Abstract
Free full text

NMR resonance assignments of thrombin reveal the conformational and dynamic effects of ligation
Associated Data
Abstract
The serine protease thrombin is generated from its zymogen prothrombin at the end of the coagulation cascade. Thrombin functions as the effector enzyme of blood clotting by cleaving several procoagulant targets, but also plays a key role in attenuating the hemostatic response by activating protein C. These activities all depend on the engagement of exosites on thrombin, either through direct interaction with a substrate, as with fibrinogen, or by binding to cofactors such as thrombomodulin. How thrombin specificity is controlled is of central importance to understanding normal hemostasis and how dysregulation causes bleeding or thrombosis. The binding of ligands to thrombin via exosite I and the coordination of Na+ have been associated with changes in thrombin conformation and activity. This phenomenon has become known as thrombin allostery, although direct evidence of conformational change, identification of the regions involved, and the functional consequences remain unclear. Here we investigate the conformational and dynamic effects of thrombin ligation at the active site, exosite I and the Na+-binding site in solution, using modern multidimensional NMR techniques. We obtained full resonance assignments for thrombin in seven differently liganded states, including fully unliganded apo thrombin, and have created a detailed map of residues that change environment, conformation, or dynamic state in response to each relevant single or multiple ligation event. These studies reveal that apo thrombin exists in a highly dynamic zymogen-like state, and relies on ligation to achieve a fully active conformation. Conformational plasticity confers upon thrombin the ability to be at once selective and promiscuous.
Blood coagulation (hemostasis) is the result of a cascade of events where zymogens are converted to active proteases through the specific action of a preceding protease (1, 2). This process is tightly regulated to ensure that stable blood clots form rapidly at the site of tissue damage. Dysregulation by several mechanisms is the cause of bleeding disorders, including hemophilia, and of thrombosis, the most common cause of morbidity and mortality in the industrialized world. Most hemostatic proteases have only a single target and are regulated by a single cofactor. However, thrombin (Fig. 1), the final protease in the cascade, has over a dozen substrates and at least five cofactors (3). Thrombin is critical for the initiation, propagation, and attenuation of the hemostatic response, and in each of these phases the activity of thrombin is directed by cofactors (4, 5). Of principal importance are the cofactors that convert thrombin between pro and anticoagulant activities. Thrombomodulin (TM) is an integral membrane protein that alters thrombin specificity from the procoagulant substrates such as PAR1, factors V and VIII, and fibrinogen to specific activation of the anticoagulant protease protein C (6). This change of function is thought to be due to the blocking of exosite I by TM, optimal presentation of the substrate, and possible allosteric effects (5). Conversely, the binding of thrombin to its cofactor Na+ converts it from an anticoagulant state to a potent procoagulant state (7). The importance of thrombin in determining the hemostatic balance justifies the vast body of research into its structure, how cofactors and substrates are recognized, and how specificity is conferred. Thrombin’s structure-activity relationship, however, remains incompletely understood, especially with respect to the role played by ligand-induced conformational change, often referred to as thrombin allostery.
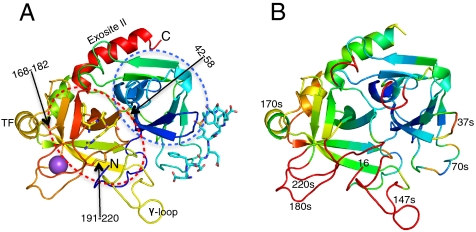
Thrombin structure. (A) Ribbon diagram of thrombin (heavy chain only) colored from N to C terminus from blue to red, with disulfide bonds shown as sticks and indicated by arrows (made with PyMol (37)). The N-terminal β-barrel is predominantly blue and is indicated by the blue broken oval, and the C-terminal β-barrel is yellow and orange and is indicated by the red broken oval. The N and C termini are indicated, as is exosite II, the γ-loop and the 170s loop (labeled TF to indicate the tissue factor binding site of fVIIa). PPACK (yellow sticks) is shown bound in the active-site cleft, hirugen (cyan sticks) is shown bound to exosite I, and Na+ (purple ball) is shown between the 180s loop (orange) and the 220s loop (red). (B) The heavy chain colored according to Cα RMSD between active thrombin (1JOU_AB) and the zymogen form of thrombin (prethrombin-2, blue at 0 to red at 2 Å shift). Important loops are indicated.
Allostery is a common theme in hemostasis. Each of the protease activation events occurs through a well-described allosteric mechanism in which the newly formed N terminus (residue 16 in chymotrypsin numbering) inserts into the “activation pocket” to form a salt bridge with Asp194, thereby stabilizing the catalytic loop containing Ser195 and the oxyanion hole (Gly193 and Ser195) (8). Crystal structures have been solved for several chymotrypsin family zymogens, and show a consistent blocking/disordering of the primary specificity pocket (S1 pocket in the traditional nomenclature (9)) and a rearrangement/disordering of the catalytic loop. This disordered/flexible to ordered/rigid conformational transition involves a discrete portion of the protein, the so-called “zymogen activation domain” composed of residues 142–153, 184–194, and 215–225, and, of course, the loop containing the new N terminus, residues 16–18 (Fig. 1B). This entire region lies in the C-terminal β-barrel domain, and makes up the activation pocket, the S1 pocket, and the catalytic loop. Although formation of the new N terminus is sufficient for full conversion to the active state for most proteases, there are examples in highly regulated processes where cofactors are required to complete the conformational change. A well characterized example is the activation of the initiating coagulation protease, factor VIIa, by tissue factor (TF) (10). TF does not bind directly in the zymogen activation domain, but to adjacent loops composed of residues 131–134 and 164–167 (thrombin numbering) (Fig. 1A). In such cases, cofactors act as allosteric activators to provide an additional level of regulatory control.
Similarly, TM has been shown to affect the structure and activity of thrombin (11). TM binds to exosite I of thrombin, remote from the zymogen activation domain, yet affects the rate of cleavage of several substrates, the accessibility of the S1 pocket, and the general features of the active-site cleft (12–16). These effects are not unique to TM, and apply to any exosite-I-binding ligand, including the C-terminal peptide derived from hirudin (known as hirugen) (16). Binding of inhibitors to the active-site cleft increases the affinity of thrombin for exosite I ligands, confirming the thermodynamic linkage between the two sites (17). In spite of the evidence that TM binding alters the conformation of thrombin, crystallographic structures of the complex did not reveal any significant differences when compared to active-site inhibited thrombin (18, 19). The reasons for the lack of conformational change are unclear, but may be due to the occupancy of the active-site cleft by a peptidyl inhibitor in the two structures. An alternative explanation is that crystal contacts will similarly stabilize thrombin in a conformational state that is not representative of its state (or equilibrium between states) in solution.
Like TM, Na+ binding has been shown to affect the structure and activity of thrombin (20). The Na+-free form has become known as “slow” thrombin due to its overall poor proteolytic efficiency relative to the Na+-bound “fast” form, and spectroscopic studies have suggested significant differences in conformation. The location of the Na+-binding site (21) in part of the zymogen activation domain (residues 221a and 224 coordinate Na+) suggests a role for Na+ binding in the zymogen-to-enzyme conformational transition. The extended Na+-binding loop is composed of residues 215–225, and these and adjacent residues on the 180s loop (184–194) form the S1 pocket, and help to explain observations that binding to Na+ opens up the active-site cleft and the primary specificity pocket (S1) of thrombin (20). Unsurprisingly, the binding of Na+ is thermodynamically linked to active site binding and exosite I binding, and it has been demonstrated that exosite I binding has the same effect on the S1 pocket as Na+ binding (17, 19). However, the nature of the Na+-induced conformational change is still unclear. Several structures have been solved of thrombin in the absence of Na+ and of variants deficient in Na+ binding, however, again ligation and crystal contacts are likely to have affected the structures to either mask the actual conformational change or induce an artifactual conformation (22). The obvious connection between the Na+-binding site and the zymogen-activation domain suggests that unliganded thrombin is zymogen-like, and that it does not exist in a single rigid conformational state, but as an ensemble of conformations in equilibrium (8). Addressing the issue of thrombin allostery has thus proved problematic using X-ray crystallography, and is likely to require a technique sensitive to the conformation and dynamics of thrombin in solution.
Five years ago we undertook the ambitious task of determining the effect of thrombin ligation in solution at three energetically linked sites, the active-site cleft, exosite I, and the Na+-binding site, using NMR. Two-dimensional 1H, 15N TROSY spectra provided a sensitive fingerprint for each state, and three-dimensional experiments were conducted to assign each resonance peak with its corresponding residue. We thus assigned seven distinct ligation states involving the active-site inhibitor PPACK, the exosite I ligand hirugen, and Na+. We conclude that thrombin is a highly dynamic molecule in the absence of ligation, and that exosite I occupancy and Na+ binding each individually stabilize and rigidify thrombin, but that the formation of the fully active state requires binding of both, or occupancy of the active site. Thus, thrombin is similar to fVIIa and requires the binding of cofactors to complete the zymogen-to-enzyme conversion. Implications for the role of thrombin allostery in hemostasis are discussed.
Results
Strategy.
Two-dimensional 1H, 15N heteronuclear correlation spectra (HSQC) spectra of uniformly 15N labeled protein samples provide a fingerprint of the local environment of backbone amides and give detailed insight into the structure and dynamics of a protein. Three-dimensional triple-resonance experiments are generally used to extend these amide resonances via their covalently linked nitrogens into a third (carbon) dimension which ultimately yields sequence assignments for the residues responsible for the original HSQC spectrum. Resonance assignment of larger proteins such as thrombin (35 kDa and ~300 residues) is hampered by two global factors: resonance overlap due to the large number of peaks (one per nonproline main chain residue and certain side chains); and a general decrease in signal intensities caused by fast relaxation due to slow overall tumbling. These limitations can be overcome to some degree by deuteration of all nonexchangeable side chain protons, effectively eliminating proton-proton (spin-spin) interactions. Using Transverse Relaxation-Optimized Spectroscopy (TROSY), we were thus able to obtain high quality (high signal-to-noise ratio and peak dispersion) two-dimensional spectra of thrombin in several ligated states. However, it was immediately clear that the number of observed peaks increased with ligation, and that the fully unliganded state produced the spectrum with the fewest resolvable resonance peaks. This loss of signal with deligation is generally the result of conformational exchange processes on an intermediate μs to ms time scale, which leads to extreme broadening of signals and reflects an equilibrium between distinct conformational states. Such motions on a time scale slower than the overall tumbling rate often involve discrete protein segments, such as loops or secondary structure elements.
A full resonance assignment requires the sequential linking of residues through three-dimensional experiments, and is made difficult when certain stretches of the protein sequence (conformationally dynamic segments) do not provide peaks of sufficient signal intensity. For the complete resonance assignment of thrombin we started with the state that produced the largest number of two-dimensional TROSY amide peaks, namely fully liganded thrombin, with PPACK occupying the active site, hirugen binding in exosite I, and Na+ coordinating in the 220s loop. Starting from this well defined, rigid state it would then be possible to assign the experimentally more challenging partially liganded and fully unliganded (apo) thrombins (Fig. 2). By identifying the resonances that shifted or disappeared upon deligation we could determine the residues that changed environment, conformation, or dynamics in response to each ligation event (or each deligation event, as illustrated in Fig. 2).
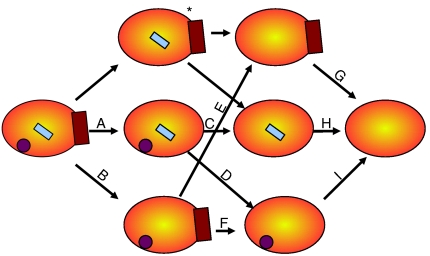
Graphical depiction of the deligation strategy. Schematic representations of thrombin (orange oval), Na+ (purple circle), PPACK (cyan rectangle) and hirugen (brown rectangle) are used to show the different deligation events. Single deligation events analyzed in this article are connected by arrows and labeled with letters. The same labeling scheme is used in Fig. 4, Table S2 and Fig. S2. Assignments for PPACKed, hirugen-bound thrombin in the absence of Na+ (indicated by an asterisk) were not determined, since PPACK alone orders the Na+-binding site.
Resonance Assignments.
Backbone amide resonances were assigned with TROSY-based triple-resonance experiments correlating CA, CB and C, on side chain-deuterated uniformly 13C, 15N-labeled wild-type thrombin inhibited by PPACK, and bound to hirugen and Na+. The resulting annotated TROSY 1H, 15N correlation map is given as Fig. 3A, and the assignments are deposited in the BioMagResBank (accession number: 16940). Of the 295 residues in α thrombin, 279 nonproline amino acids can potentially contribute a peak to the spectrum. We assigned resonances for 253 of the 279 residues (90.7%, Table S1 and Fig. 3B and Fig. S1). No resonances were observed for segments known to be dynamic, such as the N terminus of the light chain and the autolysis loop (γ-loop). In order to compare resonance assignments for the seven differently liganded states (indicated in Fig. 2) in a meaningful way, we limited our analysis to residues in the heavy chain (the catalytic domain of thrombin), excluding the inherently flexible γ-loop (147a–153). Of the remaining 234 main chain amide-containing residues we assigned 226 backbone resonances (96.6%, Table S1). The N terminus of the heavy chain (Ile16) is excluded from the analysis since primary amines are not detected in standard 1H-15N two-dimensional spectra. Interestingly, active-site residues 193–195 could not be assigned for any of the ligated states, perhaps reflecting the unusual environment surrounding the covalent inhibitor PPACK, or the inherently dynamic nature of the catalytic loop in uninhibited thrombin. Notwithstanding, the resonance assignments for fully liganded thrombin provide a sensitive signal for all of the important regions of thrombin, and thus, a useful starting point for determining the effect of deligation on thrombin structure and dynamics. It is worth noting that the complete assignment obtained for fully liganded thrombin validates our strategy for overcoming the limitations associated with the high molecular weight of the system. The numbers of residues assigned for each ligation state are given in Table S1, and the assignment status of each residue for all seven ligation states is shown as a sequence alignment in Fig. S1. Assigned resonances that underwent a significant change in chemical shift upon a deligation event (≥0.1 ppm) are listed in Table S2. To illustrate the qualitative difference between two liganded states, we present the overlays of the TROSY spectra (Fig. S2), and color a ribbon diagram of thrombin according to resonance shift or disappearance (Fig. 4).
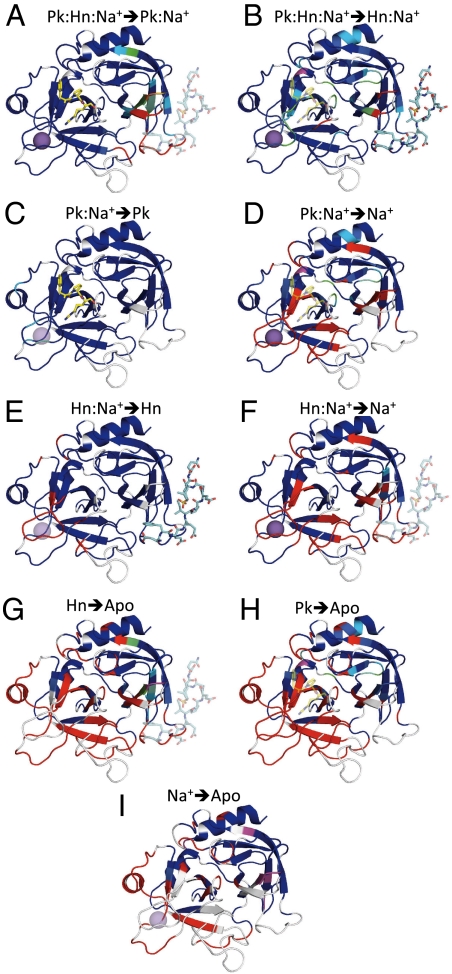
Changes in thrombin structure in response to single deligation events. Each ribbon diagram illustrates significant ppm shifts and resonance disappearance after the removal of the semitransparent ligand. Lettered as in Fig. 2, and above each diagram is a key to the deligation, with Pk for PPACK, Hn for hirugen, and Na+ for the sodium ion. Residues for which resonances are not assigned before the deligation event are white, and assigned residues are colored according to the weighted chemical shift difference from blue (< 0.1 ppm) to orange (> 1.7 ppm). Residues assigned before, but not after deligation are colored red. The purple regions indicate resonances that are identified only in the deliganded state.
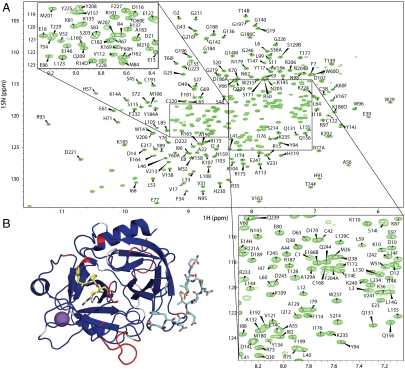
Resonance assignments for fully-liganded thrombin. (A) Two-dimensional 1H-15N TROSY NMR spectrum of PPACKed thrombin bound to hirugen and Na+, with assigned resonances labeled (chymotrypsin numbering). Zoomed views of crowded regions are shown in the insets. The 15N chemical shift of T74 (marked with #) is 97.8 ppm and its cross peak is folded back into the spectrum. Double peaks of the same residue are marked with lowercase letters. Unassigned peaks in the bottom left corner are from tryptophan side chains. (B) Ribbon diagram of fully liganded thrombin (including the light chain) is shown colored according to assignment status, with blue for assigned, red unassigned, and white for prolines.
Discussion
Crystallographic structures of thrombin have revealed many of its secrets, including how exosites are employed to bind substrates and cofactors. However, the role of conformational change in directing thrombin activity has remained enigmatic, due to a problem inherent to protein crystallography—the formation of a crystal necessarily involves crystal contacts. Conformationally dynamic/flexible segments are either unobserved in electron density or are somehow “frozen out” by crystal contacts, which can provide up to 6 kcal/mol free energy (23). Therefore, the observed conformation may not represent the state of the flexible region in solution, and although it might be one of the equilibrium states, it is not necessarily the lowest energy state or even one that is highly populated in solution. Our goal was to determine the effect of ligation on thrombin structure and dynamics in solution using NMR, focusing on three energetically linked sites: the active site, exosite I, and the Na+-binding site, but to do so we had to approach ligation in reverse. That is, we stabilized the dynamic segments of thrombin to obtain and assign resonance signals for 96% of the heavy chain (ignoring the inherently disordered γ-loop) by binding an active-site inhibitor and an exosite I ligand, in the presence of saturating concentrations of Na+. The resulting correlation map (Fig. 3) was then used as reference to assign six sequentially deligated states. In total, we assigned the residues responsible for the TROSY cross peaks for seven distinct ligation states of thrombin. Because we know from crystal structures where each ligand binds to thrombin, it is possible to distinguish between local and long-range conformational effects. In other words, we have succeeded in developing a tool to investigate the effect of ligation on the conformation and dynamics of thrombin under physiological conditions, and can now meaningfully address the issue of thrombin allostery.
Apo Thrombin Is Zymogen-Like.
Apo thrombin is a surprisingly dynamic molecule (illustrated in Fig. S3). Only 50% of potential cross peaks from the heavy chain (minus the γ-loop) could be observed and assigned (117 residues), 67% of the N-terminal β-barrel and 37% of the C-terminal β-barrel. Regions of importance that are in dynamic equilibrium/flexible include: the N terminus (17–28), exosite I (31–33, 38–43, 68, 70–77a, and 80), His57 and Asp102 of the catalytic triad (57–59, 97a–102), the contiguous loop from 138–200 (with the exception of 179–181), and the Na+-binding loop (210, 214–228). Although we do not observe the amine NH cross peak of the N-terminal Ile16 in our spectra, the failure to observe resonances immediately following Ile16 and those which make up the activation pocket suggest that Ile16 is not stably incorporated, as would be expected of a fully activated serine protease. Apo thrombin can thus be considered zymogen-like in a structural sense, and its conversion to a more stable state through ligation could be the basis of its increased activity upon binding to Na+ or hirugen, similar to the activation of fVIIa through TF binding. Interestingly, the TROSY spectrum of the constitutively slow thrombin variant E217K was indistinguishable from that obtained in the absence of Na+ (in either Li+ or Ch+, Fig. S4), indicating that the “slow” variants do not stabilize a single “inactive” conformation as has been proposed (denoted as E* (7)), but rather maintain the dynamic state of thrombin in the presence of Na+. Consistent with this interpretation, tight-binding exosite I ligands such as TM are still capable of converting these variants into a catalytically competent state. This disordered-to-ordered structural transition involving approximately 50 residues explains the high negative heat capacity associated with thrombin ligation (24), and is consistent with previous studies using hydrogen/deuterium exchange to monitor the effect of ligation on thrombin structure (25, 26). In contrast, NMR studies on the analogous unregulated serine protease trypsin revealed no conformational or dynamic differences upon binding to active-site inhibitors (27). These results suggest that cofactor regulation of thrombin, other proteases, and perhaps enzymes in general relies on a dynamic apo state. Inherent flexibility is consistent with thrombin’s function as a regulated multispecific protease, since it allows for allostery and induced-fit binding interactions. It is interesting to note that the assigned regions of apo thrombin, thus those of defined and stable conformation, are in direct contact with the light chain (Fig. S3). The function of the light chain has not been determined, however, our data suggest that it plays a ligand-like role of stabilizing and preserving the overall fold of thrombin in the absence of other ligands.
PPACK.
PPACK binding results in the ordering of all residues with missing resonances in apo thrombin, save for a significant fraction of exosite I (Fig. 4H). Several of the unassigned residues are crucially involved in TM and fibrinogen binding, namely Gln38, Ser72, Thr74, Arg75, Tyr76, and Arg77a, suggesting that binding to exosite I necessarily involves an induced-fit component. Binding of the hirugen peptide in the context of PPACK-inhibited thrombin results in the ordering of exosite I, and shifts resonances in other parts of the N-terminal β-barrel (Fig. 4A and Table S2). In contrast, the C-terminal β-barrel appears to be entirely insensitive to the binding of hirugen, with the exception of the stabilization of C-terminal stock of the γ-loop. This observation explains why the crystal structure of thrombin bound to TM in the presence of active-site inhibitor (EGRCK) showed no conformational differences when compared to active-site inhibited thrombin (18). PPACK converts the very flexible and dynamic apo thrombin into a rigid and stable enzyme. It is likely that the docking of the Arg side chain into the S1 pocket is the crucial stabilizing interaction, since it inserts into the C-terminal β-barrel and directly contacts several residues from the 180s loop and the Na+-binding loop. As a consequence, the addition of Na+ to PPACKed thrombin has no additional effect on thrombin conformation or dynamics, and only residues in direct contact with Na+-produced resonance shifts of any significance (Fig. 4C and Table S2). In the presence of PPACK, and presumably other active-site-binding ligands, substrates or inhibitors, thrombin is locked in an active (fast) conformation, as inferred recently from spectroscopic studies (28).
Hirugen.
The effect of hirugen binding to apo thrombin is remarkable and global, with 86-residues transitioning from a state that can adopt multiple conformations to one sufficiently ordered to obtain and assign TROSY cross peaks. As expected, hirugen binding orders exosite I and other segments in the N-terminal β-barrel (Fig. 4G). However, the most dramatic effect of exosite I occupancy is the stabilization of the C-terminal β-barrel (partial or full ordering of five of the six strands), including the N terminus (18–24), the 170s loop and helix (163–178), the 180s loop (182–189), and the N- and C-terminal stocks of the γ-loop (138–146 and 154–157). However, only part of the Na+-binding loop is ordered by exosite I binding, including residues 213–215 and 226–228. In contrast to PPACK binding, the effect of hirugen on the C-terminal β-barrel domain is entirely allosteric, and plotting the residues that are sensitive to hirugen binding on the structure of thrombin (Fig. 4G) provides a unique opportunity to address the conduit for transmission of the conformational change from the binding site on exosite I to the remote C-terminal β-barrel domain. One of the main conserved features of exosite I ligand binding is the burying of a hydrophobic residue (Phe56 for hirugen, Ile414 in TM, and Phe35 of the A chain of fibrinogen (29)) between the 70s loop and the 37 loop. Our data suggest that this is the main event responsible for the stabilization of the C-terminal β-barrel. The side chain of Phe56 of hirugen inserts into a hydrophobic cluster that is dynamic in the absence of ligation. The cluster, composed of Met32, Phe34, Leu40, Arg73, Thr74, and Trp141, links all of the regions stabilized by hirugen binding (Fig. S5). For instance, the 70s loop is in direct contact with the C-terminal stock of the γ-loop, which interacts with the N terminus of the heavy chain. The two strands that form the pocket for the side chain of Ile16 are flexible in apo thrombin, and are in contact with the 70s loop and Trp141. It is clear from Fig. 4G that the red regions (those destabilized by removal of hirugen, or stabilized by its binding) are all connected, either through space (H-bonds, side chain interaction, etc) or through disulfide bonds. An example of transmission through a disulfide bond is provided by Phe56 of hirugen stabilizing the strand containing Leu40 and Cys42, which is disulfide bonded to Cys58, which in turn stabilizes the catalytic residue His57. Through-space destabilization/stabilization can be seen for both β-barrel domains, but is most evident in the C-terminal domain (Fig. 4G). It is possible to conclude that in a β-barrel protein, destabilizing one strand has an effect on the adjacent strands. This “domino effect” is a simple and effective way of transferring binding information throughout the molecule.
It is interesting to note that the Na+-binding loop is not stabilized by hirugen binding. This observation is in striking contrast to PPACK which orders the Na+ site, so that further binding of Na+ has no discernable effect on the structure/dynamics of thrombin (Fig. 4C). Na+ binding to hirugen-bound thrombin allows the assignment of 19 more resonances, including residue 17 in the N terminus, 147 at the stock of the γ-loop, residues 190–191 in the active-site cleft, and 216–225 which form the Na+-binding loop. These changes are all local to Na+ as seen in Fig. 4E, but affect critical regions of thrombin, including the insertion of the N terminus, the S1 pocket, and the disulfide bond linking the Na+-binding site to the active-site cleft (Cys220–Cys191). It is thus clear that exosite I binding is insufficient to fully stabilize the active conformation of thrombin, and that in the absence of active-site interactions, Na+ binding is also required. This observation nicely explains the previously demonstrated thermodynamic linkage between exosite I and the Na+-binding site, and has important implications for thrombin function and the physiological role of Na+ binding (discussed later).
Na+.
The effect of sodium ion binding on thrombin conformation has been under investigation for the last 30 years. Several X-ray structures of thrombin without Na+ or of mutants deficient in Na+ binding have been published, however, the structures sometimes revealed large scale changes or disorder, and other times no significant difference when compared to fast thrombin. A recent analysis of these structures (22) revealed that the regions that change conformation or dynamics correspond to the zymogen activation domain, suggesting that Na+ binding following cleavage of the activation peptide might serve to promote the zymogen-to-enzyme transformation (8). In keeping with this hypothesis, the effect of Na+ binding on apo thrombin is remarkably similar to that of hirugen binding (Fig. 4I vs. Fig. 4G), although not as extensive or far reaching. There is a general ordering of the C-terminal β-barrel, ordering of the N terminus, the 170s loop and helix, and the 180s loop. The stalks of the γ-loop do not respond to Na+ binding, indicating that this may be a specific mode of communicating from exosite I to the C-terminal β-barrel domain. It is somewhat surprising to see that the Na+-binding loop itself is not stabilized in the presence of saturating Na+ concentrations. However, this observation is consistent with the small effect of Na+ on the kinetics of substrate cleavage (20), and rapid kinetics data demonstrating an equilibrium on the μs time-scale (30).
Implications for Thrombin Allostery in Coagulation.
The current hypothesis is that conversion of prothrombin to thrombin creates the active site, exosite I, and the Na+-binding site (28), however, our results show that these sites remain in a partially disordered, dynamic state. Exosite I binding is directed by electrostatics (31), and therefore does not require a rigid/preformed conformation, and subsequent hydrophobic interactions occur via an induced-fit mechanism that stabilizes exosite I and several other regions (including a significant portion of the C-terminal β-barrel). The occupancy of exosite I improves the affinity of thrombin for Na+ by helping to form the coordination site (32), and when Na+ binds, the S1 pocket and active site are stabilized to potentially improve both Km and kcat. It is likely that most thrombin substrates, importantly fibrinogen, PAR1, and protein C, will engage exosite I first (for protein C this is through TM binding). Na+ is in rapid equilibrium, so that as soon as exosite I is engaged it will bind stably to fully form the active site. This two-step allosteric mechanism does not depend on the affinity of apo thrombin for Na+, since in the absence of exosite I binding Na+ saturation does not fully order the catalytic site, and since exosite I occupancy significantly increases affinity for Na+.
Materials and Methods
Materials.
Thrombin was prepared for NMR by a modification of the previously published method (33), using M9 minimal media made with 15N ammonium chloride as the only nitrogen source, deuterated glucose or 13C deuterated glucose as the only carbon source, and D2O (Cambridge Isotope Laboratories Inc, or Cortecnet). All salts for M9 media were dissolved in D2O and lyophilized before usage to ensure complete deuteration. The samples were at least 95% deuterated, as determined by comparing side chain to main chain signals in a one-dimensional 1H experiment with deuterated and nondeuterated samples. The C-terminal hirudin peptide (residues 54–65; hirugen) was purchased from Sigma (H6769), and dissolved in water. Protein was added to the peptide to achieve a 1.1 molar excess of hirugen. Inhibition of WT thrombin was achieved by incubation with 5-fold molar excess of PPACK (Calbiochem) at room-temperature for 30 min, and confirmed with an activity assay using the chromogenic substrate S2238 (Chromogenix).
NMR Experiments and Analysis.
All proteins used for NMR experiments originated from refolded material, which ensured complete deuterium (from growing medium) to hydrogen exchange (back exchange). WT PPACK-inhibited thrombin was dialyzed into 50 mM Na-phosphate, pH 7.4, and 0.02% NaN3 with the addition of 5% w/w D2O to the final dialysis buffer. S195A and E217K samples were prepared in 20 mM Na-phosphate, pH 7.4, 100 mM NaCl, 5% D2O, and 0.02% NaN3. All experiments were performed at the same pH to exclude effects of different solvent exchange rates. Li+ and Ch+ samples were prepared with Li-phosphate, LiOH, LiN3, and LiCl or ChCl, respectively. All NMR samples contained thrombin at a concentration of approximately 100 μM. Relaxation experiments and line-width analysis were performed to confirm the nonaggregated monodisperse nature of all samples.
NMR spectra were acquired on in-house Bruker Avance 2+ 700 and 800 MHz spectrometers, and on a Bruker Avance 900 MHz spectrometer located at the European Large Scale NMR facility in Utrecht, The Netherlands. All systems were equipped with TCI triple-resonance cryoprobes and single-axis gradients. Samples were prepared with a volume of 350 μL in microtubes (Shigemi), degassed, and stored under argon. PPACK-inhibited WT thrombin was recorded at 310K (37°C), and S195A mutants were recorded at 298 K (25
°C), due to reduced sample stability. To exclude that differences in NMR spectra were due to temperature effects, two-dimensional 1H-15N-TROSY spectra of WT-PPACKed thrombin in Na+ were measured in 5 K steps in a range from 310 K–298 K, in order to track resonance assignments in this temperature range. No significant attenuation of line broadening of resonances was observed in this temperature range. All spectra were processed with Topspin 2.1 (Bruker, Karlsruhe) and analyzed in Sparky (Goddard and Kneller, SPARKY 3, University of California, San Francisco). Backbone resonance assignments were obtained from TROSY triple-resonance experiments (34), namely HNCA, HNCACB, and HN(CO)CACB for fully liganded thrombin based on in-house scripts and the software MAPPER (35). Additional HN(CO)CA, HNCO, and HN(CA)CO experiments were performed for the PPACKed-WT thrombin in Na+, S195A in Na+, and E217K in Na+. The sequence assignment for the fully liganded state was confirmed with a HN(CA)NNH experiment, which provides an independent cross check via 15N-15N backbone connectivities. Assignments of all other states were based on these assignments and independently confirmed with TROSY HNCA experiments. TROSY spectra in ChCl and LiCl solutions were identical, as shown in Fig. S4A, and so LiCl was used to compensate for ionic strength under Na+-free conditions. The TROSY spectrum of the S195A/E217K variant was indistinguishable from that of S195A thrombin in Li+ or Ch+, as shown in Fig. S4B, and due to the high level of expression and sample stability, the E217K variant was used for three-dimensional experiments to assign apo thrombin. Weighted chemical shift differences were calculated as |Δδ(1H)| + |Δδ(15N)|/5 (36).
Acknowledgments.
The authors thank Peter Gettins for helpful early discussions, and Trevor Rutherford for some of the in-house scripts and help with some of the NMR experiments. We thank Rolf Boelens, Rainer Wechselberger and Hans Wienk for insightful discussions. Funding for this project was provided by the Medical Research Council (United Kingdom) and the National Institutes of Health. B.C.L. is supported by a British Heart Foundation studentship. Access to the European NMR Large Scale Facility in Utrecht, The Netherlands, is acknowledged.
Footnotes
The authors declare no conflict of interest.
This article is a PNAS Direct Submission.
Data deposition: The sequences reported in this paper have been deposited in the BioMagResBank, www.bmrb.wisc.edu (accession no. 16940).
This article contains supporting information online at www.pnas.org/lookup/suppl/10.1073/pnas.1005255107/-/DCSupplemental.
References
Articles from Proceedings of the National Academy of Sciences of the United States of America are provided here courtesy of National Academy of Sciences
Full text links
Read article at publisher's site: https://doi.org/10.1073/pnas.1005255107
Read article for free, from open access legal sources, via Unpaywall:
https://www.pnas.org/content/pnas/107/32/14087.full.pdf
Free after 6 months at www.pnas.org
http://www.pnas.org/cgi/content/abstract/107/32/14087
Free after 6 months at www.pnas.org
http://www.pnas.org/cgi/reprint/107/32/14087.pdf
Free after 6 months at www.pnas.org
http://www.pnas.org/cgi/content/full/107/32/14087
Citations & impact
Impact metrics
Article citations
The Prothrombin-Prothrombinase Interaction.
Subcell Biochem, 104:409-423, 01 Jan 2024
Cited by: 0 articles | PMID: 38963494
Review
From haemadin to haemanorm: Synthesis and characterization of full-length haemadin from the leech Haemadipsa sylvestris and of a novel bivalent, highly potent thrombin inhibitor (haemanorm).
Protein Sci, 32(12):e4825, 01 Dec 2023
Cited by: 3 articles | PMID: 37924304 | PMCID: PMC10683372
Dynamic allostery in thrombin-a review.
Front Mol Biosci, 10:1200465, 29 Jun 2023
Cited by: 1 article | PMID: 37457835
Review
A systematic approach for evaluating the role of surface-exposed loops in trypsin-like serine proteases applied to the 170 loop in coagulation factor VIIa.
Sci Rep, 12(1):3747, 08 Mar 2022
Cited by: 2 articles | PMID: 35260627 | PMCID: PMC8904457
Serine protease dynamics revealed by NMR analysis of the thrombin-thrombomodulin complex.
Sci Rep, 11(1):9354, 30 Apr 2021
Cited by: 6 articles | PMID: 33931701 | PMCID: PMC8087772
Go to all (72) article citations
Data
Data behind the article
This data has been text mined from the article, or deposited into data resources.
BioStudies: supplemental material and supporting data
Similar Articles
To arrive at the top five similar articles we use a word-weighted algorithm to compare words from the Title and Abstract of each citation.
19F NMR reveals the conformational properties of free thrombin and its zymogen precursor prethrombin-2.
J Biol Chem, 295(24):8227-8235, 01 May 2020
Cited by: 8 articles | PMID: 32358061 | PMCID: PMC7294081
The effects of exosite occupancy on the substrate specificity of thrombin.
Arch Biochem Biophys, 489(1-2):48-54, 26 Jul 2009
Cited by: 10 articles | PMID: 19638274
Serine protease dynamics revealed by NMR analysis of the thrombin-thrombomodulin complex.
Sci Rep, 11(1):9354, 30 Apr 2021
Cited by: 6 articles | PMID: 33931701 | PMCID: PMC8087772
Thrombin plasticity.
Biochim Biophys Acta, 1824(1):246-252, 18 Jul 2011
Cited by: 30 articles | PMID: 21782041
Review
Funding
Funders who supported this work.
British Heart Foundation (1)
NMR studies on thrombin allostery and interactions
Mr Lechtenberg
Grant ID: FS/09/012/26696
Medical Research Council (1)
Structural Basis of Molecular Recognition in Haemostasis
Professor James Huntington, University of Cambridge
Grant ID: G0601596