Abstract
Free full text

5A Apolipoprotein Mimetic Peptide Promotes Cholesterol Efflux and Reduces Atherosclerosis in Mice
Abstract
Intravenous administration of apolipoprotein (apo) A-I complexed with phospholipid has been shown to rapidly reduce plaque size in both animal models and humans. Short synthetic amphipathic peptides can mimic the antiatherogenic properties of apoA-I and have been proposed as alternative therapeutic agents. In this study, we investigated the atheroprotective effect of the 5A peptide, a bihelical amphipathic peptide that specifically effluxes cholesterol from cells by ATP-binding cassette transporter 1 (ABCA1). 5A stimulated a 3.5-fold increase in ABCA1-mediated efflux from cells and an additional 2.5-fold increase after complexing it with phospholipid (1:7 mol/mol). 5A-palmitoyl oleoyl phosphatidyl choline (POPC), but not free 5A, was also found to promote cholesterol efflux by ABCG1. When incubated with human serum, 5A-POPC bound primarily to high-density lipoprotein (HDL) but also to low-density lipoprotein (LDL) and promoted the transfer of cholesterol from LDL to HDL. Twenty-four hours after intravenous injection of 5A-POPC (30 mg/kg) into apoE-knockout (KO) mice, both the cholesterol (181%) and phospholipid (219%) content of HDL significantly increased. By an in vivo cholesterol isotope dilution study and monitoring of the flux of cholesterol from radiolabeled macrophages to stool, 5A-POPC treatment was observed to increase reverse cholesterol transport. In three separate studies, 5A when complexed with various phospholipids reduced aortic plaque surface area by 29 to 53% (n = 8 per group; p < 0.02) in apoE-KO mice. No signs of toxicity from the treatment were observed during these studies. In summary, 5A promotes cholesterol efflux both in vitro and in vivo and reduces atherosclerosis in apoE-KO mice, indicating that it may be a useful alternative to apoA-I for HDL therapy.
High-density lipoprotein (HDL) has consistently been shown to be inversely related to cardiovascular disease risk (Remaley et al., 2008). HDL promotes the flux of excess cholesterol from peripheral cells to the liver by the reverse cholesterol transport (RCT) pathway (Duffy and Rader, 2006). The initial step, involves the interaction of apoA-I, the main protein component on HDL, with the ABCA1s in cells, which triggers the removal of cholesterol and phospholipid from cells and the formation of nascent HDL (Remaley et al., 2001). Additional cholesterol can also be effluxed from cells by lipid-rich forms of HDL by ABCG1 and possibly by other mechanisms (Matsuura et al., 2006).
In addition to promoting RCT, HDL has recently been shown to have numerous other antiatherogenic properties (Remaley et al., 2008), such as the ability to act as an antioxidant, suppress inflammation and thrombosis, promote vasodilatation, and maintain endothelial cell integrity. The in vivo role, however, of these other properties of HDL and even the physiologic relevance of RCT has not been definitively established.
Based on the inverse relationship between HDL and the incidence of cardiovascular disease, there has been great interest in developing drugs that raise HDL (Garcia, 2008). Except for niacin there has been limited success in finding small-molecule drugs that increase HDL and decrease cardiovascular disease (Barter, 2009). This has prompted the development of a new treatment strategy called HDL therapy, which involves the intravenous infusion of exogenous HDL to rapidly stabilize patients with acute coronary syndrome (Sethi et al., 2007; Remaley et al., 2008). Even a single infusion of HDL has been shown in animal studies to reduce plaque size, lipid content, and inflammation (Barter, 2009). Two different clinical trials (Nissen et al., 2003; Tardif et al., 2007) have shown that five treatments with either a recombinant form of a mutant form of apoA-I called apoA-IMilano or normal apoA-I purified from human plasma and reconstituted with phospholipid can reduce plaque size to a similar degree achieved with several years of statin therapy (Nanjee et al., 1996; Nissen, 2005).
Short synthetic peptide mimics of apoA-I have also been shown to reduce atherosclerosis in animal models (Bielicki et al., 2010). They are being investigated as possible therapeutic agents, because of the cost and difficulty in producing sufficient quantities of apoA-I (Sethi et al., 2007; Remaley et al., 2008). The most thoroughly investigated apoA-I mimetic peptide is the single helical peptide D-4F and its L-stereoisomer, L-4F, which has been tested in one human clinical trial (Bloedon et al., 2008). Although D-4F can promote cholesterol efflux from cells (Navab et al., 2004), bihelical peptides have been shown in vivo to be superior for promoting cholesterol efflux (Wool et al., 2009). The main antiatherogenic function of D-4F and L-4F has been proposed to be related to their ability to sequester oxidized lipids (Van Lenten et al., 2008).
In this study, we investigated the effect of 5A peptide treatment on lipid and lipoprotein metabolism in mice and the development of atherosclerosis. The 5A peptide is a bihelical peptide linked with by a proline residue (Sethi et al., 2008). The first amphipathic helix has relatively high lipid affinity, whereas the lipid affinity of the second helix was reduced by substituting five nonpolar amino acids on the hydrophobic face of the helix with alanine, hence its name. This arrangement of helices was found to reduce its cytotoxicity and increase its specificity for removing cholesterol from cells by ABCA1 (Sethi et al., 2008). Intravenous administration of the 5A peptide complexed with phospholipid was found in apoE-KO mice to raise HDL, promote RCT, and reduce atherosclerosis, indicating that 5A shares many of the same beneficial antiatherogenic features of apoA-I and may be a suitable substitute for apoA-I in HDL therapy.
Materials and Methods
Peptide Synthesis and Lipid Reconstitution.
5A (DWLKA FYDKVAEKLKEAF-P-DWAKAAYDKAAEKAKEAA) was synthesized by a solid-phase procedure, using an fluorenylmethyloxycarbonyl protocol on a Biosearch 9600 peptide synthesizer and was purified to more than 99% purity by reverse-phase high-performance liquid chromatography on an Aquapore RP-300 column (PerkinElmer Life and Analytical Sciences, Waltham, MA) (Sethi et al., 2008). For experiments with biotinylated 5A, a C6-biotin group was added during the synthesis to the N terminus, and biotin-5A was used at a 1:10 M ratio to unlabeled 5A. 5A was reconstituted with lipid at a 1:7 M ratio with palmitoyl oleoyl phosphatidyl choline (POPC) or sphingomyelin and dipalmitoyl phosphatidylcholine (DPPC) (5A-SM/DPPC;1:3.5:3.5 M ratio), as described previously (Sethi et al., 2008) and delivered intravenously into the retro-orbital sinus or by intraperitoneal injection as indicated. Unless otherwise indicated, the dose of 5A-POPC is based on the protein mass of 5A peptide. POPC liposomes were prepared by sonication (Jonas, 1986).
Mice and Diets.
Female apoE-KO (The Jackson Laboratory, Bar Harbor, ME) were fed a regular rodent chow diet (NIH-07 chow diet: 0.025% cholesterol, 4.5% fat; Ziegler Brothers, Inc., Gardner, PA). All animal studies were approved by the Animal Care and Use Committee from each institution (protocols H-0050R1 and H-0018R1, National Heart, Lung, and Blood Institute).
Isolation and Analysis of Lipoproteins.
HDL (d = 1.063–1.21 g/ml) and LDL (d = 1.019–1.063 g/ml) were isolated from human plasma by sequential ultracentrifugation, followed by dialysis at 4°C against phosphate-buffered saline, containing 0.01% (wt/vol) EDTA. Lipoproteins were analyzed on a microfluidic-based electrophoresis system, using the P200 protein chip (Agilent Technologies, Santa Clara, CA) on the 2100 Bioanalyzer (Agilent Technologies). Chromatograms from the electrophoresis were used to construct a digital gel image. Human apoA-I was measured by enzyme-linked immunosorbent assay (Wako Bioproducts, Richmond, VA). A dot blot assay was used to detect biotin-labeled 5A-POPC peptide, by applying samples to a nitrocellulose membrane (Whatman Schleicher and Schuell, Keene, NH), using a vacuum manifold device (Millipore Corporation, Billerica, MA) and then incubating the membrane with a streptavidin-horseradish peroxidase conjugate (Thermo Fisher Scientific, Waltham, MA) for 1 h and HRP Color Development Reagent (Bio-Rad Laboratories, Hercules, CA). For digital quantification, scanned images were analyzed by ImageQuant version 5.2 (GE Healthcare, Little Chalfont, Buckinghamshire, UK).
Analysis of Plasma Lipids and Lipoproteins.
Plasma lipoproteins and lipids were measured enzymatically (Wako Bioproducts) on a ChemWell 2910 analyzer (Awareness Technology, Inc., Palm City, FL). Lipoproteins were analyzed by fast-protein liquid chromatography (FPLC), using two Superose 6 HR 10/30 columns (Amar et al., 1998).
In Vitro Cholesterol Efflux Assay.
Cholesterol efflux studies were performed as described previously (Remaley et al., 2003), with the following modifications. BHK-mock and BHK stably transfected cells with human ABCA1 cDNA or ABCG1 cDNA (Vaughan and Oram, 2003; Sankaranarayanan et al., 2009) were labeled for 18 h with 1 μCi/ml of 3H-cholesterol in minimum essential medium α medium plus 10% fetal calf serum. Transporters were induced with 10 nM mifepristone in Dulbecco's modified Eagle's medium plus 0.2 mg/ml of fatty acid-free bovine serum albumin for 18 h. Cholesterol flux was measured after the addition of media containing an acceptor plus 10 nM mifepristone in minimum essential medium α medium with 0.2% fatty acid-free bovine serum albumin. After 6 h, media were collected and filtered (24 well, 25-μm pore size; Whatman Schleicher and Schuell), and cells were lysed in 0.4 ml of 0.1% SDS and 0.1 N NaOH. Radioactive counts in media and cell fractions were measured by liquid scintillation counting, and results are expressed as percentage of total counts effluxed per the indicated time interval.
In Vivo Cholesterol Efflux Assay.
Cholesterol efflux in vivo was measured as described previously (Mukhamedova et al., 2008). In brief, RAW 264.7 macrophage cells were simultaneously radiolabeled and loaded with cholesterol by incubation for 48 h with 29.7 mCi/ml [3H]cholesterol and acetylated LDL (50 μg/ml). Cells were washed, incubated for 24 h in serum-free medium, and resuspended in 0.15 M sterile saline at a concentration of 107 cells/ml. Cells were injected intraperitoneally into male C57BL/6 mice (2 × 106 cells containing 5 × 106 dpm per mouse) (n = 7). Mice were pretreated twice with 5A-POPC (30 mg/kg) 8 h before and within 1 h before cell injection. After 24 h, mice were euthanized, and blood, liver, and feces were collected. Aliquots of plasma were counted, cholesterol from liver and feces was extracted (Folch et al., 1957), and cholesterol was separated by thin-layer chromatography and counted.
Cholesterol Isotope Dilution RCT Assay.
RCT was measured in male Sprague-Dawley rats, using a cholesterol isotope dilution assay. Rats (250–300 g) were purchased with in-dwelling jugular vein cannula (Charles River Breeding Laboratories, Portage, MI). A single bolus of 5A-POPC (20 mg/ml) was administered intravenously at 1 ml/min, and 30 min after peptide administration a constant intravenous infusion (0.1 ml/h) of [2,3-13C2]cholesterol (13C2-C) (Cambridge Isotope Laboratories, Andover, MA) was administered for 5 h. The infusate was prepared by a modification of the method described by Ostlund and Matthews (1993)). In brief, 200 mg of 13C2-cholesterol was dissolved into 13 ml of warm USP ethanol and mixed slowly into 200 ml of 10% Intralipid (Fresenius-Kabi, Uppsala, Sweden) solution to a final concentration of 1.0 mg/ml 13C2-C. Food was removed, and animals were transferred to a clean cage at 7:00 AM right before starting the infusion. Fifty to 100 μl of heparinized whole blood was collected at 0.5, 2.5, 4, 5.5, 9.5, 25, and 31 h from the tail, samples were spun, and plasma was separated immediately. After the infusion, animals were individually housed in metabolic cages, and total fecal collections were made for the next 5 days.
Plasma-free cholesterol was extracted with ethanol-acetone, acetylated with toluene/pyridine/acetyl chloride, and dissolved in toluene for GC/MS analysis (Agilent 6890 gas chromatograph; J & W Scientific, Folsom, CA). Mass spectrometry was done by electron impact. Selective ion monitoring was used with m/z 368-370 of the M0/M1/M2 mass isotopomers of the molecular ion. The 13C2-cholesterol enrichment in M2 (EM2) were calculated by subtracting the percentage of M2 [M2/(M0 + M1 + M2)] of an unlabeled standard from the percentage of M2 of the sample.
Stool samples were homogenized with water, and neutral sterols (NS) and bile acids (BA) were extracted separately under basic and acidic conditions, respectively, in the presence of the internal standards 5α-cholestane and 5β-cholanic acids. The BA extract was split into two fractions. The first, used for compositional analysis by a flame ionization detector, was directly subjected to a two-step derivatization: butylation with butanolic HCl followed by silylation by N,o-bis(trimethylsilyl) trifluoroacetamide-pyridine. The second half was further purified for mass spectrometric analysis with an octadecyl solid-phase extraction cartridge, selectively eluting primarily deoxycholic acid with a 20% aqueous-methanol solution before butylation and silylation. The NS fraction was silylated directly for both compositional and isotope analysis. 13C2-C isotopic enrichments were measured, using GC/combustion-isotope ratio/MS (Thermo Finnegan MAT 253 IR-MS, Bremen, Germany). Enrichments were determined as atom percent excess by comparison of the unknown samples to a standard curve generated with gravitametrically prepared working lab standards with known enrichments. Molar percent excess is calculated as 14.5 or 15 × atom percent excess for the acetyl or silyl derivative of C, respectively. Compositional analysis and excretion measurement of BA and NS was performed by GC/flame ionization detection by comparison to the internal standards.
Analysis of Aortic Lesions.
The left ventricle of the heart was perfused with phosphate-buffered saline, followed by a fixative solution (4% paraformaldehyde, 5% sucrose, 20 mM EDTA, pH 7.4). The aorta was dissected from its origin in the heart to the ileal bifurcation and was placed in fixative solution for 18 h, stained with Sudan intravenous solution for 25 min, then destained for 25 min in 80% ethanol, and finally washed in water. After removal of any remaining adventitial fat, aortas were cut longitudinally, placed on glass slides embedded with glycerin, covered by a microscope cover glass, and sealed with nail polish. Quantification of aortic plaques from the ileal bifurcation to its origin, excluding branching vessels, was performed in a blinded fashion in triplicate, using the Image-Pro Plus version 4.1 software (Media Cybernetics, Inc., Bethesda, MD). Data are reported as percentage of total aortic surface covered by lesions.
Results
In Vitro Effect of 5A-POPC on Cholesterol Efflux.
The effect of phospholipid reconstitution of the 5A peptide on cholesterol efflux was examined in BHK cells stably transfected with ABCA1 (Fig. 1, A and B) or ABCG1 (Fig. 1, C and D). As described previously (Sethi et al., 2008), the free 5A peptide promoted cholesterol efflux by ABCA1 (Fig. 1A), but it was ineffective in promoting cholesterol efflux by ABCG1 (Fig. 1C). Reconstitution of 5A with POPC increased cholesterol efflux from ABCA1-transfected cells by approximately 3-fold (Fig. 1B), but cholesterol efflux from the control cell line increased nearly to the same degree, indicating that most of the increase was independent of ABCA1. After subtracting the non-ABCA1 component of cholesterol efflux detected from the control cell line, Vmax for cholesterol efflux by ABCA1 (12 ± 1%/18 h) was found not to significantly change after reconstitution of 5A with phospholipid, but it did lower the Km for cholesterol efflux from 6.17 ± 0.98 to 1.22 ± 0.51 μM. In contrast to free 5A peptide, 5A-POPC was also capable of promoting cholesterol efflux from ABCG1-transfected cells (Fig. 1D), but again after subtraction of the cholesterol efflux results from the control cell line only approximately 20 ± 1.5% of the increase in cholesterol efflux after phospholipid reconstitution was caused by ABCG1.
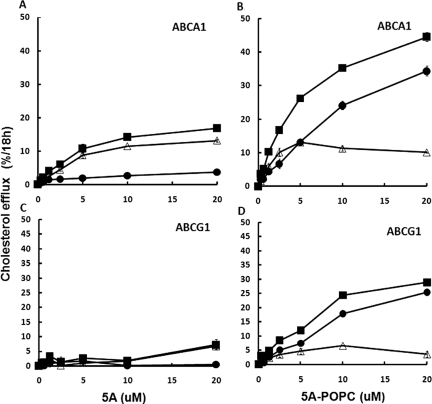
Effect of 5A on cholesterol efflux. Free 5A (A and C) or 5A-POPC (B and D) at the indicated doses were used to efflux cholesterol from ABCA1-transfected (A and B) or ABCG1-transfected (C and D) BHK cells. Contribution of cholesterol efflux from the transporter () was calculated after subtraction of cholesterol efflux from the transfected cell line (■) from the mock-transfected control cell line (●). Results are expressed as the mean ± 1 S.D. of triplicates.
Ex Vivo Effect of 5A-POPC on Plasma Lipoproteins.
Reconstitution of 5A with POPC resulted in only a single peak containing both peptide and phospholipid, which migrated on FPLC slightly before HDL (Fig. 2, A and B). When biotin-labeled 5A was reconstituted with POPC, incubated with human plasma at 37°C for 45 min, and separated by FPLC, approximately 65% of 5A was found bound to HDL, with the remaining bound to LDL and a small amount to very low-density lipoprotein (Fig. 2C). Incubation of 5A-POPC with plasma did not significantly shift the elution position of human apoA-I to either lower or higher molecular weight fractions (Fig. 2D). Treatment of plasma with 5A-POPC was also associated with a considerable increase in the size of both HDL and LDL, as observed by FPLC (Fig. 2, E and F). The 5A-POPC treatment increased 11-fold the phospholipid content of HDL (Fig. 2E), whereas the cholesterol content of HDL increased by approximately 26% and the cholesterol content of LDL decreased by approximately 18% (Fig. 2F).
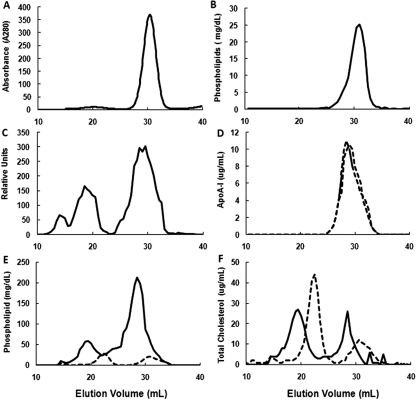
Effect of ex vivo incubation of 5A-POPC on plasma lipoproteins after separation by FPLC. A, protein absorbance FPLC profile of 5A reconstituted with POPC (1:7 M ratio). B, phospholipid FPLC profile of 5A reconstituted with POPC (1:7 M ratio). C, FPLC profile of biotinylated 5A-POPC (5 μg /ml final concentration) incubated with plasma for 45 min at 37°C and separated by FPLC. D, apoA-I FPLC profile of plasma before (dashed line) and after (solid line) incubation with 5A-POPC (5 μg /ml final concentration) for 45 min at 37°C. E, phospholipid FPLC profile of plasma before (dashed line) and after (solid line) incubation with 5A-POPC (5 μg/ml final concentration) for 45 min at 37°C. F, total cholesterol FPLC profile of plasma before (dashed line) and after (solid line) incubation with 5A-POPC (5 μg /ml final concentration) for 45 min at 37°C.
The ex vivo effect of 5A-POPC treatment on isolated HDL and LDL and plasma lipoproteins was also examined after separation by electrophoresis and staining with a dye sensitive to phospholipids (Fig. 3). 5A-POPC was found to migrate in a pre-β position (Fig. 3, lane 1) before the two major HDL subfractions observed in the α position for isolated human HDL (Fig. 3, lane 2). When 5A-POPC was incubated with HDL, it seemed to form a major band in the α position, and some slower migrating bands in the pre-β region (Fig. 3, lane 3). When 5A-POPC was incubated with isolated LDL (Fig. 3, lane 5), it seemed to fuse with LDL, leaving only a faint residual band in the pre-β region. Finally, when 5A-POPC was added to serum (Fig. 3, lane 6), it increased the intensity of the band in the LDL region and slowed its migration, but the primary change was a marked increase in the intensity of a lipoprotein band in the same region as isolated 5A-POPC and an increase in the intensity of a band in the pre-β position.
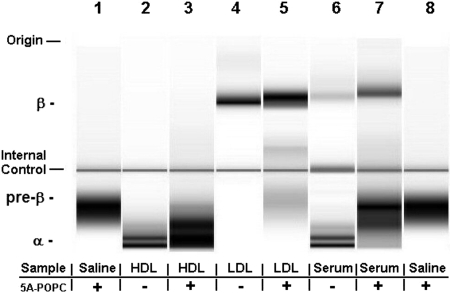
Effect of ex vivo incubation of 5A-POPC on plasma lipoproteins after separation by electrophoresis. 5A-POPC (5 μg/ml final dose) was incubated for 45 min at 37°C with either HDL or LDL isolated by density gradient ultracentrifugation or with human serum and then separated by electrophoresis on the 2100 Bioanalyzer. Lanes 1 and 8, 5A-POPC; lane 2, HDL; lane 3, HDL plus 5A-POPC; lane 4, LDL; lane 5, LDL plus 5A-POPC; lane 6, human serum; lane 7, human serum plus 5A-POPC. Horizontal lines indicate location of internal control.
In Vivo Effect of 5A-POPC on Plasma Lipoproteins.
5A-POPC (30 mg/kg) was injected intravenously into apoE-KO mice, and plasma lipoproteins were monitored over time by FPLC (Fig. 4). 5A-POPC was found by 3 h to decrease LDL-cholesterol by approximately 17% and increased HDL-cholesterol by 29% (Fig. 4, A and B). By 24 h, LDL-cholesterol returned to baseline but HDL-cholesterol further increased to 149% above baseline. Cholesteryl ester was found by 3 h to increase in LDL by approximately 13% and at 24 h to decrease by 42%, whereas for HDL it increased by 75 and 181%, at 3 and 24 h, respectively (Fig. 4, C and D). The major change, however, in HDL composition was in phospholipids (Fig. 4, E and F). At 3 h phospholipid content of HDL increased by 72% and at 24 h by 219%.
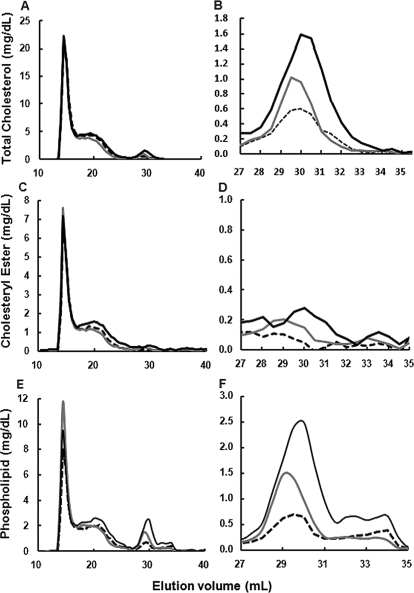
Effect of in vivo treatment of 5A-POC on plasma lipid and lipoproteins. A, C, and E, apoE-KO mice (n = 5) were injected intravenously with 5A-POPC (30 mg/kg), and pooled plasma collected at baseline (dashed lines), 3 h after injection (gray solid lines), and 24 h after injection (black solid lines) was separated by FPLC and analyzed for total cholesterol (A), cholesteryl esters (C), and phospholipids (E). B, D, and F, enlargements of the HDL peak from A, C, and E, respectively.
In Vivo Effect of 5A-POPC on RCT.
The in vivo effect of 5A-POPC on RCT was examined with three different experimental approaches. In the first study, after an intravenous bolus of 5A-POPC (30 mg/kg), plasma was removed at various times and tested for its ability to promote cholesterol efflux from ABCA1- and ABCG1-transfected BHK cells (Fig. 5). Treatment with 5A-POPC was found to increase cholesterol efflux by approximately 15 ± 5% at 6 h and 20 ± 8% by 24 h. 5A-POPC treatment had no significant effect on cholesterol efflux by ABCG1 at 6 h but increased ABCG1 cholesterol efflux by approximately 47 ± 13% at 24 h. No increase in nonspecific cholesterol efflux was observed from the mock-transfected control cell line after treatment with 5A-POPC.
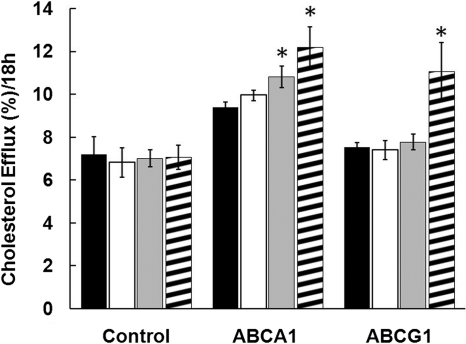
Effect of in vivo treatment of 5A-POC on cholesterol efflux by plasma. apoE-KO mice (n = 6) were injected intravenously with 5A-POPC (30 mg/kg), and pooled plasma collected at baseline (black bars) and 1 h (white bars), 6 h (gray bars), and 24 h (striped bars) after injection were tested for cholesterol efflux from control mock-transfected cells, ABCA1-transfected cells, or ABCG1-transfected cells. Results represent the mean ± 1 S.D. of quadruplicates. , p < 0.05 compared with baseline result.
The effect of 5A-POPC on RCT from peripheral tissues was also tested in rats by a cholesterol isotope dilution study (Fig. 6), which monitors the efflux of unlabeled cholesterol from peripheral tissues, which results in the dilution of the plasma tracer 13C-cholesterol that is continuously intravenously infused. Treatment with 5A-POPC (40 mg/Kg) increased the efflux of cholesterol into the plasma from peripheral tissues by approximately 20 ± 4% at the low dose (40 mg/kg) and 65 ± 7% at the high dose (100 mg/kg) (Fig. 6A). No significant change was observed in fecal excretion of neutral sterols or bile acids after 5A-POPC treatment (Fig. 6B).
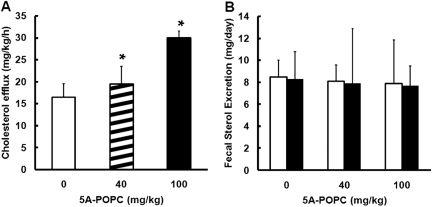
Effect of 5A-POPC treatment on in vivo cholesterol efflux from total tissues. A, cholesterol efflux from tissues in rats (n = 4) injected intravenously with the indicated dose of 5A-POPC was determined from isotope dilution curves. B, fecal sterol excretion was determined for neutral sterols (open bars) and bile acids (solid bars) for rats treated with the indicated dose of 5A-POPC. Results represent the mean ± 1 S.D. of triplicates. , p < 0.05 compared with the 0-dose treatment result.
Intraperitoneal treatment of mice with 5A-POPC (30 mg/kg) was found to promote cholesterol efflux from intraperitoneal RAW 264.7 macrophages labeled with 3H-cholesterol (Fig. 7). The C57BL/6 mice were treated with 5A-POPC 8 h before and again within 1 h of injection of macrophages. Twenty-four hours after intraperitoneal injection of the radiolabeled macrophages, the 5A-POPC treatment increased the appearance of radioactive plasma cholesterol by 43 ± 8% over control mice. 5A-POPC treatment also led to an increase in the excretion of the radiotracer as fecal sterols and bile acids after 24 h by 42 ± 6 and 500 ± 40%, respectively. The amount of radiolabeled cholesterol in the liver increased by 13 ± 6% but was not statistically significant (data not shown).
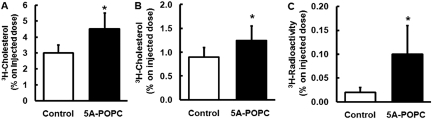
Effect of 5A-POPC treatment on in vivo cholesterol efflux from macrophages. C57BL/6 mice (n = 7) were injected intraperitoneally with RAW 264.7 macrophage radiolabeled with 3H-cholesterol. 5A-POPC (30 mg/kg) was injected intraperitoneally 8 h before and within 1 h before cell injection. Twenty-four hours after cell injection, plasma was monitored for radioactive counts (A), and stool was monitored for fecal sterols (B) and fecal bile acids (C). Results represent the mean ± 1 S.D. of seven replicates. , p < 0.01 compared with the untreated control group.
Effect of 5A-POPC Treatment on Atherosclerosis.
The effect of 5A-POPC treatment on atherosclerosis in apoE-KO mice on a normal chow diet was tested in three different experiments. In the first study (Fig. 8A), we compared the protection provided by 5A-POPC versus POPC liposomes alone. Four-month-old mice were treated intravenously three times a week (Monday, Wednesday, and Friday), with 5A-POPC (30 mg/kg 5A plus 1.25 mg POPC) or POPC liposomes (1.25 mg of POPC) for 13 weeks. Compared with the POPC-treated control group, mice treated with 5A-POPC showed 29% less aortic lesion area (6.6 ± 0.5 versus 9.3 ± 1.2%; p = 0.05). No significant difference was observed from the 5A-POPC or POPC treatment on liver or renal function tests or body weight (Table 1).

Effect of 5A-POPC treatment on atherosclerosis. Each set of data are represented by a scattered dot plot (left) and a box and whiskers plot (right). A and B, 4-month-old apoE-KO mice (n = 8) treated intravenously with POPC or 5A-POPC (30 mg/kg) (A) or 8-month old apoE-KO mice (n = 8) treated intravenously with saline or 5A-POPC (30 mg/kg) (B) on a normal chow diet were injected every Monday, Wednesday, and Friday for 13 weeks and then analyzed for percentage of surface area coverage of aortic plaque. C, 4-month old apoE-KO mice (n = 8) on a normal chow diet were injected intraperitoneally with 5A-SM/DPCC (30 mg/kg) or saline every Monday, Wednesday, and Friday for 13 weeks and then analyzed for percentage of surface area coverage of aortic plaque.
TABLE 1
Effect of 5A-POPC treatment of plasma chemistry profile and body weight
Four-month-old apoE-KO mice (n = 8) were injected intravenously with 5A-POPC (30 mg/kg) every Monday, Wednesday, and Friday for 13 weeks and weighed and then plasma was analyzed for the indicated parameters. Results represent the mean ± 1 S.D. of eight replicates.
Parameter | POPC | 5A-POPC | p Value |
---|---|---|---|
Aspartate aminotransferase (U/l) | 85 ± 10 | 81 ± 13 | 0.8 |
Alanine aminotransferase (U/l) | 38 ± 5 | 39 ± 2 | 0.9 |
LDH (U/l) | 252 ± 18 | 274 ± 39 | 0.62 |
Creatinine (mg/dl) | 0.14 ± 0.02 | 0.11 ± 0.03 | 0.3 |
Blood urea nitrogen (mg/dl) | 19 ± 0.5 | 19 ± 1 | 0.83 |
Glucose (mg/dl) | 135 ± 2 | 144 ± 9 | 0.48 |
Weight (g) | 26 ± 2 | 24 ± 0.5 | 0.2 |
In a second study, 8-month-old mice, with advanced atherosclerotic plaques, were treated with 5A-POPC (30 mg/kg i.v.) three times a week for 13 weeks (Fig. 8B) and compared with saline-treated mice. At the end of the study, the mice were found to have approximately 4-fold more atherosclerosis than what was observed in the first study of the younger mice, but the 5A-POPC treated mice showed 30% less aortic surface coverage than saline-injected mice (28.8 ± 4.7 versus 41.1 ± 4.8%, p = 0.03). In a third study (Fig. 8C), 4-month-old mice were treated with a different lipid formulation of 5A. Based on the observation that nascent HDL is enriched in SM, which can lead to increased efflux of cholesterol from cells (Liu et al., 2003), 5A was reconstituted with 5A-SM/DPPC (1:3.5:3.5 M ratio), and it was injected intraperitoneally three times a week for 13 weeks. In this study control mice received only saline injections. Compared with the control group, 54% less plaque surface coverage of the aorta was observed with the 5A treatment (3.2 ± 0.4 versus 6.9 ± 0.6%, p = 0.001).
Discussion
ApoA-I preparations used to treat patients have first been reconstituted with phospholipid to mimic nascent type HDL (Nissen, 2005; Tardif et al., 2007), which is particularly good in promoting cholesterol efflux, especially by ABCA1 (Mukhamedova et al., 2007). We, therefore, first tested the effect of reconstituting 5A with phospholipid on cholesterol efflux (Fig. 1). Compared with the free 5A peptide, no difference was found between lipid-free 5A and 5A-POPC in the Vmax for cholesterol efflux by ABCA1, although the phospholipid addition seemed to increase the affinity of the peptide complex to ABCA1 based on its lower Km (Fig. 1). A similar finding has recently been made for another amphipathic peptide (Bielicki et al., 2010). These results suggest that either the 5A-POPC complex can directly interact with ABCA1 in the same way that pre-β HDL has recently been proposed to be both a product and substrate of the ABCA1 (Duong et al., 2008) or 5A can dissociate from the complex and then interact with the transporter. A potential advantage of reconstituting 5A with phospholipid is that it also increased cholesterol efflux by ABCG1, which, unlike ABCA1, prefers phospholipid-rich acceptor particles (Cavelier et al., 2006). The addition of phospholipid to 5A caused even a larger increase in cholesterol efflux from the mock-transfected control cells, which most likely is occurring by an aqueous diffusion process, which also requires phospholipid-rich acceptor particles (Yancey et al., 2003).
Ex vivo experiments with human plasma revealed that the 5A peptide has a preference for interacting with HDL, but it can also bind to LDL, depending on the relative concentration of the two lipoproteins (Figs. 2 and and3).3). It is noteworthy that it seemed to transfer cholesterol from LDL particles to HDL, but the main effect of 5A-POPC treatment of plasma was a marked increase in cholesterol and in particular the phospholipid content of HDL (Figs. 2 and and3).3). The phospholipid content of HDL from mice treated with 5A-POPC was also increased (Fig. 4). Enrichment of phospholipid on HDL has been shown to increase the capacity of HDL for removing cholesterol from cells (Davidson et al., 1994; Yancey et al., 2003; Silva et al., 2008), which may have accounted for the increased ability of serum from treated mice to promote more cholesterol efflux (Fig. 5).
Using two different approaches, 5A-POPC treatment was shown to increase the in vivo flux of cholesterol (Figs. 6 and and7).7). 5A-POPC mobilized radiolabeled cholesterol from peritoneal macrophages and increased the excretion of fecal neutral sterols and bile salts (Fig. 7). Likewise, 5A-POPC was also found to increase the global efflux of cholesterol from peripheral tissues, as measured in the cholesterol isotope dilution study, but it did not seem to increase overall fecal neutral sterol excretion of sterols or bile salts by this method (Fig. 6). This difference may be caused by differences in the experimental details of these two studies, such as dose, route, and number of 5A treatments and animal model. Multiple treatments with 5A-POPC may also be needed to deliver enough cholesterol to liver to then up-regulate the expression of hepatic genes that facilitate the excretion of fecal sterols and bile salts. It is also likely that the cholesterol isotope dilution method is relatively insensitive to the flux of cholesterol from macrophages, because of its small pool size compared with the whole body. Mobilization of cholesterol from macrophages and redistribution to other tissues, however, may still be sufficient for reducing atherosclerosis, without increased fecal loss of sterols (Remaley et al., 2008). For example, it has been shown in mice that acute infusion of HDL mobilizes cholesterol from peripheral tissues, which will reduce atherosclerosis, but does not increase the level of fecal sterols (Alam et al., 2001).
Treatment of mice with 5A was found to reduce atherosclerosis (Fig. 8) comparable with what has been previously observed with apoA-I and D-4F (Kawashiri et al., 2002). Reconstitution of 5A with sphingomyelin and DPPC seemed to be even more effective than when 5A was reconstituted with POPC (Fig. 8), although it was delivered by intraperitoneal injection instead of intravenously like the other treatments. More studies are needed to investigate the route of delivery and the effect of different types and amounts of phospholipids on their ability to alter the antiatherogenic properties of amphipathic peptides. One study has already reported that different types of phospholipids affect the ability of apoA-I to reduce inflammation in endothelial cells (Baker et al., 2000). It should also be noted that we only examined progression of atherosclerosis and that in the future regression models will also have to be used to determine whether 5A and related peptides can reduce existing disease, which is more relevant to how these peptides would likely be used to treat patients.
Recently, a single helical peptide called ATI-5261, which also promotes cholesterol efflux by ABCA1, was also found to reduce atherosclerosis in mice (Bielicki et al., 2010). Although the results from ATI-5261 and the 5A peptide are consistent with the concept that these peptides reduce atherosclerosis in mice, because of their ability to promote cholesterol efflux, it does not exclude other possibilities. In fact, 5A was recently observed to be as potent as apoA-I in reducing inflammation in a rabbit collar model (Tabet et al., 2010). In this study, it was found that 5A decreased the expression of vascular cell adhesion molecule and intercellular adhesion molecule on endothelial cells and decreased reactive oxygen species production in endothelial cells (Tabet et al., 2010). 5A has also been found to reduce the activation of the adhesion protein CD11 on monocytes (Murphy et al., 2008). Cholesterol efflux from cells, such as macrophages, has been shown to decrease their inflammatory phenotype (Yvan-Charvet et al., 2010; deGoma et al., 2008), so the ability of 5A to promote cholesterol efflux can perhaps still be related to its anti-inflammatory properties. The D-4F peptide has also been shown to reduce platelet activation (Buga et al., 2010), enhance the antioxidant properties of HDL, and sequester oxidized lipids (Van Lenten et al., 2008). The 5A peptide has not been tested for these other antiatherogenic properties, but given the fact that the key structural motif that seems to be necessary for all of these processes is the presence of an amphipathic helix, these properties may be shared by other amphipathic peptides.
In summary, the 5A peptide, which was designed to specifically efflux cholesterol by the ABCA1, was found when injected into mice to increase HDL, particularly its phospholipid concentration, increase cholesterol efflux from macrophages and other peripheral tissues, and reduce atherosclerosis. These features and its apparent lack of toxicity make it a potential substitute for apoA-I in HDL therapy. Additional structure–function studies of 5A and related peptides may not only enhance the antiatherogenic effects of these peptides, but may also lead to a better understanding of which properties of HDL are most important for reducing atherosclerosis.
Acknowledgments
We thank Dr. Jack Oram for generously providing the ABCA1- and ABCG1-transfected BHK cells.
This work was supported by the Intramural Research Program of the National Institutes of Health, National Heart, Lung, and Blood Institute. W.D. was supported by the National Health and Medical Research Council of Australia [Australian Postgraduate Award 526709]. D.S. is a Fellow of the Health and Medical Research Council of Australia [Award 586607].
Article, publication date, and citation information can be found at http://jpet.aspetjournals.org.
10.1124/jpet.110.167890.
ABBREVIATIONS:
- apo
- apolipoprotein
- Athero
- atherosclerosis
- HDL
- high-density lipoprotein
- LDL
- low-density lipoprotein
- RCT
- reverse cholesterol transport
- POPC
- palmitoyl oleoyl phosphatidyl choline
- ABC
- ATP-binding cassette transporter
- KO
- knockout
- DPPC
- dipalmitoyl phosphatidylcholine
- SM
- sphingomyelin
- FPLC
- fast-protein liquid chromatography
- BHK
- baby hamster kidney
- BA
- bile acids
- NS
- neutral sterols
- 13C2-C
- [2,3-13C2]cholesterol
- GC
- gas chromatography
- MS
- mass spectrometry
References
- Alam K, Meidell RS, Spady DK. (2001) Effect of up-regulating individual steps in the reverse cholesterol transport pathway on reverse cholesterol transport in normolipidemic mice. J Biol Chem 276:15641–15649 [Abstract] [Google Scholar]
- Amar MJ, Dugi KA, Haudenschild CC, Shamburek RD, Foger B, Chase M, Bensadoun A, Hoyt RF, Jr, Brewer HB, Jr, Santamarina-Fojo S. (1998) Hepatic lipase facilitates the selective uptake of cholesteryl esters from remnant lipoproteins in apoE-deficient mice. J Lipid Res 39:2436–2442 [Abstract] [Google Scholar]
- Baker PW, Rye KA, Gamble JR, Vadas MA, Barter PJ. (2000) Phospholipid composition of reconstituted high density lipoproteins influences their ability to inhibit endothelial cell adhesion molecule expression. J Lipid Res 41:1261–1267 [Abstract] [Google Scholar]
- Barter P. (2009) Lessons learned from the Investigation of Lipid Level Management to Understand its Impact in Atherosclerotic Events (ILLUMINATE) trial. Am J Cardiol 104:10E–15E [Abstract] [Google Scholar]
- Bielicki JK, Zhang H, Cortez Y, Zheng Y, Narayanaswami V, Patel A, Johansson J, Azhar S. (2010) A new HDL mimetic peptide that stimulates cellular cholesterol efflux with high efficiency greatly reduces atherosclerosis in mice. J Lipid Res 51:1496–1503 [Europe PMC free article] [Abstract] [Google Scholar]
- Bloedon LT, Dunbar R, Duffy D, Pinell-Salles P, Norris R, DeGroot BJ, Movva R, Navab M, Fogelman AM, Rader DJ. (2008) Safety, pharmacokinetics, and pharmacodynamics of oral apoA-I mimetic peptide D-4F in high-risk cardiovascular patients. J Lipid Res 49:1344–1352 [Europe PMC free article] [Abstract] [Google Scholar]
- Buga GM, Navab M, Imaizumi S, Reddy ST, Yekta B, Hough G, Chanslor S, Anantharamaiah GM, Fogelman AM. (2010) L-4F alters hyperlipidemic (but not healthy) mouse plasma to reduce platelet aggregation. Arterioscler Thromb Vasc Biol 30:283–289 [Europe PMC free article] [Abstract] [Google Scholar]
- Cavelier C, Lorenzi I, Rohrer L, von Eckardstein A. (2006) Lipid efflux by the ATP-binding cassette transporters ABCA1 and ABCG1. Biochim Biophys Acta 1761:655–666 [Abstract] [Google Scholar]
- Davidson WS, Lund-Katz S, Johnson WJ, Anantharamaiah GM, Palgunachari MN, Segrest JP, Rothblat GH, Phillips MC. (1994) The influence of apolipoprotein structure on the efflux of cellular free cholesterol to high density lipoprotein. J Biol Chem 269:22975–22982 [Abstract] [Google Scholar]
- deGoma EM, deGoma RL, Rader DJ. (2008) Beyond high-density lipoprotein cholesterol levels evaluating high-density lipoprotein function as influenced by novel therapeutic approaches. J Am Coll Cardiol 51:2199–2211 [Europe PMC free article] [Abstract] [Google Scholar]
- Duffy D, Rader DJ. (2006) Emerging therapies targeting high-density lipoprotein metabolism and reverse cholesterol transport. Circulation 113:1140–1150 [Abstract] [Google Scholar]
- Duong PT, Weibel GL, Lund-Katz S, Rothblat GH, Phillips MC. (2008) Characterization and properties of pre-β-HDL particles formed by ABCA1-mediated cellular lipid efflux to apoA-I. J Lipid Res 49:1006–1014 [Europe PMC free article] [Abstract] [Google Scholar]
- Folch J, Lees M, Sloane Stanley GH. (1957) A simple method for the isolation and purification of total lipides from animal tissues. J Biol Chem 226:497–509 [Abstract] [Google Scholar]
- Garcia RA. (2008) Pharmacological therapies for raising HDL cholesterol beyond synthetic small molecules. Curr Opin Investig Drugs 9:274–280 [Abstract] [Google Scholar]
- Jonas A. (1986) Reconstitution of high-density lipoproteins. Methods Enzymol 128:553–582 [Abstract] [Google Scholar]
- Kawashiri MA, Zhang Y, Puré E, Rader DJ. (2002) Combined effects of cholesterol reduction and apolipoprotein A-I expression on atherosclerosis in LDL receptor deficient mice. Atherosclerosis 165:15–22 [Abstract] [Google Scholar]
- Liu L, Bortnick AE, Nickel M, Dhanasekaran P, Subbaiah PV, Lund-Katz S, Rothblat GH, Phillips MC. (2003) Effects of apolipoprotein A-I on ATP-binding cassette transporter A1-mediated efflux of macrophage phospholipid and cholesterol: formation of nascent high density lipoprotein particles. J Biol Chem 278:42976–42984 [Abstract] [Google Scholar]
- Matsuura F, Wang N, Chen W, Jiang XC, Tall AR. (2006) HDL from CETP-deficient subjects shows enhanced ability to promote cholesterol efflux from macrophages in an apoE- and ABCG1-dependent pathway. J Clin Invest 116:1435–1442 [Europe PMC free article] [Abstract] [Google Scholar]
- Mukhamedova N, Escher G, D'Souza W, Tchoua U, Grant A, Krozowski Z, Bukrinsky M, Sviridov D. (2008) Enhancing apolipoprotein A-I-dependent cholesterol efflux elevates cholesterol export from macrophages in vivo. J Lipid Res 49:2312–2322 [Abstract] [Google Scholar]
- Mukhamedova N, Fu Y, Bukrinsky M, Remaley AT, Sviridov D. (2007) The role of different regions of ATP-binding cassette transporter A1 in cholesterol efflux. Biochemistry 46:9388–9398 [Abstract] [Google Scholar]
- Murphy AJ, Woollard KJ, Hoang A, Mukhamedova N, Stirzaker RA, McCormick SP, Remaley AT, Sviridov D, Chin-Dusting J. (2008) High-density lipoprotein reduces the human monocyte inflammatory response. Arterioscler Thromb Vasc Biol 28:2071–2077 [Abstract] [Google Scholar]
- Nanjee MN, Crouse JR, King JM, Hovorka R, Rees SE, Carson ER, Morgenthaler JJ, Lerch P, Miller NE. (1996) Effects of intravenous infusion of lipid-free apo A-I in humans. Arterioscler Thromb Vasc Biol 16:1203–1214 [Abstract] [Google Scholar]
- Navab M, Anantharamaiah GM, Reddy ST, Hama S, Hough G, Grijalva VR, Wagner AC, Frank JS, Datta G, Garber D, et al. (2004) Oral D-4F causes formation of pre-β high-density lipoprotein and improves high-density lipoprotein-mediated cholesterol efflux and reverse cholesterol transport from macrophages in apolipoprotein E-null mice. Circulation 109:3215–3220 [Abstract] [Google Scholar]
- Nissen SE. (2005) Effect of intensive lipid lowering on progression of coronary atherosclerosis: evidence for an early benefit from the Reversal of Atherosclerosis with Aggressive Lipid Lowering (REVERSAL) trial. Am J Cardiol 96:61F–68F [Abstract] [Google Scholar]
- Nissen SE, Tsunoda T, Tuzcu EM, Schoenhagen P, Cooper CJ, Yasin M, Eaton GM, Lauer MA, Sheldon WS, Grines CL, et al. (2003) Effect of recombinant ApoA-I Milano on coronary atherosclerosis in patients with acute coronary syndromes: a randomized controlled trial. J Am Med Assoc 290:2292–2300 [Abstract] [Google Scholar]
- Ostlund RE, Jr, Matthews DE. (1993) [13C]cholesterol as a tracer for studies of cholesterol metabolism in humans. J Lipid Res 34:1825–1831 [Abstract] [Google Scholar]
- Remaley AT, Amar M, Sviridov D. (2008) HDL-replacement therapy: mechanism of action, types of agents and potential clinical indications. Expert Rev Cardiovasc Ther 6:1203–1215 [Abstract] [Google Scholar]
- Remaley AT, Stonik JA, Demosky SJ, Neufeld EB, Bocharov AV, Vishnyakova TG, Eggerman TL, Patterson AP, Duverger NJ, Santamarina-Fojo S, et al. (2001) Apolipoprotein specificity for lipid efflux by the human ABCAI transporter. Biochem Biophys Res Commun 280:818–823 [Abstract] [Google Scholar]
- Remaley AT, Thomas F, Stonik JA, Demosky SJ, Bark SE, Neufeld EB, Bocharov AV, Vishnyakova TG, Patterson AP, Eggerman TL, et al. (2003) Synthetic amphipathic helical peptides promote lipid efflux from cells by an ABCA1-dependent and an ABCA1-independent pathway. J Lipid Res 44:828–836 [Abstract] [Google Scholar]
- Sankaranarayanan S, Oram JF, Asztalos BF, Vaughan AM, Lund-Katz S, Adorni MP, Phillips MC, Rothblat GH. (2009) Effects of acceptor composition and mechanism of ABCG1-mediated cellular free cholesterol efflux. J Lipid Res 50:275–284 [Europe PMC free article] [Abstract] [Google Scholar]
- Sethi AA, Amar M, Shamburek RD, Remaley AT. (2007) Apolipoprotein AI mimetic peptides: possible new agents for the treatment of atherosclerosis. Curr Opin Investig Drugs 8:201–212 [Abstract] [Google Scholar]
- Sethi AA, Stonik JA, Thomas F, Demosky SJ, Amar M, Neufeld E, Brewer HB, Davidson WS, D'Souza W, Sviridov D, et al. (2008) Asymmetry in the lipid affinity of bihelical amphipathic peptides. A structural determinant for the specificity of ABCA1-dependent cholesterol efflux by peptides. J Biol Chem 283:32273–32282 [Europe PMC free article] [Abstract] [Google Scholar]
- Silva RA, Huang R, Morris J, Fang J, Gracheva EO, Ren G, Kontush A, Jerome WG, Rye KA, Davidson WS. (2008) Structure of apolipoprotein A-I in spherical high density lipoproteins of different sizes. Proc Natl Acad Sci USA 105:12176–12181 [Europe PMC free article] [Abstract] [Google Scholar]
- Tabet F, Remaley AT, Segaliny AI, Millet J, Yan L, Nakhla S, Barter PJ, Rye KA, Lambert G. (2010) The 5A apolipoprotein A-I mimetic peptide displays antiinflammatory and antioxidant properties in vivo and in vitro. Arterioscler Thromb Vasc Biol 30:246–252 [Europe PMC free article] [Abstract] [Google Scholar]
- Tardif JC, Gregoire J, L'Allier PL, Ibrahim R, Lesperance J, Heinonen TM, Kouz S, Berry C, Basser R, Lavoie MA, et al. (2007) Effects of reconstituted high-density lipoprotein infusions on coronary atherosclerosis: a randomized controlled trial. J Am Med Assoc 297:1675–1682 [Abstract] [Google Scholar]
- Van Lenten BJ, Wagner AC, Jung CL, Ruchala P, Waring AJ, Lehrer RI, Watson AD, Hama S, Navab M, Anantharamaiah GM, et al. (2008) Anti-inflammatory apoA-I-mimetic peptides bind oxidized lipids with much higher affinity than human apoA-I. J Lipid Res 49:2302–2311 [Europe PMC free article] [Abstract] [Google Scholar]
- Vaughan AM, Oram JF. (2003) ABCA1 redistributes membrane cholesterol independent of apolipoprotein interactions. J Lipid Res 44:1373–1380 [Abstract] [Google Scholar]
- Wool GD, Vaisar T, Reardon CA, Getz GS. (2009) An apoA-I mimetic peptide containing a proline residue has greater in vivo HDL binding and anti-inflammatory ability than the 4F peptide. J Lipid Res 50:1889–1900 [Europe PMC free article] [Abstract] [Google Scholar]
- Yancey PG, Bortnick AE, Kellner-Weibel G, de la Llera-Moya M, Phillips MC, Rothblat GH. (2003) Importance of different pathways of cellular cholesterol efflux. Arterioscler Thromb Vasc Biol 23:712–719 [Abstract] [Google Scholar]
- Yvan-Charvet L, Wang N, Tall AR. (2010) Role of HDL, ABCA1, and ABCG1 transporters in cholesterol efflux and immune responses. Arterioscler Thromb Vasc Biol 30:139–143 [Europe PMC free article] [Abstract] [Google Scholar]
Articles from The Journal of Pharmacology and Experimental Therapeutics are provided here courtesy of American Society for Pharmacology and Experimental Therapeutics
Full text links
Read article at publisher's site: https://doi.org/10.1124/jpet.110.167890
Read article for free, from open access legal sources, via Unpaywall:
https://europepmc.org/articles/pmc2913774?pdf=render
Free after 12 months at intl-jpet.aspetjournals.org
http://intl-jpet.aspetjournals.org/cgi/reprint/334/2/634.pdf
Free after 12 months at intl-jpet.aspetjournals.org
http://intl-jpet.aspetjournals.org/cgi/content/full/334/2/634
Free to read at intl-jpet.aspetjournals.org
http://intl-jpet.aspetjournals.org/cgi/content/abstract/334/2/634
Citations & impact
Impact metrics
Article citations
Novel peptide inhibitors targeting CD40 and CD40L interaction: A potential for atherosclerosis therapy.
Curr Res Struct Biol, 6:100110, 14 Nov 2023
Cited by: 0 articles | PMID: 38106460 | PMCID: PMC10724548
Proteomic Determinants of Variation in Cholesterol Efflux: Observations from the Dallas Heart Study.
Int J Mol Sci, 24(21):15526, 24 Oct 2023
Cited by: 0 articles | PMID: 37958510 | PMCID: PMC10648649
Apolipoproteins as potential communicators play an essential role in the pathogenesis and treatment of early atherosclerosis.
Int J Biol Sci, 19(14):4493-4510, 21 Aug 2023
Cited by: 5 articles | PMID: 37781031 | PMCID: PMC10535700
Review Free full text in Europe PMC
Apolipoprotein-mimetic nanodiscs reduce lipid accumulation and improve liver function in acid sphingomyelinase deficiency.
Nanomedicine, 53:102705, 24 Aug 2023
Cited by: 0 articles | PMID: 37633404 | PMCID: PMC10530155
A Current Update on the Role of HDL-Based Nanomedicine in Targeting Macrophages in Cardiovascular Disease.
Pharmaceutics, 15(5):1504, 15 May 2023
Cited by: 4 articles | PMID: 37242746 | PMCID: PMC10221824
Review Free full text in Europe PMC
Go to all (73) article citations
Similar Articles
To arrive at the top five similar articles we use a word-weighted algorithm to compare words from the Title and Abstract of each citation.
Combined deficiency of ABCA1 and ABCG1 promotes foam cell accumulation and accelerates atherosclerosis in mice.
J Clin Invest, 117(12):3900-3908, 01 Dec 2007
Cited by: 347 articles | PMID: 17992262 | PMCID: PMC2066200
The combination of L-4F and simvastatin stimulate cholesterol efflux and related proteins expressions to reduce atherosclerotic lesions in apoE knockout mice.
Lipids Health Dis, 12:180, 08 Dec 2013
Cited by: 4 articles | PMID: 24314261 | PMCID: PMC3866605
Simvastatin reduces atherogenesis and promotes the expression of hepatic genes associated with reverse cholesterol transport in apoE-knockout mice fed high-fat diet.
Lipids Health Dis, 10:8, 18 Jan 2011
Cited by: 29 articles | PMID: 21241519 | PMCID: PMC3031258
ATP-binding cassette transporters A1 and G1, HDL metabolism, cholesterol efflux, and inflammation: important targets for the treatment of atherosclerosis.
Curr Drug Targets, 12(5):647-660, 01 May 2011
Cited by: 69 articles | PMID: 21039336
Review
Funding
Funders who supported this work.