Abstract
Free full text

Unique multipotent cells in adult human mesenchymal cell populations
Associated Data
Abstract
We found adult human stem cells that can generate, from a single cell, cells with the characteristics of the three germ layers. The cells are stress-tolerant and can be isolated from cultured skin fibroblasts or bone marrow stromal cells, or directly from bone marrow aspirates. These cells can self-renew; form characteristic cell clusters in suspension culture that express a set of genes associated with pluripotency; and can differentiate into endodermal, ectodermal, and mesodermal cells both in vitro and in vivo. When transplanted into immunodeficient mice by local or i.v. injection, the cells integrated into damaged skin, muscle, or liver and differentiated into cytokeratin 14-, dystrophin-, or albumin-positive cells in the respective tissues. Furthermore, they can be efficiently isolated as SSEA-3(+) cells. Unlike authentic ES cells, their proliferation activity is not very high and they do not form teratomas in immunodeficient mouse testes. Thus, nontumorigenic stem cells with the ability to generate the multiple cell types of the three germ layers can be obtained through easily accessible adult human mesenchymal cells without introducing exogenous genes. These unique cells will be beneficial for cell-based therapy and biomedical research.
Recent advances in stem cell research have revealed the existence of various types of tissue stem cells that contribute to the functional maintenance of organs and to cell renewal, tissue remodeling, and repair (1, 2). These stem cells are expected to contribute to regenerative medicine, but this will require elucidation of their stem cell properties to control their proliferation and differentiation. Among the many kinds of tissue stem cells, hematopoietic stem cells and neural stem cells have been characterized most extensively (i.e., their ability to self-renew and differentiate into tissue-specific cell types has been clearly demonstrated at the single-cell level) (3, 4). In contrast, some of the properties of mesenchymal stem cells remain obscure. For example, one mesenchymal cell type, the bone marrow stromal cell (MSC), differentiates into cells of the same mesenchymal lineage, such as osteocytes, cartilage, and adipocytes, but also differentiates into cells of other lineages, such as neuronal cells and liver cells, suggesting that their differentiation is not tissue-specific, and that they are thus qualified as multipotent cells (5–8). In most cases, however, the differentiation was demonstrated in a heterogeneous population comprising MSCs and not at the single-cell level. Therefore, it remains under debate whether different subsets of cells are responsible for differentiation into cell types of different lineages, such as osteocytes and neuronal cells, or whether a distinctly multipotent stem cell type exists that is responsible for differentiation across all the oligo-lineage boundaries. Furthermore, hair follicle stem cells and dermal stem cells of the skin differentiate into cells positive for neuronal and smooth muscle cell markers, but their differentiation into cells of all three germ layers has not been demonstrated at the single-cell level (9–11).
In the present study, we demonstrate, at the single-cell level, that adult human skin fibroblasts, MSCs, and native bone marrow aspirates contain a distinct type of stem cell that is capable of generating cells with characteristics of all three germ layers. These cells are indistinguishable from other major mesenchymal cells in adherent culture, but when they are transferred to suspension culture, they form characteristic cell clusters that are positive for pluripotency markers and exhibit self-renewal and differentiation. Furthermore, they can be efficiently isolated as cells positive for both SSEA-3, a human pluripotency marker, and CD105, a mesenchymal cell marker. The cells exhibit multipotency, but their proliferation activity is not very high. Furthermore, although retaining their differentiation ability in vivo, these cells, unlike authentic ES cells, do not form teratomas in testes of immunodeficient mice. Our findings thus suggest that adult human mesenchymal cell populations, such as skin fibroblasts and MSCs, contain distinctly multipotent stem cells and that further studies of these cells will promote a better understanding of mesenchymal stem cell properties. Collection and enrichment of these cells should contribute to improved differentiation efficiency in mesenchymal cell populations. Finally, because these cells are easily accessible, they will be a realistic source of adult human multipotent stem cells that are capable of differentiation into cells with characteristics of all three germ layers without the need to introduce exogenous genes. These cells thus hold great promise for cell-based therapy and biomedical research.
Results
Analysis of Cell Clusters Generated from Human Mesenchymal Cells.
We found that naive human MSCs (H-MSCs) grown in adherent culture spontaneously formed characteristic cell clusters at a very low frequency that appeared similar to clusters formed by human ES cells at an early stage (Fig. 1A) (12), suggesting that naive H-MSCs might contain multipotent cells. At a certain size, these cell clusters stopped growing and had a heterogeneous appearance (Fig.1B).

Characterization of M-clusters. (A and B) Characteristic cell clusters that occur spontaneously in adherent cultures of naive H-MSCs. (C and D) MC culture of H-fibroblasts on day 7 showing an M-cluster (C, arrow). Immunocytochemical localization of Nanog (E and F), Oct3/4 (G), Sox2 (H), PAR4 (I), and SSEA-3 (J) in M-clusters formed by H-fibroblasts (E, I, and J) and H-MSCs (F, G, and H). ALP(+) human ES cells (K), M-cluster (H-fibroblast) (L), and naive H-fibroblasts (M). (N) Schematic diagram of the self-renewal of Muse cells. (Scale bars: A–C, 100 μm; D–M, 50 μm.)
Dormant tissue stem cells are activated when tissues are exposed to stress, burdens, or damage (13–16). We therefore explored the possibility of whether stress conditions could be exploited for a method to enrich the putative stem cells in adult human mesenchymal cell populations. We subjected two strains of human skin fibroblasts (H-fibroblasts) and four strains of H-MSCs to six different stress conditions, including long-term trypsin incubation (LTT) for 8 or 16 hr (Table S1 and SI Materials and Methods).
Stem cells are often grown in suspension culture, which is an efficient and convenient method to maintain their stem cell properties (17, 18). H-fibroblasts or H-MSCs that survived the stress treatments were therefore suspended in methylcellulose (MC) medium (19) at a density of 8,000 cells per milliliter (MC culture; SI Materials and Methods) and grown for 7 days (Fig. 1C). Each condition gave rise to cell clusters with sizes of up to 50–150 μm in diameter (Fig. 1D). Using different filters, we separated the cell clusters according to their size and characterized them by immunocytochemistry. Most of the clusters with a diameter larger than 25 μm contained cells positive for the pluripotency markers Nanog, Oct3/4, SSEA-3, PAR-4, and Sox2 and were positive for alkaline phosphatase (ALP) staining (Fig. S1). We therefore only counted cell clusters larger than 25 μm. Among the stress conditions tested, 16-hr LTT was most potent in the formation of cell clusters in H-fibroblasts, and 8-hr LTT was most potent in the formation of cell clusters in H-MSCs (Table S1 and SI Results). As expected, the formed clusters contained cells positive for the above pluripotency markers (Fig. 1 E–J) and ALP staining (Fig. 1 K–M). We called these cells multilineage differentiating stress enduring (Muse) cells because they express pluripotency markers; as described below, they differentiate into ectodermal, endodermal, and mesodermal cells; and they endure through stress conditions. We refer to H-fibroblasts and H-MSCs treated with 16-hr and 8-hr LTT, respectively, as “Muse-enriched cell populations” (MEC populations).
To calculate the frequency of Muse-cell-derived cell cluster (M-cluster; SI Results) formation accurately, MEC populations derived from both H-fibroblasts and H-MSCs were subjected to single-cell suspension culture after limiting dilution (Fig. S2 and SI Materials and Methods), showing that 11.6 ± 1.6% of the cells in the H-fibroblast–MEC population and 8.1 ± 0.2% of the cells in the H-MSC–MEC population proceeded to form M-clusters after 7 days. Naive populations (without LTT) were also examined and showed that 1.3 ± 0.1% (H-fibroblasts) and 1.1 ± 0.1% (H-MSCs) of the cells formed M-clusters in single-cell suspension culture after limiting dilution.
Self-Renewal and Expansion of Muse Cells.
After LTT, Muse cells began to divide after 1–2 days in MC culture and continued to divide at a rate of ≈1.3 days per cell division until day 8, forming cell clusters. Cell proliferation gradually slowed by days 11–12 and ceased around day 14, with cell clusters reaching a maximum size of 150 μm (Fig. S3 and SI Materials and Methods).
When M-clusters formed in single-cell suspension culture after limiting dilution (day 12) were dissociated into single cells by a 5-min trypsin treatment and returned to single-cell suspension culture, the cells survived but divided very slowly (5–7 days per cell division) or sometimes not at all (Fig. 1N1). However, transfer of single M-clusters to adherent culture reinitiated cell proliferation and produced expanded cells. When cultures that had expanded to ≈3,000–5,000 cells were dissociated and subjected to single-cell suspension culture without LTT, 48.0 ± 5.8% (H-fibroblasts) and 40.3 ± 9.1% (H-MSCs) of the cells formed M-clusters (Fig. 1N2). When cultures were allowed to expand to 5–10 × 104 cells and were then subjected to LTT to produce MEC populations (Fig. 1N3), 12.3 ± 1.3% (H-fibroblasts) and 8.5 ± 0.5% (H-MSCs) of these cells formed second generation M-clusters. We repeated this culture cycle, consisting of LTT → suspension culture → adherent culture, five times, and every cell generation showed similar behavior and a similar frequency of M-cluster formation. We also confirmed that the fifth generation M-clusters were still positive for pluripotency markers and ALP staining. Furthermore, karyotypes of cells expanded from M-clusters did not show detectable abnormalities (Fig. S4 and SI Results). In conclusion, the proliferation activity of Muse cells is not very high, and proliferation stops in suspension culture when the cell clusters reach a defined size. Nevertheless, the proliferation of Muse cells can be reinitiated by transfer to adherent culture, which is followed by the formation of next-generation M-clusters, demonstrating the capacity of Muse cells for self-renewal and proliferation.
Differentiation of M-Clusters.
To analyze their differentiation ability, single M-clusters formed in single-cell suspension culture after limiting dilution were transferred onto gelatin-coated dishes. After 7 days of culture, immunocytochemistry revealed cells positive for neurofilament-M [an ectodermal marker; the ratio of positive cells was 3.5 ± 0.5% (H-fibroblasts) and 3.7 ± 0.6% (H-MSCs)], α-smooth muscle actin [α-SMA; mesodermal, 12.2 ± 1.8% (H-fibroblasts) and 8.0 ± 0.6% (H-MSCs)], α-fetoprotein [endodermal, 2.7 ± 0.1% (H-fibroblasts) and 3.2 ± 0.3% (H-MSCs)], cytokeratin 7 [endodermal, 5.5 ± 0.1% (H-fibroblasts) and 3.4 ± 0.6% (H-MSCs)], or desmin [mesodermal, 14.2 ± 0.4% (H-fibroblasts) and 10.1 ± 0.5% (H-MSCs)] (Fig. 2 A–E). RT-PCR of cells derived from first- and third-generation M-clusters confirmed that these cells expressed α-fetoprotein (endodermal) and GATA6 (endodermal), microtubule-associated protein-2 (MAP-2; ectodermal), and Nkx2.5 (mesodermal), whereas these markers were not clearly detected in naive fibroblasts and MSC populations (Fig. 2F).
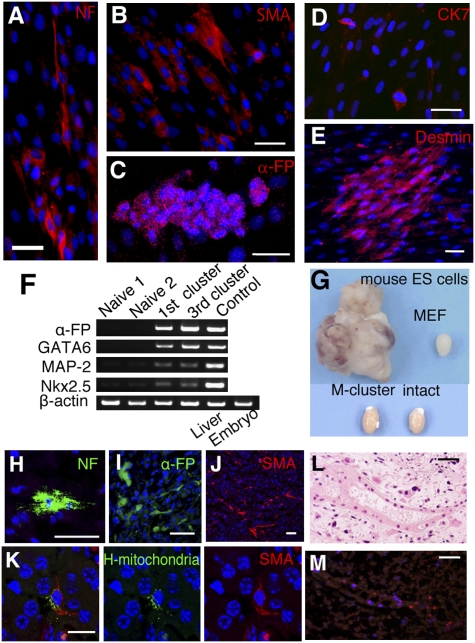
Differentiation of Muse cells in vitro and in testes. Immunocytochemistry of neurofilament-M (NF) (A), α-SMA (B), α-fetoprotein (α-FP) (C), cytokeratin 7 (CK7) (D), and desmin (E) in cells derived from a single M-cluster (H-fibroblasts). (F) RT-PCR analysis of naive cells and first- and third-generation M-clusters (first and third clusters) derived from H-fibroblasts. Positive controls were human fetus liver (Liver) for α-FP and whole human embryo (Embryo) for GATA6, MAP-2, and Nkx2.5. (G–M) Testes of immunodeficient mice injected with cells. (G) Uninjected testes (intact) and testes injected with mouse ES cells (8 weeks), mouse embryonic fibroblast (MEF) cells (8 weeks), and M-clusters (6 months). Immunohistochemistry of NF (H), α-FP (I), and SMA (J) in testes injected with MEC populations and M-clusters. (K) Double-labeling of human mitochondria (green) and SMA (red). The tube-like structure (L) was positive for human mitochondria in the adjacent section (M; red). (Scale bars: A–E and H–L, 50 μm; M, 20 μm.)
We injected MEC populations or M-clusters into the testes of immunodeficient mice to test whether they form teratomas. None of the testes injected with MEC populations or M-clusters formed teratomas for up to 6 months, and most of the testes were not significantly larger than control testes (Fig. 2G and SI Results). In the MEC- or M-cluster-injected testes, cells positive for human mitochondria and for ectodermal (neurofilament), endodermal (α-fetoprotein), and mesodermal (SMA) lineage markers were detected (Fig. 2 H–M and Fig. S5).
We next tested the differentiation of MEC populations in vivo by transplanting the cells into damaged tissues of the back skin (by local injection of GFP-labeled H-MSC–MEC population), gastrocnemius muscle (i.v. injection of GFP-H-fibroblast–MEC population), or liver (i.v. injection of GFP-H-fibroblast–MEC population) of immunodeficient mice. In regenerating skin, after 2 weeks, 79.5 ± 2.0% of the transplanted cells in the epidermis also expressed cytokeratin 14 (Fig. 3A). GFP(+) cells were also incorporated into regenerating muscle. After 2 weeks, the nuclei of these cells were centrally located, but after 4 weeks, 96.1 ± 2.2% of the GFP(+) cells had the appearance of mature myofibers with peripheral nuclei and expressed human dystrophin (Fig. 3 B and C). Some of the transplanted cells expressed satellite cell marker Pax7 (Fig. 3B). In the regenerating liver, after 4 weeks, most of the cells positive for human Golgi complex expressed human albumin (84.9 ± 5.3%) and human antitrypsin (87.6 ± 3.0%; Fig. 3 D and E). These data suggest that MEC populations can differentiate into ectodermal, endodermal, and mesodermal lineage cells in vivo.
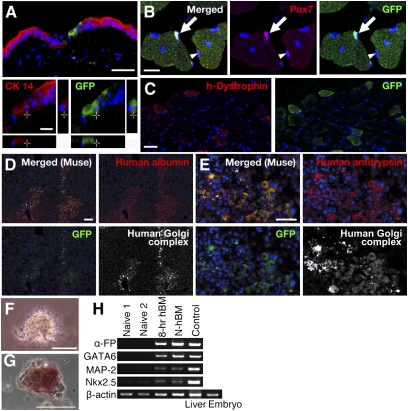
Transplantation of Muse cells and M-cluster formation from bone marrow. (A–E) Differentiation of GFP-labeled MEC population (H-fibroblasts) in damaged tissues of immunodeficient mice. (A) Cells locally injected into the edge of the excised region. Transplanted GFP(+) cells expressed cytokeratin 14 (red) in the regenerating epidermis (2 weeks). (B) Two weeks after i.v. injection, GFP(+) cells with central nuclei were seen in cardiotoxin-injected cutaneous muscle. Transplanted GFP(+) cells (arrow) and host cells [GFP(−), arrowhead] that expressed Pax7 were seen. (C) After 4 weeks, the GFP(+) muscle fibers expressed human dystrophin (h-Dystrophin; red). Four weeks after i.v. injection, most of the transplanted GFP(+) cells in liver with CCl4-induced damage were positive for human Golgi complex (D and E, white); some of them expressed human albumin (D, red) or human antitrypsin (E, red). (F–H) Formation of M-clusters from bone marrow-derived mononucleated cells. (F) M-clusters formed with 8-hr LTT (8-hr hBM-MC, day 7). (G) ALP(+) cells in 8-hr hBM-MC (day 7). (H) RT-PCR of naive H-MSCs (Naive 1 and Naive 2); M-clusters formed with 8-hr LTT (8-hr hBM) or without LTT [Naive hBM (N-hBM)]. Positive controls were human fetus liver (Liver) for α-fetoprotein (α-FP) and whole human embryo (Embryo) for GATA6, MAP-2, and Nkx2.5. (Scale bars: A, B, E, F, and G, 50 μm; C and D, 100 μm.)
M-Cluster Formation Directly from Native Bone Marrow Aspirate.
The experiments described so far were performed with Muse cells and M-clusters derived from cell cultures, which may have acquired characteristics that differ from those of cells in situ. Mesenchymal cells are known to reside in the mononucleated cell fraction of bone marrow, which can be collected directly from native tissue without culturing. We therefore tested whether cells directly collected from human bone marrow (hBM) would also be able to form M-clusters. Isolated mononucleated cells were either subjected directly to MC culture (naive hBM-MC) or to 8-hr LTT before MC culture (8-hr hBM-MC). After 7 days, 8-hr hBM-MC formed M-clusters at a frequency of 0.3 ± 0.04%, ≈60–75 times higher than that of naive hBM-MC (0.004 ± 0.001%) (Fig. 3F). M-clusters from both naive hBM-MC and 8-hr hBM-MC were ALP(+) (Fig. 3G), and RT-PCR of cells expanded from single M-clusters on gelatin-coated dishes showed expression of α-fetoprotein, GATA6, MAP-2, and Nkx2.5 (Fig. 3H). These results suggest that M-clusters can be formed directly from hBM and that they can be enriched by 8-hr LTT.
Naive hBM-MC formed M-clusters at an extremely low frequency. Because culturing can change the composition of cell populations, cells in stable culture may have a different propensity to form M-clusters than cells from native tissues. To test this possibility, we grew hBM aspirate in adherent culture to collect primary MSCs and subjected the cells directly to MC culture without 8-hr LTT. This protocol resulted in a much higher frequency of M-cluster formation of 0.3 ± 0.08%. When primary MSCs were further cultured to the second and fifth passages, the frequency of M-cluster formation without LTT increased up to 0.5 ± 0.04% and 0.9 ± 0.1%, respectively. Consistent with this finding, 1.3 ± 0.1% of naive H-fibroblasts and 1.1 ± 0.1% of naive H-MSCs formed M-clusters in single-cell suspension culture as described above. These results suggest that Muse cells have a high stress tolerance and can endure in vitro culture and the subculture procedures.
Bone marrow contains many cell types, including MSCs, hematopoietic lineage cells, and endothelial cells (19). To determine which fraction contains the Muse cells, we isolated mononucleated cells from hBM aspirate and subjected them to magnetic affinity cell sorting (MACS) using antibodies against CD34 and CD117 [markers for hematopoietic cells (20)] and CD105 [marker for MSCs (5, 7)]. We then subjected the cells to 8-hr LTT and allowed them to grow in MC culture for 7 days. The CD34+/117+/105− cell fraction produced few M-clusters, but the CD34−/117−/105+ fraction produced 50 times more M-clusters than the CD34−/117−/105− fraction (SI Results). This result suggests that the majority of Muse cells belong to the CD105(+) mesenchymal cell population.
Characteristic Features of Muse Cells.
FACS analysis revealed that among tested surface markers, MEC populations showed a substantially increased number of cells that were SSEA-3(+) [a human pluripotency marker (12)] compared with naive populations (Fig. 4A and SI Results). The percentage of SSEA-3(+) cells detected by FACS [1.1 ± 0.05% (H-MSCs) and 1.8 ± 0.22% (H-fibroblasts); Fig. 4A] and immunocytochemistry [0.7 ± 0.1% (H-fibroblasts); Fig. 4B] in naive cells approached the previously determined frequency of M-cluster formation in single-cell suspension culture (≈1%), as described above. For MEC populations, the percentage of SSEA-3(+) cells detected by FACS [11.6 ± 0.15% (H-MSCs) and 8.6 ± 0.0.32% (H-fibroblasts); Fig. 4A] was also comparable to the frequency of M-cluster formation in single-cell suspension culture [11.6 ± 1.6% (H-fibroblasts) and 8.1 ± 0.2% (H-MSCs)] as stated. Furthermore, the expression of Oct3/4 and Sox2 in SSEA-3(+) cells was demonstrated in cultured H-fibroblasts using immunocytochemistry (Fig. 4 D and E). We therefore used FACS to separate SSEA-3(+) and (−) cells from MEC populations of both H-fibroblasts and H-MSCs and subjected each fraction to single-cell suspension culture after limiting dilution. The result showed that 56.5 ± 3.2% (H-MSCs) and 60.0 ± 4.5% (H-fibroblasts) of the SSEA-3(+) cells generated M-clusters, whereas only a few M-clusters developed in the SSEA-3(−) fraction.
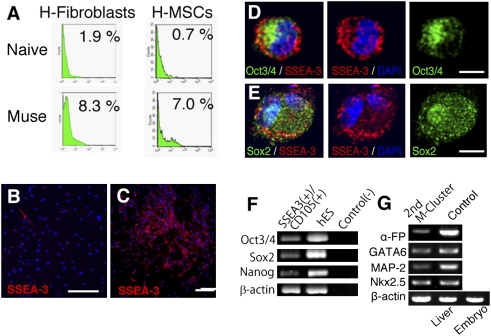
Characterization of Muse cells. (A) FACS analysis for SSEA-3 expression in naive cells (Naive) and MEC populations (Muse) derived from H-fibroblasts and H-MSCs. SSEA-3(+) cells (red) in a naive population (B) and in cells expanded from a single M-cluster derived from a FACS-sorted SSEA-3(+) cell (C), both from H-fibroblasts. Immunocytochemistry of Oct3/4 (D, green, Sox2 (E, green), and SSEA-3 (D and E, red) in Muse cells derived from H-fibroblasts. (F) RT-PCR of Oct3/4, Sox2, and Nanog in directly isolated SSEA-3+/CD105+ cells from bone marrow, human ES cells for a positive control, and the template without reverse transcription for a negative control [Control(−)]. (G) RT-PCR of second-generation M-clusters (2nd M-cluster) from bone marrow-derived mononucleated cells. Positive controls were human fetus liver (Liver) for α-fetoprotein (α-FP) and whole human embryo (Embryo) for GATA6, MAP-2, and Nkx2.5. (Scale bars: B and C, 100 μm; D, 10 μm; E–G, 5 μm.)
The importance of SSEA-3(+) cells was also seen in the transplantation experiments. In contrast to the integration and differentiation of MEC populations in damaged skin, muscle, and liver (Fig. 3 A–E), transplantation of SSEA-3(−) populations resulted in a significantly smaller number of integrated cells and fewer cells that were positive for the tissue markers (Fig. S6 and SI Results).
Of note, at the scale of 3,000–5,000 cells, only ≈45.0 ± 3.2% (H-fibroblasts) of the cells that expanded from a single FACS-sorted SSEA-3(+) cell were SSEA-3(+) (Fig. 4C). This result suggests that proliferation of Muse cells may give rise to Muse cells and non-Muse cells.
The possibility remains that Muse cells are artificially induced by LTT. As described above, the majority of Muse cells exist in the bone marrow's CD105(+) cell fraction. Furthermore, SSEA-3(+) cells showed Muse cell properties. We therefore attempted to collect Muse cells directly from adult hBM aspirates by isolating them as SSEA-3/CD105 double-positive cells. Double-positive cells, which constituted 0.04 ± 0.008% of bone marrow-derived mononucleated cells, were directly subjected to RT-PCR, which showed the expression of Nanog, Oct3/4, and Sox2 in these cells (Fig. 4F). Isolated SSEA-3+/CD105+ cells were further subjected to single-cell suspension culture after limiting dilution without LTT. At 7 days, 11.4 ± 1.2% of the cells (corresponding to 0.003–0.005% of the mononucleated cells) formed M-clusters that were ALP(+). Single M-clusters were then again expanded in adherent culture to 3,000 cells and subsequently subjected to single-cell suspension culture. Of these cells, 33.5 ± 3.1% formed second-generation M-clusters, and RT-PCR of the cells that expanded from a single M-cluster on gelatin-coated dishes indicated that the cells expressed α-fetoprotein, GATA6, MAP-2, and Nkx2.5 (Fig. 4G), suggesting that cells with properties consistent with those of Muse cells reside in adult hBM.
Discussion
We isolated a specific type of human mesenchymal stem cell (i.e., Muse cell) that is capable of generating cells with the characteristics of all three germ layers from a single cell. Muse cells are (i) stress tolerant; (ii) indistinguishable from general mesenchymal cells in adhesion culture; (iii) able to form M-clusters in suspension culture that are positive for pluripotency markers and ALP staining; (iv) able to self-renew; (v) not very high in their proliferation activity and not shown to form teratomas in immunodeficient mouse testes; (vi) able to differentiate into endodermal, ectodermal, and mesodermal cells both in vitro and in vivo; and (vii) efficiently isolated from naive tissues as cells positive for both CD105 and SSEA-3.
To investigate whether Muse cells exist in native tissues or whether LTT induces cells to acquire properties of multipotent stem cells, we isolated SSEA-3/CD105 double-positive cells directly from hBM aspirates and subjected them to single-cell suspension culture without LTT. The formation of M-clusters showed that Muse cells or potential Muse cells already exist in adult native hBM. LTT is thus not necessary for collecting Muse cells but is a method to enrich them.
The properties of several kinds of stem cells present in mesenchymal tissue, such as neural crest-derived stem cells (NCSCs) that exist both in the bone marrow and skin, MSCs, skin-derived precursors (SKPs), perivascular cells, and adipose-derived stem cells, were recently analyzed and described (11, 21–24). Of note, SKPs can be clonally expanded and serve as dermal stem cells for use in skin homeostasis and repair (11). NCSCs can also be clonally expanded, and they may contribute to nerve repair (22). Although, these stem cells can differentiate into ectodermal (e.g., neural marker-positive cells) and mesodermal (e.g., SMA-positive cells, osteocytes, chondrocytes) lineage cells (11, 21–24), their differentiation into representatives of all three germ layers has not been reported. Muse cells are unique among mesenchymal stem cells in that they are able to differentiate not only into ectodermal and mesodermal cells but into endodermal cells. That is, a single Muse cell can generate cells representative of each of the three germ layers. Considering that multilineage differentiation of MSCs, such as into neuronal cells, muscle cells, and liver cells, has been reported (5–8), it is possible that Muse cells contribute to such multilineage differentiation of MSCs.
Muse cells may have practical advantages for regenerative medicine, such as their easy accessibility and differentiation potential. They are not tumorigenic but retain differentiation ability in vivo after transplantation. In fact, they expressed endodermal, ectodermal, and mesodermal lineage markers after injection into mouse testes; integrated into damaged skin, muscle, and liver tissue; and differentiated into cells expressing the respective tissue markers cytokeratin 14 (ectodermal), dystrophin (mesodermal), and albumin (endodermal). Moreover, the multipotency of Muse cells does not need to be induced by the introduction of exogenous genes, and they can be isolated from skin and bone marrow, which are accessible from an individual or from a cell bank. Typically, each tissue contains a very small number of stem cells, and like other stem cells, the proportion of Muse cells in bone marrow-derived mononucleated cells is very small. However, large numbers of Muse cells can be obtained from mesenchymal cell populations by simply cycling the cells through a series of culturing steps, namely, Muse-cell selection followed by formation of M-clusters in suspension culture and expansion of the cells in adherent culture.
Muse cells did not show characteristics of tumorigenic proliferation, and, consistently, they did not develop into teratomas in mice testes. It has recently been reported that epiblast stem cells cultured under certain conditions also did not form teratomas in testes, even though they showed pluripotency in vitro (25). This finding suggests that even pluripotent cells do not always show the formation of teratomas in testes. Furthermore, if Muse cells are normally maintained in adult human tissues, such as in skin fibroblasts, their proliferation must be strictly regulated; otherwise, they would easily form tumors in virtually every part of the body.
Questions regarding the origin, location, and physiological roles of Muse cells in fibroblasts and MSCs will require further study.
Materials and Methods
Culture Cells.
Two strains of human skin fibroblasts and four strains of H-MSCs were maintained at 37 °C in α-minimum essential medium (α-MEM) containing 10% (vol/vol) FBS and 0.1 mg/mL kanamycin. Mononucleated cells were collected from six hBM aspirates using Lymphoprep Tubes (Axis-Shield PoC AS). For selection of FBS, an H-MSC clone was plated onto a 24-well dish at a density of 1.5 × 104 cells per cm2. Serum lots (ES cell grade; HyClone) were checked by adding a sample from each lot to a well at a concentration of 10% (vol/vol) in α-MEM and cultured for 1–2 weeks. The serum in the well that showed the highest frequency of spontaneous cell cluster formation, as shown in Fig. 1 A and B, was chosen for further experiments.
Generation of MEC Populations and M-Clusters.
MEC populations were produced by treating cells with LTT (16 hr for H-fibroblasts and 8 hr for H-MSCs), followed by vortexing at 1,800–2,200 rpm for 3 min (MS1 Minishaker, IKA Works, Staufen, Germany) and centrifugation at 740 × g for 15 min. To produce M-clusters, individual cells were cultured in MC or in single-cell suspension culture. For MC culture, culture dishes were first coated with polyHEMA (P3932; Sigma) to avoid attachment of cells to the bottom of the dish. MC (MethoCult H4100; StemCell Technologies) was diluted in 20% (vol/vol) FBS in α-MEM to a final concentration of 0.9%. The cell concentration in the semisolid MC medium was adjusted to be 8 × 103 cells per milliliter. Cells and MC were mixed thoroughly by gentle pipetting, and the mixture was transferred to a polyHEMA-coated dish. At this concentration, the cell-to-cell distance was sufficiently large to minimize cell aggregation. For single-cell suspension culture, MEC populations were subjected to a limiting dilution with 10% (vol/vol) FBS in α-MEM and single cells were plated into each well coated with polyHEMA. The frequency of M-cluster formation was calculated from three experiments for each strain, with a minimum of 250 wells per experiment.
Detailed protocols for cell culture, stress conditions, ALP staining, immunocytochemistry, immunohistochemistry, transplantation experiments, RT-PCR, karyotyping, MACS sorting, and FACS analysis are provided in SI Text.
Acknowledgments
We thank Dr. Thomas Walz (Harvard Medical School) for proofreading the manuscript and Dr. Hiroshi Hamada (Osaka University, Japan) for providing antibodies. We thank the late Keiji Takita, Director General of the Japan New Energy and Industrial Technology Development Organization, who passed away during this study. This work was supported by the Japan New Energy and Industrial Technology Development Organization.
Footnotes
The authors declare no conflict of interest.
*This Direct Submission article had a prearranged editor.
This article contains supporting information online at www.pnas.org/lookup/suppl/10.1073/pnas.0911647107/-/DCSupplemental.
References
Articles from Proceedings of the National Academy of Sciences of the United States of America are provided here courtesy of National Academy of Sciences
Full text links
Read article at publisher's site: https://doi.org/10.1073/pnas.0911647107
Read article for free, from open access legal sources, via Unpaywall:
https://europepmc.org/articles/pmc2889306?pdf=render
Free after 6 months at www.pnas.org
http://www.pnas.org/cgi/content/full/107/19/8639
Free after 6 months at www.pnas.org
http://www.pnas.org/cgi/reprint/107/19/8639.pdf
Free after 6 months at www.pnas.org
http://www.pnas.org/cgi/content/abstract/107/19/8639
Citations & impact
Impact metrics
Citations of article over time
Alternative metrics
Smart citations by scite.ai
Explore citation contexts and check if this article has been
supported or disputed.
https://scite.ai/reports/10.1073/pnas.0911647107
Article citations
Intravenous administration of muse cells improves cerebral ischemia outcome via immunomodulation in the spleen.
J Cereb Blood Flow Metab, 271678X241290363, 13 Oct 2024
Cited by: 0 articles | PMID: 39397400 | PMCID: PMC11563515
Mesenchymal stem cells from adipose tissue prone to lose their stemness associated markers in obesity related stress conditions.
Sci Rep, 14(1):19702, 24 Aug 2024
Cited by: 0 articles | PMID: 39181924 | PMCID: PMC11344827
Structural reconstruction of mouse acute aortic dissection by intravenously administered human Muse cells without immunosuppression.
Commun Med (Lond), 4(1):174, 09 Sep 2024
Cited by: 0 articles | PMID: 39251746 | PMCID: PMC11384757
High-throughput fluorescence lifetime imaging flow cytometry.
Nat Commun, 15(1):7376, 04 Sep 2024
Cited by: 0 articles | PMID: 39231964 | PMCID: PMC11375057
Sequencing-based study of neural induction of human dental pulp stem cells.
Hum Cell, 37(6):1638-1648, 29 Aug 2024
Cited by: 0 articles | PMID: 39210197
Go to all (293) article citations
Other citations
Data
Data behind the article
This data has been text mined from the article, or deposited into data resources.
BioStudies: supplemental material and supporting data
Similar Articles
To arrive at the top five similar articles we use a word-weighted algorithm to compare words from the Title and Abstract of each citation.
Human adipose tissue possesses a unique population of pluripotent stem cells with nontumorigenic and low telomerase activities: potential implications in regenerative medicine.
Stem Cells Dev, 23(7):717-728, 17 Jan 2014
Cited by: 96 articles | PMID: 24256547
CD271 antigen defines a subset of multipotent stromal cells with immunosuppressive and lymphohematopoietic engraftment-promoting properties.
Haematologica, 95(4):651-659, 23 Feb 2010
Cited by: 115 articles | PMID: 20179086 | PMCID: PMC2857196
In utero transplantation of human bone marrow-derived multipotent mesenchymal stem cells in mice.
J Orthop Res, 24(3):301-312, 01 Mar 2006
Cited by: 19 articles | PMID: 16482576
Stem cell plasticity in the hematopoietic system.
Int J Hematol, 79(1):7-14, 01 Jan 2004
Cited by: 15 articles | PMID: 14979472
Review