accelerate the growth of liver metastases by inactivating effector T cells. This study expands our understanding of immune-suppressive cells in the liver of tumor-bearing hosts and provides an immunologic basis for the eventual development of hepatic metastases in individuals harboring localized intra-abdominal cancer.
MATERIALS AND METHODS
Animals and cancer models
Male C57BL/6 (H-2Kb), BALB/c (H-2Kd), CD45.1 (B6.SJL-Ptprca/BoAiTac), and OTI (B6.Cg-RAG2tm1Fwa-TgN) mice (4–8 weeks old) were purchased from Taconic (Germantown, NY, USA). B6.129S2(C)-Il8rbtm1Mwm/J mice lacking the CXCR2 receptor were purchased from Jackson Laboratories (Bar Harbor, ME, USA). Mice, which develop pancreatic neoplasia endogenously by expressing a single mutant Kras allele in progenitor cells of the pancreas (gift of David Tuveson, University of Pennsylvania, Philadelphia, PA, USA), were generated by breeding LSL-KrasG12D mice with p48Cre mice, which express Cre recombinase from a pancreatic progenitor-specific promoter [11, 12]. For our orthotopic tumor model, C57BL/6 mice were challenged i.p. with MCA38 colorectal cancer cells (5×105; gift of Nicholas P. Restifo, National Cancer Institute, Bethesda, MD, USA). Mice were housed in a pathogen-free environment. The New York University School of Medicine Institutional Animal Care and Use Committee (New York, NY, USA) approved animal procedures.
Cellular isolation
Liver NPC were isolated as described previously [13]. Briefly, the portal vein of mice was cannulated and infused with 1% Collagenase IV (Sigma Chemical Co., St. Louis, MO, USA). The liver was then removed and minced. Cells were centrifuged at low speed (30 g) to exclude hepatocytes followed by high-speed (300 g) centrifugation to isolate the NPC, which were then enriched further over a 40% Optiprep (Sigma Chemical Co.) gradient. Liver CD11b+Gr1+ cells were purified by FACS sorting using a MoFlo cell sorter (Beckman Coulter, Fullerton, CA, USA). Tumor-associated CD11b+Gr1+ cells were purified from among tumor-infiltrating leukocytes by enzymatic and mechanical digestion of i.p. tumor nodules, as described previously [14], followed by FACS sorting. For splenic T cell (CD4+, CD8+, or CD90+) or DC (CD11c+) isolation, single-cell suspensions of splenocytes were prepared by positive selection using immunomagnetic microbeads and passage over LS columns (Miltenyi Biotec, Bergisch-Gladbach, Germany). BM suspensions were generated as described [15]. Spleen and BM CD11b+Gr1+ cells were purified by FACS.
Flow cytometry
Flow cytometry was performed on a FACSCaliber instrument (BD Biosicences, Franklin Lakes, NJ, USA) after incubating 5 × 105 cells/tube with 1 μg Fc block (clone 2.4G2; Monoclonal Antibody Core Facility, Memorial Sloan-Kettering Cancer Center, New York, NY, USA) and then labeling with 1 μg fluorescently conjugated antibody. Dead cells were excluded by staining with 7-amino-actinomycin D (BD Biosciences). Cells were stained for MHC class I (H-2Kb), MHC class II (I-Ab), CD1d (1B1), CD3ε (17A2), CD4 (RM4-5) CD8α (53–6.7) CD11b (M1/70), CD11c (HL3), CD25 (PC61.5), CD31 (MEC 13.3), CD40 (HM40-3), CD44 (IM7), CD45.2 (104), CD69 (H1.2F3), CD178 (MFL3), CD195 (2D7/CCR5), IL-4Rα (mIL4R-M1), Ly6C (HK1.4), Ly6G (1A8), Gr1 (RB6-8C5), F4/80 (BM8), Mac-3 (M3/84), or Foxp3 (FJK-16 s; all from BD Biosciences). MFI was calculated for each surface marker. To measure cellular turnover in vivo, mice were injected i.p. with fluorescently conjugated BrdU (1 mg; BD Biosciences). Thirty-six hours later, leukocytes were harvested from multiple tissues, fixed, permeabilized, and analyzed by flow cytometry.
Cytokine and reactive oxygen species measurement
For cytokine analysis, cellular suspensions were cultured in complete medium (RPMI 1640 with 10% heat-inactivated FBS, 2 mM L-glutamine, 100 U/ml penicillin, 100 μg/ml streptomycin, and 0.05 mM 2-ME) for 24 h before supernatant harvest and assay using the Milliplex Map Immunoassay (Millipore, Billerica, MA, USA) and the Luminex 200 system (Luminex, Austin, TX, USA), according to the respective manufacturer’s protocol. NO production in 24 h conditioned media was measured using the Griess reaction, according to the manufacturer’s protocol (Cayman Chemical, Ann Arbor, MI, USA). All cellular suspensions were plated at a concentration of 1 × 106 cells/ml unless specified otherwise.
T cell assays—in vitro
T cell proliferation assays were performed as described with modifications [16]. To induce proliferation by CD3 ligation, T cells (1×105) were cultured with plate-bound anti-CD3 (BD Biosciences), supplemented with anti-CD28 (1 μg/ml; BD Biosciences). To induce T cell proliferation in response to mitogen, T cells (1×105) were cultured with Con A (5 μg/ml; Sigma Chemical Co.). For antigen-specific T cell proliferation, CD8+ OTI TCR-transgenic T cells (1×105) were stimulated with Ova (10 μg/ml; AnaSpec, San Jose, CA, USA). All assays were performed in triplicate in 96-well dishes. In each experiment, on Day 2 or 3, T cells were pulsed with 3[H]-thymidine for an additional 24 h. To assay inhibition of T cell proliferation, freshly isolated CD11b+Gr1+ cells were irradiated (3000 rads) and added to T cells in various concentrations. In some experiments, anti-IL-10 mAb (10 μg/ml; clone JES5-16E03, eBioscience, San Diego, CA, USA) was added. In selected experiments, CD11b+Gr1+ cells (1×105) were cultured with equal numbers of T cells for various time-points before analysis of T cell surface phenotype by flow cytometry.
T cell assays—in vivo
To determine the effect of CD11b+Gr1+ cells on T cell proliferation in the liver, naïve C57BL/6 mice were administered 2 × 106 CD8+ Ova-restricted OTI T cells i.v. and then immunized i.v. 24 h later with splenic DC.Ova (10 μg/ml) or DC.Ova + liver CD11b+Gr1+ cells (5×105) from tumor-bearing hosts. At 90 h, hepatic NPC were isolated from recipient mice, and the number of CD8+ Ova Tetramer+ (Vaccine and Cell Therapy Core Facility, New York University) T cells was measured by flow cytometry.
CTL assays
CTL assays were performed as described [16]. Briefly, mice were immunized twice at weekly intervals with 1 × 105 splenic DC.Ova. One week after the second treatment, splenocytes from immunized mice were harvested and restimulated at 5 × 106 cells/well in 24-well plates with Ova (10 μg/ml) for 5 days. Effectors were then harvested and tested against 1 × 104 51 [Cr]-labeled EG7 or EL4 (both American Type Culture Collection, Manassas, VA, USA) targets in various E:T ratios for 6 h in 96-well dishes. In selected assays, CD11b+Gr1+ cells from the liver of tumor-bearing hosts were added.
Tumor experiments
Tumor experiments were performed as described previously with modifications [15, 17]. For s.c. models, C57BL/6 mice were anesthetized and challenged with 2 × 105 EG7 cells s.c. in their back. In selected mice, EG7 cells were mixed with OTI T cells (2×105) immediately before transfer. In additional animals, liver MDSC (5×105) were added to the EG7 + OTI mixture. Day of tumor development was recorded. In the liver metastases models, mice were challenged with a portal venous injection of 2 × 104 B16.Ova cells (gift of Ronald P. DeMatteo, Memorial Sloan-Kettering Cancer Center), alone or mixed with OTI T cells (2×105). In selected mice, tumor cells were also mixed with liver MDSC (6×105) immediately before inoculation. Time to death from liver metastases was recorded. To study liver metastases development in situ in tumor-bearing hosts, C57BL/6 mice were administered 8 × 104 B16 cells into their portal venous system via intrasplenic injection, followed by splenectomy. Selected mice were challenged simultaneously with an i.p. injection of 5 × 105 MCA38 cells. To deplete Gr1+ cells in vivo, RB6-8C5 was used (thrice weekly injections of 150 μg; Monoclonal Antibody Core Facility, Memorial Sloan-Kettering Cancer Center). On Day 21, mice were killed, and the number of liver tumors was counted.
Histology and immunofluorescence
For histological analysis, specimens were fixed with 10% buffered formalin, dehydrated in ethanol, and then embedded with paraffin and stained with H&E. For cytospin analysis, FACS-sorted CD11b+Gr1+ cells were centrifuged onto ProbeOn slides (Fisher Biotech, Pittsburg, PA, USA) and stained with H&E. Photographs were taken with a Leica DM2M microscope (Leica Microsystems, Bannockburn, IL, USA) and an Optronics digital camera (Optronics, Goleta, CA, USA). For immunofluorescence studies, frozen sections of liver were stained with anti-Gr-1 and anti-CD11b (eBioscience). Images were captured on a Zeiss Axiovert 200M (Carl Zeiss, Peabody, MA, USA) fluorescence microscope.
Statistics
Data are presented as mean ± sem. Survival was measured according to the Kaplan-Meier method. Statistical significance was determined by the Student’s t-test and the log-rank test using SPSS statistical software (SPSS Inc., Chicago, IL, USA). P < 0.05 was considered significant.
RESULTS
CD11b+Gr1+ cells expand in the livers of mice with intra-abdominal cancer
To investigate immune-suppressive cells in the liver of mice with advanced cancer, we isolated liver NPC from naive mice and mice harboring i.p. MCA38 tumors and analyzed them for coexpression of CD11b and Gr1. Although CD11b+Gr1+ cells constituted only 1–2% of NPC in normal liver, they expanded to 50–70% in mice with advanced tumor (Fig. 1A). Consistent with this observation, frozen sections of liver from mice-bearing intra-abdominal MCA38 tumor demonstrated abundant infiltration of cells staining positive for CD11b and Gr1, and liver sections from normal mice had almost no staining for these markers (Fig. 1B). Conversely, in vivo administration of MCA38 conditioned media did not result in CD11b+Gr1+ cellular expansion (not shown). Furthermore, s.c. tumor challenge with MCA38 (5×105) did not result in substantial expansion of hepatic CD11b+Gr1+ cells, suggesting that this phenomenon is specific to intra-abdominal tumor growth (Fig. 1C). To determine the time course of expansion of CD11b+Gr1+ leukocytes in the liver, mice were killed at various time intervals after i.p. challenge with MCA38. Expansion of CD11b+Gr1+ cells was minimal on Day 6 but increased to 30% of NPC by Day 10 and peaked at ~3 weeks after tumor challenge, when mice were visibly ill from tumor burden (Fig. 1D). Furthermore, although the BM contained >25% CD11b+Gr1+ cells at a baseline, the relative expansion of CD11b+Gr1+ cells in the liver of tumor-bearing hosts exceeded other compartments (Fig. 1D). Cellular turnover of CD11b+Gr1+ cells was also higher in the liver than in other compartments of i.p. tumor-bearing hosts, as measured by in vivo uptake of BrdU (Fig. 1E). To exclude the possibility that occult liver metastases induced intra-hepatic CD11b+Gr1+ cellular expansion, mice were seeded i.p. with MCA38 cells expressing YFP. On Day 21, livers were analyzed by flow cytometry. No YFP+ cells were seen. Similarly, there was no outgrowth of tumor cells in vitro after culture of liver cellular suspensions from mice with i.p. tumor (both not shown).
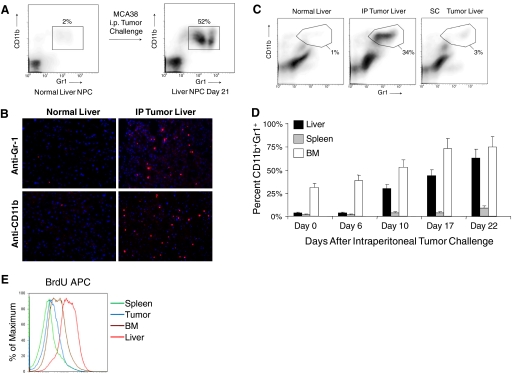
CD11b+Gr1+ cells expand in the liver of mice harboring intra-abdominal tumor. (A) C57BL/6 mice were seeded with MCA38 i.p. Liver NPC were then harvested on Day 21 and analyzed for CD11b and Gr1 expression by flow cytometry. Data are representative of experiments done more than five times using at least three mice in each experiment. (B) s.c. MCA38 administration resulted in minimal expansion of intra-hepatic myeloid cells by Day 14. Data are representative of experiments done twice using three mice each time. IP, i.p. (C) Immunoflourescent microscopic analysis of the liver in mice-bearing MCA38 tumor (Day 14) using antibodies against Gr-1 and CD11b. (D) Time course of CD11b+Gr1+ cellular expansion in multiple compartments of tumor-bearing hosts. Cells were harvested at various time intervals after i.p. MCA38 challenge and analyzed for CD11b and Gr1 coexpression by flow cytometry. CD11b+Gr1+ cells underwent the highest relative increase in the liver (P<0.05). Averages of two to three mice/time-point are shown. This experiment was repeated three times with consistent results. (E) To determine the turnover of CD11b+Gr1+ cells in various compartments, on Day 14 after tumor challenge, i.p. MCA38-bearing mice received BrdU (100 μg). Thirty-six hours later, CD11b+Gr1+ were harvested from various compartments and incorporation of BrdU determined by flow cytometry. This experiment was repeated three times using three mice/group.
To determine whether hepatic CD11b+Gr1+ cells also expand in mice with early, preinvasive intra-abdominal cancers, we analyzed LSL-KrasG12D mice that express a mutant Kras allele in progenitor cells of the pancreas [12]. By 3 months of age, these mice develop hyperplastic mucinous pancreatic ducts consistent with early PanIN lesions. More advanced PanIN lesions with cellular cribiform morphology were observed by 9 months (Fig. 2). This endogenous pancreatic neoplasia model reflects the stepwise progression of human gastrointestinal carcinogenesis [18]. There was a modest expansion of CD11b+Gr1+ cells in the liver by 3 months of life, which increased to >20% by 9 months (Fig. 2). In contrast, splenic CD11b+Gr1+ cellular expansion was not evident until 9 months (not shown). Immunofluorescence studies confirmed expansion of CD11b+ and Gr1+ cells in the liver of mice with early, preinvasive pancreatic neoplasia (Supplemental Fig. 1).
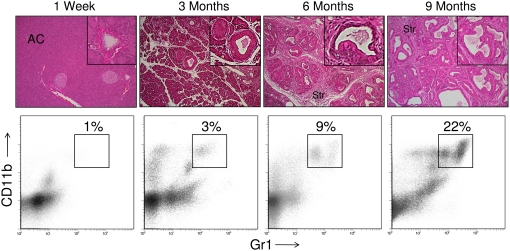
CD11b+Gr1+ cells expand in the liver of mice with PanIN lesions. Mice expressing a single mutant Kras allele in the progenitor cells of the pancreas develop a stepwise progression from normal pancreatic parenchyma with organized acinar cell (AC) architecture to preinvasive pancreatic cancer. Sporadic, early PanIN lesions are evident at 3 months, followed by complete replacement of acinar tissue with PanIN lesions by 9 months. Progression of the disease is accompanied by a large stromal reaction (Str). In livers of LSL-KrasG12D mice, there is a gradual expansion of the CD11b+Gr1+ population. These data are representative of experiments repeated more than three times using two to four mice/time-point. M.
Liver CD11b+Gr1+ cells are phenotypically distinct from MDSC in other tissues
Two distinct populations of CD11b+Gr1+ cells were recruited to the liver in mice with intra-abdominal tumor: a CD11b+Gr1inter population and a CD11b+Gr1high population (Fig. 3A). The CD11b+Gr1inter population was composed almost exclusively of NPC with low side-scatter, and the CD11b+Gr1high population was composed predominantly of cells exhibiting high side-scatter. Moreover, nearly all NPC with high side-scatter (~85%) exhibited the CD11b+Gr1high phenotype, and only a fraction of NPC with low side-scatter properties (~30%) was CD11b+Gr1inter (Fig. 3A). In total, 2–4 × 106 liver NPC/tumor-bearing mouse expressed the CD11b+Gr1inter phenotype, and 0.5–1 × 106 cells expressed the CD11b+Gr1high phenotype.
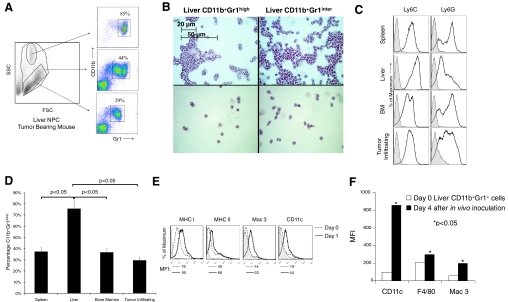
Liver CD11b+Gr1+ cells exhibit distinct phenotypic and morphologic properties. (A) Liver NPC were harvested from mice with i.p. MCA38 tumors (Day 17). Distinct CD11b+Gr1high [high side-scatter (SSC)] and CD11b+Gr1inter (low side-scatter) populations were expanded in the liver. Data are representative of experiments done more than five times using more than three mice in each experiment. FSC, Forward-scatter. (B) CD11b+Gr1high or CD11b+Gr1inter liver populations were FACS-sorted and then cytospun at high density (5×106 cells/ml; upper panels) or low density (5×105 cells/ml; lower panels), stained with H&E, and visualized by light microscopy (original, 20×). (C) Phenotypic analysis of Ly6C and Ly6G expression on bulk CD11b+Gr1+ cells from the spleen, liver, BM, or tumor in mice bearing advanced i.p. MCA38 tumors. Shaded histograms represent isotype controls. (D) CD11b+Gr1inter cellular frequency in various tissue compartments of tumor-bearing hosts (P<0.05). (E) Expression of surface markers on hepatic CD11b+Gr1+ cells from mice with advanced MCA38 tumor after overnight culture. Shaded histograms represent isotype controls. MFIs are indicated below the histograms for each respective analysis. (F) Alterations in selected surface marker expression on hepatic CD11b+Gr1+ cells from C57BL/6 tumor-bearing mice after CFSE labeling and injection into CD45.1+ naïve mice. Data shown are representative of experiments repeated more than three times using three mice/data point. *, P < 0.05.
We analyzed the two hepatic CD11b+Gr1+ populations from mice with i.p. MCA38 by light microscopy. The CD11b+Gr1inter population was composed mostly of cells with rounded or oval-shaped nuclei characteristic of immature monoctyes. Conversely, CD11b+Gr1high cells exhibited dumbbell-shaped nuclei characteristic of PMNs (Fig. 3B). The surface phenotype of the CD11b+Gr1high and CD11b+Gr1inter cells was also divergent. CD11b+Gr1inter cells expressed higher Ly6C, and Ly6G expression was higher in the CD11b+Gr1high population. In addition, CD40 expression was higher on CD11b+Gr1high cells, and the CD11b+Gr1inter cells expressed higher MHC I, F4/80, and CD31 (Supplemental Fig. 2). Similar phenotypic findings were seen in the endogenous model of pancreatic neoplasia (not shown).
We next determined whether liver CD11b+Gr1+ cells were distinct from CD11b+Gr1+ cells infiltrating the spleen, BM, or tumor. Liver CD11b+Gr1+ cells expressed higher levels of Ly6C but lower Ly6G compared with MDSC populations in other tissues (Fig. 3C). Consistent with these findings, in mice with i.p. MCA38 tumor, liver CD11b+Gr1+ cells contained a considerably higher fraction of CD11b+Gr1inter cells (75%) compared with spleen (37%), BM (37%), or tumor-associated CD11b+Gr1+ cells (30%; Fig. 3D). We compared further the detailed surface phenotype of liver CD11b+Gr1+ cells with their splenic counterparts and found that liver CD11b+Gr1+ cells express lower MHC I and MHC II but higher CD31, F4/80, and CD195 (Supplemental Fig. 3).
Liver CD11b+Gr1+ develop features of APC
To determine the cellular fate of liver CD11b+Gr1+ cells in animals with intra-abdominal cancers, we FACS-sorted liver CD11b+Gr1+ cells from i.p. tumor-bearing mice and cultured them in complete media without exogenous stimulation. After 24 h, hepatic CD11b+Gr1+ cells increased expression of MHC class I and II, as well as DC (CD11c) and macrophage (Mac-3) differentiation markers (Fig. 3E). To confirm the in vitro findings, CD11b+Gr1+ cells were harvested from the livers of CD45.2+ C57BL/6 mice with intra-abdominal MCA38 tumors, labeled with fluorescent dye, reinjected i.v. into naïve, congenic CD45.1+ mice, and reharvested from the liver 4 days later for phenotypic analysis. Again, expression of DC and macrophage differentiation markers increased on the recovered hepatic CD45.2+CD11b+Gr1+ cells (Fig. 3F).
Tumor production of KC is required for hepatic CD11b+Gr1+ cell expansion
As KC is a powerful chemoattractant for granulocytes [19], we postulated that tumor-derived KC may be required for hepatic CD11b+Gr1+ cellular expansion. To test this, we cultured MCA38 tumor cells and single cell suspensions of collagenase-digested pancreata from 6-month-old wild-type or LSL-KrasG12D mice and measured KC produced in culture. MCA38 tumor and PanIN lesions produced high levels of KC (Fig. 4A). Furthermore, i.p. MCA38 tumor challenge in mice lacking the KC receptor diminished CD11b+Gr1+ cellular expansion by >75% (Fig. 4B).
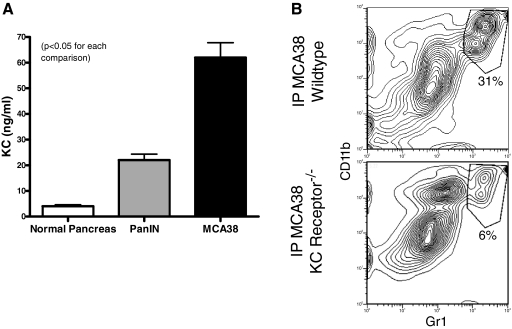
KC receptor ligation is required for CD11b+Gr1+ cellular expansion in the liver. (A) Single cell suspensions of wild-type pancreas, pancreas from LSL-KrasG12D mice with endogenous PanIN lesions, or MCA38 cells were cultured (1×106 cells/ml for 24 h) before supernatant harvest and analysis of KC levels. PanIN and MCA38 cells produced high levels of KC. Average of triplicates are shown (P<0.05). (B) C57BL/6 wild-type and B6.129S2(C)-Il8rbtm1Mwm mice (which are deficient in the KC receptor) were challenged with i.p. MCA38 before sacrifice on Day 14. i.p. tumor growth was similar in both groups (not shown). However, hepatic CD11b+Gr1+ cells expanded only in wild-type mice. Experiments were repeated twice with two mice/group.
Liver CD11b+Gr1+ cells in intra-abdominal tumor-bearing hosts produce robust levels of regulatory and proinflammatory cytokines
To assess the immune regulatory potential of liver Gr1+CD11b+ cells, we tested their production of proinflammatory and regulatory cytokines. Liver or spleen Gr1+CD11b+ cells from naïve and tumor-bearing mice were cultured in conditioned media, and supernatants were assayed. In the endogenous, preinvasive pancreatic cancer (Fig. 5, A and B) and the orthotopic colorectal tumor (Fig. 5, C and D) models, liver Gr1+CD11b+ cells produced high levels of numerous cytokines and chemokines, including IL-1α, IL-1β, IL-6, IL-10, TNF-α, G-CSF, MCP-1, IP-10, LIX, KC, RANTES, MIP-1α, MIP-1β, and MIP-2. Conversely, Gr1+CD11b+ splenocytes from the same mice were nonproductive. Taken together, these data, along with the surface phenotype findings (Fig. 3, C and D, and Supplemental Fig. 3), suggest that hepatic Gr1+CD11b+ cells of tumor-bearing hosts are distinct from Gr1+CD11b+ cells in other tissues. Notably, however, liver and spleen Gr1+CD11b+ cells produced similar levels of NO (Supplemental Fig. 4) and took up 3H-arginine at similar rates (not shown).
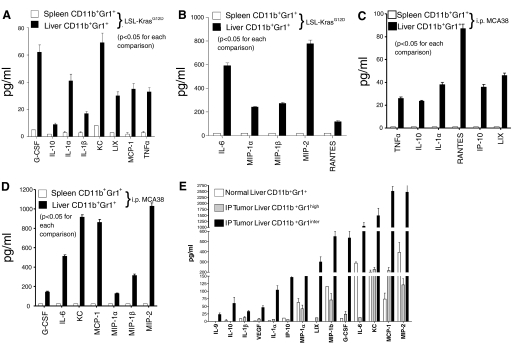
Liver CD11b+Gr1+ cells from tumor-bearing hosts produce augmented levels of inflammatory mediators compared with MDSC in other compartments. (A–D) Liver and spleen CD11b+Gr1+ cells were isolated from (A and B) 9-month-old LSL-KrasG12D mice harboring preinvasive pancreatic cancer or (C and D) C57BL/6 mice with advanced MCA38 tumor and cultured for 24 h at a concentration of 1 × 106 cells/ml. Cytokine and chemokine levels were measured in cell culture supernatant (P<0.05 for all tests). (E) Liver CD11b+Gr1inter and CD11b+Gr1high cells from mice with i.p. MCA38 tumors as well as bulk CD11b+Gr1+ cells from untreated mice were cultured in conditioned media and tested from cytokine and chemokine production. The CD11b+Gr1inter population was the cellular subgroup responsible for the elevated levels of inflammatory mediators in the hepatic CD11b+Gr1+ population in tumor-bearing hosts (P<0.05 for all tests). Cytokine experiments were repeated more than five times with similar results using an average of three mice/group.
To determine the relative contribution of hepatic CD11b+Gr1inter and CD11b+Gr1high cells to the inflammatory cytokine milieu produced by bulk liver CD11b+Gr1+ cells, we FACS-sorted both populations from livers of mice harboring i.p. MCA38 tumor and cultured them as above. Surprisingly, the production of inflammatory mediators by liver CD11b+Gr1+ cells from tumor-bearing hosts was attributable exclusively to the CD11b+Gr1inter population. Conversely, the CD11b+Gr1high population produced similar levels of inflammatory mediators as bulk CD11b+Gr1+ cells from normal liver (Fig. 5E). To determine whether differences in the CD11b+Gr1inter subset composition accounted for the higher levels of inflammatory mediators produced by liver CD11b+Gr1+ cells compared with spleen, we FACS-sorted CD11b+Gr1inter populations from both organs of MCA38-bearing mice and measured TNF-α, IL-6, and MCP-1 levels in culture. However, liver CD11b+Gr1inter cells produced higher levels of these inflammatory mediators than CD11b+Gr1inter cells from the spleen, suggesting that the functional differences between liver and spleen CD11b+Gr1+ cells were not attributable to relative numbers CD11b+Gr1inter cells (Supplemental Fig. 5).
Liver MDSC inhibit T cell proliferation
To test the immune-suppressive capabilities of liver CD11b+Gr1+ cells, we performed proliferation assays using CD90+ spleen T cells in the presence or absence of liver CD11b+Gr1+ cells from mice with advanced i.p. MCA38 tumors. At high concentrations, liver CD11b+Gr1+ populations had a dramatic suppressive effect on T cell proliferation and were partially suppressive at intermediate concentrations (Fig. 6A). Similarly, hepatic MDSC were highly inhibitory to T cell proliferation in response to mitogen, even when compared with MDSC from other compartments (Fig. 6B). To test the capacity of liver MDSC to suppress antigen-restricted T cell proliferation, OTI T cells were stimulated with cognate peptide for 3 days before pulsing with 3H-thymidine. Under these conditions, liver CD11b+Gr1+ cells from mice with i.p. MCA38 inhibited T cell proliferation even at low concentrations (Fig. 6C). These findings suggest that antigen-specific T cell proliferation is more sensitive to inhibition by liver MDSC.
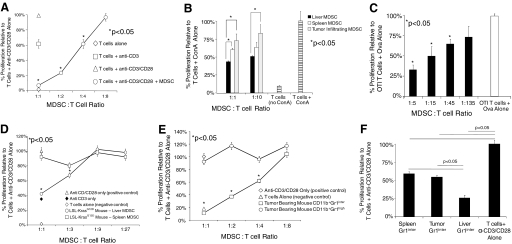
Liver CD11b+Gr1+ cells from intra-abdominal tumor-bearing hosts inhibit T cell proliferation to a variety of stimuli. (A) Splenic T cells (1×105) were cultured alone or were stimulated with plate-bound anti-CD3, supplemented with anti-CD28 (1 μg/ml). To test their inhibitory capabilities, liver CD11b+Gr1+ cells from mice harboring advanced i.p. (Day 21) MCA38 tumors were added in various concentrations. (B) To test the inhibitory effects of MDSC on T cell proliferation in response to mitogen, T cells were cocultured with Con A (5 μg/ml), alone or with Con A + CD11b+Gr1+ cells from various compartments of i.p. tumor-bearing hosts (maximal CPM=7×103). (C) To test liver MDSC inhibition of antigen-restricted T cell proliferation, CD11b+Gr1+ cells from the livers of mice harboring i.p. MCA38 tumors were cultured with OTI T cells (1×105) and Ova (10 μg/ml) for 72 h before pulsing with 3[H]-thymidine (maximal CPM=6×104). (D) Liver and spleen MDSC from mice with preinvasive pancreatic cancer were tested for their capacity to inhibit T cell proliferation to anti-CD3/CD28 as above. (E) Hepatic CD11b+Gr1inter and CD11b+Gr1high populations of i.p. MCA38-bearing mice were sorted before use in anti-CD3/CD28 proliferation assays. (F) The inhibitory capacity of spleen, liver, and tumor-infiltrating CD11b+Gr1inter populations was compared directly in an anti-CD3/CD28 T cell proliferation assay using a 2:1 T cell:MDSC ratio (maximal CPM=2–4×104 for anti-CD3/CD28 proliferation assays). All assays shown were performed in triplicate and repeated three to six times using two to four mice/group. *, P < 0.05.
To determine whether resident liver CD11b+Gr1+ cells from mice with preinvasive tumors are also inhibitory, CD11b+Gr1+ cells were harvested from the livers of 9-month-old LSL-KrasG12D mice and tested for their ability to inhibit T cell proliferation. Consistent with our previous results, liver CD11b+Gr1+ cells, but not spleen-derived CD11b+Gr1+ cells, inhibited T cell proliferation at the two highest concentrations tested (Fig. 6D).
CD11b+Gr1inter but not CD11b+Gr1high liver MDSC exert T cell inhibition
As hepatic CD11b+Gr1inter cells produced high levels of immune-regulatory cytokines, we postulated that the CD11b+Gr1inter cells would have the greatest inhibitory phenotype. To test this, we repeated the anti-CD3/CD28 T cell proliferation assay, including FACS-sorted CD11b+Gr1inter or CD11b+Gr1high cells. Supporting our hypothesis, CD11b+Gr1inter cells were highly suppressive of T cell proliferation, and CD11b+Gr1high cells were completely noninhibitory (Fig. 6E). In addition, when comparing the inhibitory capability of liver CD11b+Gr1inter cells directly with CD11b+Gr1inter populations from other compartments of tumor-bearing hosts, the liver CD11b+Gr1inter population was most inhibitory (Fig. 6F).
As IL-6 and MCP-1 are associated with tumor recurrence in the liver [20, 21] and are produced at markedly elevated levels by liver CD11b+Gr1+ cells in mice with intra-abdominal tumors (Fig. 5), we tested whether liver MDSC from mice lacking expression of IL-6 or MCP-1 were less able to suppress T cell proliferation in vitro. However, hepatic MDSC from tumor-bearing mice lacking IL-6 or MCP-1 expression were equally immune-suppressive as wild-type MDSC (not shown). Furthermore, although IL-10 is produced at markedly elevated levels by liver MDSC (Fig. 5) and is a powerful negative regulator of T cell stimulation [22], blockade of IL-10 using a mAb did not mitigate the in vitro inhibitory function of liver MDSC (not shown). Similarly, conditioned media from MCA38 cells alone were not suppressive of T cell proliferation in response to CD3/CD28 ligation (not shown).
Liver MDSC prevent CTL-mediated tumor lysis
To determine whether liver MDSC can prevent CD8+ T cell-mediated cytotoxicity, we used them in a CTL assay. Naïve mice were immunized twice at weekly intervals with normal spleen DC.Ova. One week after the second immunization, spleens were harvested, restimulated in vitro, and then tested against 51[Cr]-labeled EG7 targets. This strategy resulted in considerable target lysis (Fig. 7). However, addition of liver CD11b+Gr1+ cells from mice with i.p. MCA38 abrogated lytic function, suggesting that liver MDSC can block CD8+ T cell-mediated cytotoxicity (Fig. 7). Lysis of EL4 targets, which do not produce OVA, was minimal, confirming the antigen specificity of the assay (not shown).
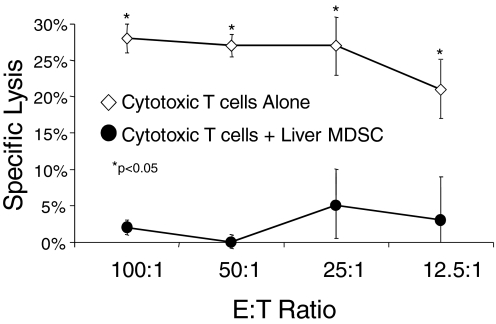
Liver MDSC inhibit CTL-mediated cytolysis. Splenocytes from mice twice immunized with DC.Ova were harvested 1 week after the second immunization and restimulated in vitro with Ova for 5 days before cytolysis assay using EG7 targets. In parallel, hepatic CD11b+Gr1+ cells from i.p. MCA38-bearing hosts were mixed with the restimulated splenocytes in a 1:1 ratio before assay. MDSC eliminated CTL lysis (P<0.05 for all E:T ratios). Experiments were done in triplicate using three mice/group and repeated twice with similar results. *, P < 0.05.
Liver MDSC prevent activation of T cell phenotype and induce Treg differentiation
To explore further the cellular mechanism of T cell suppression by resident liver MDSC, we examined whether MDSC directly prevented T cells from activation upon exogenous stimulation. CD8+ splenic T cells were cultured for 24 h alone or stimulated by CD3/CD28 ligation as above. In selected wells, equal numbers of liver CD11b+Gr1+ cells from mice with advanced i.p. MCA38 tumors were added. Liver MDSC partially prevented up-regulation of CD25, CD44, and CD69 in response to CD3/CD28 ligation (Fig. 8A). Conversely, liver CD11b+Gr1+ cells from normal mice had no effect (not shown).
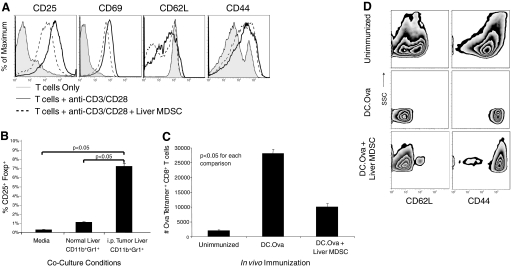
Liver MDSC prevent T cell activation in vitro and in vivo. (A) CD8+ T cells were cultured in 96-well plates alone, with plate-bound anti-CD3/CD28, or with anti-CD3/CD28 + liver CD11b+Gr1+ cells from i.p. tumor-bearing hosts in a 1:1 MDSC:T cell ratio for 24 h before analysis of T cell activation marker expression. (B) CD4+ T cells were harvested from spleens of naive mice and cultured for 4 days alone, with CD11b+Gr1+ cells from normal C57BL/6 mouse liver or with CD11b+Gr1+ cells from the livers of C57BL/6 mice harboring advanced i.p. MCA38 tumors. On Day 5, flow cytometry was performed. CD3+CD4+ T cells were gated and the fraction of CD4+CD25+Foxp+ Tregs determined. Coexpression of CD25 and Foxp was highest on CD4+ T cells cultured with liver CD11b+Gr1+ cells from tumor-bearing hosts (P<0.05). (C) To determine whether liver MDSC prevent T cell proliferation in vivo, OTI T cells (2×106) were transferred to naïve mice, which were then reinjected 24 h later with saline or DC.Ova (1×106) or DC.Ova + liver MDSC (5×105). T cell proliferation was determined by measuring the number of hepatic CD8+Ova Tetramer+ T cells at 90 h. Addition of liver MDSC to DC.Ova immunizations decreased T cell proliferation in vivo (P<0.05). (D) In the same experiment, Ova Tetramer+ T cells were analyzed for expression of CD62L and CD44. Liver MDSC prevented full phenotypic activation. Experiments were repeated twice using two to three mice/immunization group.
To determine whether liver MDSC from mice with intra-abdominal cancer could induce CD4+ T cell differentiation into Tregs, MDSC from the livers of normal or tumor-bearing (i.p. MCA38) mice were cultured for 4 days with CD4+ T cells. Consistent with our previous results, hepatic MDSC isolated from mice harboring i.p. tumor increased expression of the CD4+CD25+ Foxp+ Treg phenotype (Fig. 8B).
Liver MDSC prevent T cell priming in vivo
To determine whether liver CD11b+Gr1+ cells from tumor-bearing hosts could suppress immunity in vivo, naïve C57BL/6 mice were adoptively transferred with OTI T cells and 24 h later, with DC.Ova or DC.Ova + liver CD11b+Gr1+ cells, harvested from tumor-bearing mice. At 90 h, the number of intra-hepatic Ova Tetramer+CD8+ T cells was measured using flow cytometry. Mice treated with DC.Ova had an almost threefold higher expansion of Ova Tetramer+CD8+ T cells compared with mice immunized with DC.Ova + MDSC (Fig. 8C). Furthermore, there was incomplete down-regulation of CD62L and up-regulation of CD44 on hepatic Ova Tetramer+CD8+ T cells in mice immunized with DC.Ova + MDSC compared with mice immunized with DC.Ova alone, suggesting that liver MDSC prevent activation of antigen-restricted T cells in vivo (Fig. 8D).
Liver CD11b+Gr1+ cells promote tumor growth in vivo and enable liver metastases
To determine whether liver MDSC inhibit anti-tumor responses in vivo, we tested them in several models. C57BL/6 mice were seeded s.c. with EG7 cells alone, EG7 + OTI T cells, or EG7 + OTI T cells + liver MDSC from mice with advanced i.p MCA38 tumors (Day 22). As expected, mice challenged with EG7 alone developed tumor between 7 and 14 days after inoculation, and coinjection of OTI T cells protected mice completely from tumor development (Fig. 9A). However, addition of liver CD11b+Gr1+ cells abrogated the tumor-protective effects of OTI T cells (Fig. 9A). These data suggest that liver MDSC from tumor-bearing mice inhibit anti-tumor immunity in vivo. Liver MDSC from mice with early i.p. MCA38 tumor (Day 10) also partially prevented OTI-mediated tumor protection in the same adoptive transfer model (Fig. 9B).
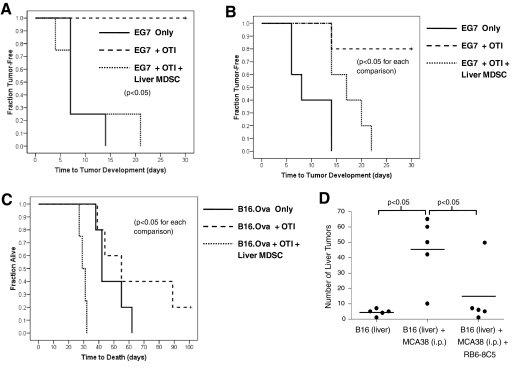
Liver MDSC inhibit anti-tumor immunity and enable growth of liver metastases. (A) Naïve C57BL/6 mice were challenged with EG7 (2×105) only, EG7 + OTI T cells (2×105), or EG7 + OTI T cells + liver CD11b+Gr1+ cells (5×105), which were harvested from mice with advanced (Day 21) i.p. MCA38 tumors (n=4–5/group). Time to tumor development is shown. (B) The same experimental scheme was repeated as in A, except that in the current experiment, liver CD11b+Gr1+ cells were derived from mice with early (Day 10) i.p. MCA38 tumors (n=5/group). (C) To determine whether liver MDSC can enable the growth of hepatic metastases, mice were challenged with a portal venous infusion of B16.Ova (2×104), B16.Ova + OTI T cells (5×105), or B16.Ova + OTI T cells + liver MDSC (6×105) harvested from mice with advanced i.p. MCA38 tumors (n=4–5/group). (D) To determine whether hepatic MDSC in tumor-bearing mice accelerate the growth of liver metastases in situ, mice were simultaneously challenged with intrasplenic B16 alone(8×104), intrasplenic B16 + i.p. MCA38 (5×105) or intrasplenic B16 + i.p. MCA38 + RB6-8C5 to deplete MDSC (thrice weekly doses of 150 μg/mouse). On Day 21, when i.p. tumor burdens were large, mice were killed, hepatectomy was performed, and the number of surface hepatic metastases was counted (n=5/group; horizontal lines represent average number of tumors for each group).
To determine whether liver MDSC promote the growth of hepatic metastases, naive mice were seeded via a portal venous injection of B16.Ova, B16.Ova + OTI T cells, or B16.Ova + OTI T cells + liver MDSC from mice with advanced i.p. tumor. As expected, mice receiving OTI T cells were partially protected from tumor development (Fig. 9C). However, addition of MDSC to immunization concentrates not only abrogated the protective effects of OTI but resulted in more rapid death from liver metastases than mice challenged with B16.Ova alone, suggesting hepatic CD11b+Gr1+ cells suppress endogenous immunity beyond simply countering the protective effects of adoptively transferred OTI T cells (Fig. 9C).
We next tested whether liver MDSC promote hepatic metastases in situ in tumor-bearing hosts with extra-hepatic maliganacies. Mice were challenged with infrasplenic B16 alone or challenged simultaneously with infrasplenic B16 and i.p. MCA38 and intrasplenic B16. After tumor challenge, splenectomy was performed to prevent intrasplenic tumor growth and partially eliminate the confounding effect of spleen MDSC. On Day 21, when i.p. tumor burdens were large, mice were killed and the number of liver metastases counted. Animals harboring i.p. tumor had a far higher average number of liver metastases (45 nodules) compared with animals challenged with B16 only (four nodules; Fig. 9D). To determine whether CD11b+Gr1+ cells were responsible for the enhanced growth of liver metastases in mice with i.p. tumor, Gr1+ cells were serially depleted in a group of mice challenged with i.p. MCA38 and intrasplenic B16. Remarkably, in depleted animals, the number of liver metastases decreased significantly (mean 15 nodules), suggesting that Gr1+ cells enabled mice with i.p. MCA38 tumors to grow liver metastases at an accelerated rate (Fig. 9D).
DISCUSSION
Until recently, the domain of MDSC was thought to be limited to primary and secondary lymphoid organs and among leukocytes associated with the tumor itself. Within the past year, Ilkovitch and Lopez [23] reported that adoptively transferred MDSC home to the liver and that native hepatic CD11b+Gr1+ cells disable Kupffer cells effectively in mice with advanced malignancy. Similarly, Li et al. [24] also reported recently that in mice with advanced malignancy, intra-hepatic CD11b+Gr1+ disable NK cell immunity. Our findings expand on these observations and extend our understanding of liver CD11b+Gr1+ immune-suppressive cells. In particular, we show that even in animals bearing preinvasive intra-abdominal tumors, CD11b+Gr1+ cells accumulate with the liver (Fig. 2). In LSL-KrasG12D mice with early PanIN lesions, CD11b+Gr1inter cells expand the liver but not the spleen as early as the 3rd month of life (Fig. 2). The fact that even early intra-abdominal carcinogenesis induces intra-hepatic immune suppression may explain partially why patients, who present with very early gastrointestinal cancers, such as localized pancreatic cancer, recur frequently with liver metastases. We show that expansion of liver CD11b+Gr1+ cells is contingent on KC (Fig. 4, A and B) and is limited to tumors growing within the peritoneal cavity, as s.c. tumors did not result in intra-hepatic expansion of the CD11b+Gr1+ population (Fig. 1C). We define the specific cellular subsets of intra-hepatic CD11b+Gr1+ cells based on cell granularity and morphology (Fig. 3, A and B), cellular surface phenotype (Supplemental Fig. 2), cytokine profile (Fig. 5E), and suppressive function (Fig. 6E). The basic monocytic and PMN-like subsets of liver CD11b+Gr1+ cells as well as their characterization as immature macrophages or DC are similar to those suggested previously for spleen MDSC [25, 26]. However, we show that the relative composition of these subsets in the liver (Fig. 3, C and D), their cellular phenotype, including expression of MHC I, MHC II, F4/80, CD31, and CD195 (Supplemental Fig. 3), and cytokine profile (Fig. 5, A–D and Supplemental Fig. 5) are considerably different from MDSC in other tissues. In fact, hepatic CD11b+Gr1+ cells produce robust levels of an array of proinflammatory and regulatory cytokines and chemokines, and spleen CD11b+Gr1+ cells are nonsecretory. The importance of each of these cytokines, individually or collectively, in the suppressive mechanism of liver MDSC requires further investigation.
Although the other recent studies have focused mostly on the capacity of liver MDSC to inhibit Kupffer cells and NK cells [23, 24], our work focused on T cell inhibition in vitro and in vivo. In particular, we show that CD11b+Gr1+ cells from the liver of tumor-bearing hosts inhibit T cell proliferation in response to various activation stimuli: CD3/CD28 ligation (Fig. 6, A and D–F), mitogen (Fig. 6B), and antigen (Fig. 6C) and antigen-pulsed DC (Fig. 8C). We also show that hepatic MDSC prevent activation of T cell surface phenotype in vitro (Fig. 8A) and in vivo (Fig. 8D) and induce the generation of Tregs (Fig. 8B). Furthermore, we show that liver CD11b+Gr1+ cells inhibit CTL-meditated cytolysis (Fig. 7). In consort with these findings, using adoptive transfer experiments, this is the first report to show that the CD11b+Gr1+ cells within the liver can enable hematogenous intra-hepatic metastases development by disabling antigen-restricted T cells (Fig. 9C). We also show using in situ models that liver CD11b+Gr1+ cells can accelerate the growth rate of liver metastases (Fig. 9D). By extension, these data suggest that liver CD11b+Gr1+ cells may have a contributory role in the development of liver metastases in patients with intra-abdominal cancer.
The reason for increased MDSC expansion specifically within the liver is uncertain. A plausible hypothesis is that MDSC expansion in the liver is related to the fact that venous drainage of tumor antigen from peritoneal organs passes directly through the liver via the portal venous system. Consistent with the notion that secreted factors are the impetus for recruitment, our data suggest that tumor production KC is required for liver MDSC expansion. However, the fact that simple injection of tumor-conditioned media, i.p. or directly into the portal venous circulation, did not increase hepatic CD11b+Gr1+ populations, suggests that the impetus for recruitment is more complex than secreted factors passing through the liver, and direct, unexplained tumor-related cellular factors are vital. The mechanism of inhibition of liver MDSC is also uncertain. We tested the inhibitory function of liver MDC in transgenic mice lacking expression of IL-6 or MCP-1, as high levels of these cytokines are associated with cancer progression in the liver [20, 21]. However, elimination of IL-6 or MCP-1 did not mitigate the inhibitory effects of liver MDSC on T cell proliferation. Similarly, blockade of IL-10, an immunosuppressive cytokine produced at markedly high levels by liver MDSC, did not abrogate their inhibitory activity. These experiments using regulatory cytokine and chemokine blockade, which failed to reverse MDSC inhibitory function, as well as the fact that tumor conditioned media were not inhibitory, suggest that direct cellular contact between MDSC and T cells is critical.
Furthermore, a difficult area in the field of tumor-induced immune suppression is the uncertain existence of a homologous counterpart in humans. That is, although the CD11b+Gr1+ phenotype is becoming a commonly accepted starting point for evaluating potentially suppressive myeloid-derived cells, there is no universal counterpart to this in human immunology [27]. Human MDSC have been purported to be Lin–HLA-DR–, CD14dim/–CD11b+CD33+ [28]. However, human MDSC vary in their expression of a number of other myeloid markers, including CD11c, CD13, CD15, CD31, and CD34 [29]. Rodriguez et al. [30] reported recently that a population of CD11b+CD66b+VEGFR+CD16dimCD62Ldim cells expands in the peripheral blood of renal cell carcinoma patients and may have a role in resistance to antiangiogenesis-targeted chemotherapy. Ochoa et al. [31] also showed that a large granular cell CD11b+CD15– population is found in the peripheral blood of renal cell carcinoma patients and expresses high levels of arginase I. However, establishing a definitive link between expansion of a particular population of myeloid-derived cells and tumor progression in humans still requires further study.
Even less is known about the possibility of immune suppression in the human liver in intra-abdominal cancer patients. A recent report characterizing human liver APC in hepatectomy specimens found that Lin–CD16–CD11c+CD141+ and Lin–CD16–CD11c+CD1c+ DC subsets are found in increased proportions in the liver relative to the peripheral blood, produce high levels of IL-10, and suppress T cell immunity in vitro. These APC populations generated CD4+CD25+Foxp3+ Tregs in vitro via IL-10-dependent mechanisms [32]. Goddard et al. [33] also found that human monocyte-derived CD11b+ APC in the human liver produce high IL-10 and low IFN-γ compared with skin APC and are poorly immunogenic. However, determination of the role of these cells and other myeloid-derived cells in the human liver in enabling hepatic metastases in cancer patients requires further investigation.
Despite the absence of a definitive corollary in human liver, our work in murine models proves the principal that gastrointestinal cancer, even at its earliest preinvasive stages, can induce tumor-enabling, immune-suppressive cells to expand in the liver at a distance from the actual site of tumor. These cells, which require KC for expansion, are distinct from spleen MDSC in terms of phenotype and secretory profile, potently suppress T cell proliferation and cytotoxicity in a variety of experimental contexts, and induce the development of Tregs. Our data showing that liver CD11b+Gr1+ cells promote liver tumor growth in adoptive transfer and in situ models suggest that translational studies aimed at finding correlative immune-suppressive cells in the human liver of cancer patients and investigating its association with the eventual development of liver metastases are warranted.
AUTHORSHIP
The authors contributed as follows: Michael Connolly—data collection, design, and data analysis; Jon Mallen-St. Clair—data collection, data analysis, and critical review; Andrea Bedrosian—data collection, data analysis, and critical review; Ashim Malhotra—data analysis and critical review; Valery Vera—data analysis and critical review; Junaid Ibrahim—data analysis and critical review; Justin Henning—data analysis and critical review; H. Leon Pachter—conception and critical review; Dafna Bar-Sagi—conception, design, and critical review; Alan B. Frey—conception, design, data analysis, and critical review; George Miller—conception, design, critical review, data collection, and manuscript preparation.
ACKNOWLEDGMENTS
This work was supported in-part by a Whitehead Fellowship Award for Junior Faculty in Biomedical and Biological Sciences (G. M.), a Liver Scholar Award from the American Liver Foundation (G. M.), and National Institutes of Health awards F30 AA 017344-01 (J. M-S. C.), CA55360-18 (D. B-S.), and CA108573 (A. F. B.).
Footnotes
Abbreviations: BM=bone marrow, CD62L=CD62 ligand, DC=dendritic cell(s), DC.Ova=DC (1×106) pulsed with Ova, Foxp3=forkhead box p3, IP-10=IFN-inducible protein 10, KC=keratinocyte-derived chemokine, Lin=lineage, LIX=LPS-induced chemokine, LSL=Lox-STOP-Lox, Mac-3= macrophage antigen 3, MDSC=myeloid-derived suppressor cell(s), MFI= mean fluorescence index, NPC=nonparenchymal cell(s), Ova= Ova257–264 peptide, PanIN lesions=preinvasive pancreatic cancer, PMN=polymorphonuclear neutrophil, Treg=T regulatory cell, VEGF=vascular endothelial growth factor, YFP=yellow fluorescent protein
The online version of this paper, found at www.jleukbio.org, includes supplemental information.
References
- Crispe I N, Giannandrea M, Klein I, John B, Sampson B, Wuensch S. Cellular and molecular mechanisms of liver tolerance. Immunol Rev. 2006;213:101–118. [Abstract] [Google Scholar]
- Solari M G, Thomson A W. Human dendritic cells and transplant outcome. Transplantation. 2008;85:1513–1522. [Europe PMC free article] [Abstract] [Google Scholar]
- Xia S, Guo Z, Xu X, Yi H, Wang Q, Cao X. Hepatic microenvironment programs hematopoietic progenitor differentiation into regulatory dendritic cells maintaining liver tolerance. Blood. 2008;112:3175–3185. [Europe PMC free article] [Abstract] [Google Scholar]
- Pillarisetty V G, Shah A B, Miller G, Bleier J I, DeMatteo R P. Liver dendritic cells are less immunogenic than spleen dendritic cells because of differences in subtype composition. J Immunol. 2004;172:1009–1017. [Abstract] [Google Scholar]
- Limmer A, Ohl J, Wingender G, Berg M, Jungerkes F, Schumak B, Djandji D, Scholz K, Klevenz A, Hegenbarth S, Momburg F, Hämmerling G J, Arnold B, Knolle P A. Cross-presentation of oral antigens by liver sinusoidal endothelial cells leads to CD8 T cell tolerance. Eur J Immunol. 2005;35:2970–2981. [Abstract] [Google Scholar]
- Goubier A, Dubois B, Gheit H, Joubert G, Villard-Truc F, Asselin-Paturel C, Trinchieri G, Kaiserlian D. Plasmacytoid dendritic cells mediate oral tolerance. Immunity. 2008;29:464–475. [Europe PMC free article] [Abstract] [Google Scholar]
- McMillan D C, McArdle C S. Epidemiology of colorectal liver metastases. Surg Oncol. 2007;16:3–5. [Abstract] [Google Scholar]
- Rosenblum J D, Boyle C M, Schwartz L B. The mesenteric circulation. Anatomy and physiology. Surg Clin North Am. 1997;77:289–306. [Abstract] [Google Scholar]
- Bail J P, Foultier M T, Patrice T. Experimental model of liver metastases: adhesion and growth of cells on contact with endothelial or hepatocyte cell monolayer cultures. Res Exp Med (Berl) 1994;194:53–61. [Abstract] [Google Scholar]
- Gervaz P, Scholl B, Mainguene C, Poitry S, Gillet M, Wexner S. Angiogenesis of liver metastases: role of sinusoidal endothelial cells. Dis Colon Rectum. 2000;43:980–986. [Abstract] [Google Scholar]
- Clark C E, Hingorani S R, Mick R, Combs C, Tuveson D A, Vonderheide R H. Dynamics of the immune reaction to pancreatic cancer from inception to invasion. Cancer Res. 2007;67:9518–9527. [Abstract] [Google Scholar]
- Hingorani S R, Petricoin E F, Maitra A, Rajapakse V, King C, Jacobetz M A, Ross S, Conrads T P, Veenstra T D, Hitt B A, Kawaguchi Y, Johann D, Liotta L A, Crawford H C, Putt M E, Jacks T, Wright C V, Hruban R H, Lowy A M, Tuveson D A. Preinvasive and invasive ductal pancreatic cancer and its early detection in the mouse. Cancer Cell. 2003;4:437–450. [Abstract] [Google Scholar]
- Pillarisetty V G, Miller G, Shah A B, DeMatteo R P. GM-CSF expands dendritic cells and their progenitors in mouse liver. Hepatology. 2003;37:641–652. [Abstract] [Google Scholar]
- Monu N, Frey A B. Suppression of proximal T cell receptor signaling and lytic function in CD8+ tumor-infiltrating T cells. Cancer Res. 2007;67:11447–11454. [Europe PMC free article] [Abstract] [Google Scholar]
- Miller G, Lahrs S, Pillarisetty V G, Shah A B, DeMatteo R P. Adenovirus infection enhances dendritic cell immunostimulatory properties and induces natural killer and T-cell-mediated tumor protection. Cancer Res. 2002;62:5260–5266. [Abstract] [Google Scholar]
- Miller G, Pillarisetty V G, Shah A B, Lahrs S, Xing Z, DeMatteo R P. Endogenous granulocyte-macrophage colony-stimulating factor overexpression in vivo results in the long-term recruitment of a distinct dendritic cell population with enhanced immunostimulatory function. J Immunol. 2002;169:2875–2885. [Abstract] [Google Scholar]
- Miller G, Lahrs S, DeMatteo R P. Overexpression of interleukin-12 enables dendritic cells to activate NK cells and confer systemic antitumor immunity. FASEB J. 2003;17:728–730. [Abstract] [Google Scholar]
- Hara H, Suda K. Review of the cytologic features of noninvasive ductal carcinomas of the pancreas: differences from invasive ductal carcinoma. Am J Clin Pathol. 2008;129:115–129. [Abstract] [Google Scholar]
- Chintakuntlawar A V, Chodosh J. Chemokine CXCL1/KC and its receptor CXCR2 are responsible for neutrophil chemotaxis in adenoviral keratitis. J Interferon Cytokine Res. 2009;29:657–666. [Europe PMC free article] [Abstract] [Google Scholar]
- Naugler W E, Sakurai T, Kim S, Maeda S, Kim K, Elsharkawy A M, Karin M. Gender disparity in liver cancer due to sex differences in MyD88-dependent IL-6 production. Science. 2007;317:121–124. [Abstract] [Google Scholar]
- Yoshidome H, Kohno H, Shida T, Kimura F, Shimizu H, Ohtsuka M, Nakatani Y, Miyazaki M. Significance of monocyte chemoattractant protein-1 in angiogenesis and survival in colorectal liver metastases. Int J Oncol. 2009;34:923–930. [Abstract] [Google Scholar]
- Dhodapkar M V, Steinman R M, Krasovsky J, Munz C, Bhardwaj N. Antigen-specific inhibition of effector T cell function in humans after injection of immature dendritic cells. J Exp Med. 2001;193:233–238. [Europe PMC free article] [Abstract] [Google Scholar]
- Ilkovitch D, Lopez D M. The liver is a site for tumor-induced myeloid-derived suppressor cell accumulation and immunosuppression. Cancer Res. 2009;69:5514–5521. [Europe PMC free article] [Abstract] [Google Scholar]
- Li H, Han Y, Guo Q, Zhang M, Cao X. Cancer-expanded myeloid-derived suppressor cells induce anergy of NK cells through membrane-bound TGF-β 1. J Immunol. 2009;182:240–249. [Abstract] [Google Scholar]
- Movahedi K, Guilliams M, Van den Bossche J, Van den Bergh R, Gysemans C, Beschin A, De B P, Van Ginderachter J A. Identification of discrete tumor-induced myeloid-derived suppressor cell subpopulations with distinct T cell-suppressive activity. Blood. 2008;111:4233–4244. [Abstract] [Google Scholar]
- Youn J I, Nagaraj S, Collazo M, Gabrilovich D I. Subsets of myeloid-derived suppressor cells in tumor-bearing mice. J Immunol. 2008;181:5791–5802. [Europe PMC free article] [Abstract] [Google Scholar]
- Nagaraj S, Gabrilovich D I. Tumor escape mechanism governed by myeloid-derived suppressor cells. Cancer Res. 2008;68:2561–2563. [Abstract] [Google Scholar]
- Talmadge J E. Pathways mediating the expansion and immunosuppressive activity of myeloid-derived suppressor cells and their relevance to cancer therapy. Clin Cancer Res. 2007;13:5243–5248. [Abstract] [Google Scholar]
- Almand B, Clark J I, Nikitina E, van Beynen J, English N R, Knight S C, Carbone D P, Gabrilovich D I. Increased production of immature myeloid cells in cancer patients: a mechanism of immunosuppression in cancer. J Immunol. 2001;166:678–689. [Abstract] [Google Scholar]
- Rodriguez P C, Ernstoff M S, Hernandez C, Atkins M, Zabaleta J, Sierra R, Ochoa A C. Arginase I-producing myeloid-derived suppressor cells in renal cell carcinoma are a subpopulation of activated granulocytes. Cancer Res. 2009;69:1553–1560. [Europe PMC free article] [Abstract] [Google Scholar]
- Ochoa A C, Zea A H, Hernandez C, Rodriguez P C. Arginase, prostaglandins, and myeloid-derived suppressor cells in renal cell carcinoma. Clin Cancer Res. 2007;13:721s–726s. [Abstract] [Google Scholar]
- Bamboat Z M, Stableford J A, Plitas G, Burt B M, Nguyen H M, Welles A P, Gonen M, Young J W, DeMatteo R P. Human liver dendritic cells promote T cell hyporesponsiveness. J Immunol. 2009;182:1901–1911. [Europe PMC free article] [Abstract] [Google Scholar]
- Goddard S, Youster J, Morgan E, Adams D H. Interleukin-10 secretion differentiates dendritic cells from human liver and skin. Am J Pathol. 2004;164:511–519. [Europe PMC free article] [Abstract] [Google Scholar]
Articles from Journal of Leukocyte Biology are provided here courtesy of The Society for Leukocyte Biology
Full text links
Read article at publisher's site: https://doi.org/10.1189/jlb.0909607
Read article for free, from open access legal sources, via Unpaywall:
https://jlb.onlinelibrary.wiley.com/doi/pdfdirect/10.1189/jlb.0909607
Free after 12 months at www.jleukbio.org
http://www.jleukbio.org/cgi/content/full/87/4/713
Free to read at www.jleukbio.org
http://www.jleukbio.org/cgi/content/abstract/87/4/713
Citations & impact
Impact metrics
Citations of article over time
Smart citations by scite.ai
Explore citation contexts and check if this article has been
supported or disputed.
https://scite.ai/reports/10.1189/jlb.0909607
Article citations
Multi-parametric atlas of the pre-metastatic liver for prediction of metastatic outcome in early-stage pancreatic cancer.
Nat Med, 30(8):2170-2180, 28 Jun 2024
Cited by: 5 articles | PMID: 38942992 | PMCID: PMC11416063
Role of T cells in liver metastasis.
Cell Death Dis, 15(5):341, 16 May 2024
Cited by: 1 article | PMID: 38755133 | PMCID: PMC11099083
Review Free full text in Europe PMC
Key players of immunosuppression in epithelial malignancies: Tumor-infiltrating myeloid cells and γδ T cells.
Cancer Rep (Hoboken), 7(5):e2066, 01 May 2024
Cited by: 0 articles | PMID: 38703051 | PMCID: PMC11069128
Review Free full text in Europe PMC
Molecular mechanisms of pancreatic cancer liver metastasis: the role of PAK2.
Front Immunol, 15:1347683, 26 Jan 2024
Cited by: 8 articles | PMID: 38343537 | PMCID: PMC10853442
The Brain Pre-Metastatic Niche: Biological and Technical Advancements.
Int J Mol Sci, 24(12):10055, 13 Jun 2023
Cited by: 3 articles | PMID: 37373202 | PMCID: PMC10298140
Review Free full text in Europe PMC
Go to all (71) article citations
Data
Data behind the article
This data has been text mined from the article, or deposited into data resources.
BioStudies: supplemental material and supporting data
Similar Articles
To arrive at the top five similar articles we use a word-weighted algorithm to compare words from the Title and Abstract of each citation.
Modulating the expression of IFN regulatory factor 8 alters the protumorigenic behavior of CD11b+Gr-1+ myeloid cells.
J Immunol, 183(1):117-128, 01 Jul 2009
Cited by: 31 articles | PMID: 19542426 | PMCID: PMC2744444
Recruitment of a myeloid cell subset (CD11b/Gr1 mid) via CCL2/CCR2 promotes the development of colorectal cancer liver metastasis.
Hepatology, 57(2):829-839, 08 Jan 2013
Cited by: 150 articles | PMID: 23081697
Premetastatic soil and prevention of breast cancer brain metastasis.
Neuro Oncol, 15(7):891-903, 17 Apr 2013
Cited by: 53 articles | PMID: 23595625 | PMCID: PMC3688013
Lymphocyte-mediated Immune Regulation in Health and Disease: The Treg and γδ T Cell Co-conspiracy.
Immunol Invest, 45(8):767-775, 12 Sep 2016
Cited by: 9 articles | PMID: 27617588 | PMCID: PMC5167356
Review Free full text in Europe PMC
Funding
Funders who supported this work.
Junior Faculty in Biomedical and Biological Sciences
Liver Scholar Award from the American Liver Foundation
NCI NIH HHS (2)
Grant ID: CA108573
Grant ID: CA55360-18
NIAAA NIH HHS (1)
Grant ID: F30 AA 017344-01
NIDDK NIH HHS (2)
Grant ID: K08 DK085278
Grant ID: K08 DK085278-01
NIGMS NIH HHS (1)
Grant ID: T32 GM008444
National Institutes of Health (3)
Grant ID: F30 AA 017344-01
Grant ID: CA55360-18
Grant ID: CA108573