Abstract
Free full text

High genetic compatibility and increased pathogenicity of reassortants derived from avian H9N2 and pandemic H1N1/2009 influenza viruses
Abstract
H9N2 influenza viruses have been circulating worldwide in multiple avian species and repeatedly infecting mammals, including pigs and humans, posing a significant threat to public health. The coexistence of H9N2 and pandemic influenza H1N1/2009 viruses in pigs and humans provides an opportunity for these viruses to reassort. To evaluate the potential public risk of the reassortant viruses derived from these viruses, we used reverse genetics to generate 127 H9 reassortants derived from an avian H9N2 and a pandemic H1N1 virus, and evaluated their compatibility, replication ability, and virulence in mice. These hybrid viruses showed high genetic compatibility and more than half replicated to a high titer in vitro. In vivo studies of 73 of 127 reassortants revealed that all viruses were able to infect mice without prior adaptation and 8 reassortants exhibited higher pathogenicity than both parental viruses. All reassortants with higher virulence than parental viruses contained the PA gene from the 2009 pandemic virus, revealing the important role of the PA gene from the H1N1/2009 virus in generating a reassortant virus with high public health risk. Analyses of the polymerase activity of the 16 ribonucleoprotein combinations in vitro suggested that the PA of H1N1/2009 origin also enhanced polymerase activity. Our results indicate that some avian H9-pandemic reassortants could emerge with a potentially higher threat for humans and also highlight the importance of monitoring the H9-pandemic reassortant viruses that may arise, especially those that possess the PA gene of H1N1/2009 origin.
H9N2 influenza viruses circulate worldwide and are endemic in multiple terrestrial avian species in Asia (1–4). It is noteworthy that H9N2 influenza viruses in poultry have occasionally been transmitted to mammalian species, including humans and pigs (5–9). Human H9N2 infections produce a typical human flu-like illness that can easily be overlooked (6, 10), so they may have a greater opportunity to adapt to humans and acquire the ability of human-to-human transmission. In fact, several serological surveys revealed that a large number of people in China, ranging from 13.7% to 37.2%, might have evidence of prior infections of the H9N2 virus (11, 12). In addition, previous studies demonstrated that a significant proportion of H9N2 field isolates have acquired preference for a human virus-like receptor (10, 13). Thus, H9N2 influenza virus, along with H5N1 virus, is high on the list of candidates that could potentially cause another human influenza pandemic.
Pandemic H1N1/2009 influenza virus has spread by human-to-human transmission across the globe at an unprecedented rate since it was first isolated from humans in Mexico in 2009 (14, 15, 16). Recently, it has been announced by the World Health Organization (WHO) that the pandemic H1N1 influenza virus is now in the postpandemic period but is expected to become a recurrent seasonal influenza virus and circulate for some years (WHO; http://www.who.int/csr/disease/swineflu/en/). Additionally, pandemic H1N1/2009 influenza viruses were also frequently isolated from pigs (17–19) that were proposed to be “mixing vessels” for the reassortment of influenza viruses.
Coinfection with H9N2 and pandemic H1N1/2009 influenza viruses in the same host (e.g., pigs and humans) provides the opportunity for reassortment between these viruses. Reassortment is an important mechanism for the generation of a pandemic influenza strain (20, 21). For example, the pandemic influenza viruses of 1957 and 1968 emerged through genetic reassortment of avian viruses with the prevailing human viruses to possess novel antigenicity and efficient human-to-human transmissibility (22, 23). These facts remind us that avian H9N2 influenza viruses could acquire some functions critical for pandemic strains by reassortment with pandemic H1N1 influenza viruses when infecting the same host. Furthermore, previous studies revealed that frequent reassortment is an important evolution mechanism of H9N2 influenza viruses (4, 5) and that the pandemic H1N1/2009 influenza virus had already reassorted with the H1N1 swine influenza virus (24). Therefore, the concern is that if H9N2 influenza virus reassorts with a pandemic (H1N1) virus, another pandemic strain will emerge.
Reverse genetics provides a tool to predict the potential public health risk of novel influenza viruses. Using such an approach, previous studies generated various avian H5N1–human H3N2 reassortant viruses to evaluate their biological properties (25, 26). In the current study, we generated a panel of reassortants derived from contemporary avian H9N2 and pandemic H1N1/2009 viruses by reverse genetics to study their genetic compatibility and biological characteristics. Theoretically, 254 (256 minus 2 parental viruses) genotypes of reassortants can be generated from two distinct influenza A viruses. In the present study, we attempted to generate all of the 127 (128 minus 1 H9N2 parental virus) reassortants derived from an avian H9N2 influenza virus (A/chicken/Hebei/LC/2008, HB08) and a pandemic H1N1 influenza virus (A/Beijing/16/2009, BJ09). These reassortants possessed an HA gene from the avian H9N2 influenza virus and the other seven genes from either parental virus. Our in vitro and in vivo analyses indicated that these two influenza viruses possessed high genetic compatibility and some reassortants showed enhanced pathogenicity in mice compared with parental viruses. Therefore, the possibility of novel pandemic strains being generated from reassortment between avian H9N2 and H1N1/2009 influenza viruses exists.
Results
Replication Abilities of the Reassortant Viruses Between HB08 and BJ09 in Vitro.
To investigate the properties of H9 subtype avian–pandemic reassortant viruses, we first established an eight-plasmid reverse genetics (rg) system of the two parental viruses, avian H9N2 influenza virus (HB08) and 2009 pandemic H1N1 influenza virus (BJ09). Transfections of all of the 127 combinations of plasmids between these two parental viruses with the HA gene from avian H9N2 virus were performed. At 72 h posttransfection, culture supernatant was inoculated into 10-d-old specific-pathogen-free (SPF) embryonated chicken eggs to produce virus stocks. The titer of each virus was determined by plaque assay on Madin–Darby canine kidney (MDCK) cells. The rgHB08 and rgBJ09 viruses replicated efficiently in vitro with titers of 8.9 log10 pfu/mL and 7.5 log10 pfu/mL, respectively. Given the titers achieved for each reassortant, the 127 reassortants could be categorized into four groups:
i) Reassortants with a high replication ability (replication group I). A total of 73 reassortants (~58% of the 127 reassortants) possessed replication ability comparable to that of HB08 and BJ09 viruses with titers ≥7.0 log10 pfu/mL (Table S1).
ii) Reassortants with a moderate replication ability (replication group II). This group contained 36 reassortants (~28% of reassortants) with titers ranging from 4 to 6 log10 pfu/mL (Table S2).
iii) Reassortants with a low replication ability (replication group III). Five influenza viruses (~4% of reassortants) grew poorly in vitro with titers between 1 and 3 log10 pfu/mL, suggesting that the replication of these viruses was impaired (Table S3).
iv) Nonviable reassortants (replication group IV). A total of 13 reassortants (~10% of reassortants) had severe replication defects characterized by the absence of any observable cytopathic effects (CPE) or visible plaques in MDCK cells (Table S4).
To determine the effect of a single genomic segment on virus replication in vitro, two viruses with only one gene segment from different origins were paired and analyzed by the paired-samples t test. Statistical analyses suggested that the introduction of BJ09 PB2 (P < 0.01) or NS (P < 0.05) could enhance the replication of reassortants, and the introduction of HB08 PB1 (P < 0.01), NP (P < 0.01), NA (P < 0.01), or M (P < 0.01) usually enhanced reassortant virus replication (Table S5). No significant difference in viral titers was observed between the pairs of viruses with HB08 PA or BJ09 PA in the same genetic background (P > 0.05).
The interaction between two segments of all combinations was also analyzed (Table S6). We noted that some gene segments could coordinate better with heterologous genes than with homologous genes. For example, BJ09 PB2 usually enhanced the replication of the reassortants with HB08 M (P < 0.05) in the same genetic background; and BJ09 NS increased the replication ability of the reassortants with HB08 PB2 (P < 0.05) or NA (P < 0.01). HB08 PB1 enhanced the reassortants with BJ09 PB2 in the same genetic background (P < 0.01); HB08 NP enhanced the reassortants with BJ09 PB1 (P < 0.01), PA (P < 0.01), NA (P < 0.01), or NS (P < 0.01); HB08 NA enhanced the reassortants with BJ09 PB2 (P < 0.01), PA (P < 0.01), NP (P < 0.01), M (P < 0.05), or NS (P < 0.01); and HB08 M enhanced the reassortants with BJ09 NA (P < 0.01).
Taken together, our data show that the gene segments from avian H9N2 and pandemic H1N1 viruses could readily coexist and functionally coordinate, indicating that these two viruses had high genetic compatibility.
Pathogenicity of the Reassortants in Mice.
The 50% mouse infectious dose (MID50), 50% mouse lethal dose (LD50), and viral replication in tissues, including lung, nasal turbinate, brain, liver, spleen, kidney, and colon, of two parental viruses in BALB/c mice were evaluated. Both of the parental viruses readily infected mice (MID50 < 101 pfu), and the LD50 of HB08 and BJ09 viruses was 105.5 and 104.8 pfu, respectively. These viruses replicated efficiently in the lung and nasal turbinate (>107 pfu/mL) on day 4 following an intranasal inoculation of 104 pfu (Table S7), whereas no virus was detected in other tissues. With the 105 pfu inoculation dose, HB08 virus caused 8.1% weight loss and did not cause any deaths, and BJ09 virus caused 15.1% weight loss with one mouse death.
We next evaluated the pathogenicity in mice of all 73 reassortants in replication group I in vitro (with titer ≥7.0 log10 pfu/mL). All tested reassortants readily infected the mice (MID50 < 101.5 pfu) and could replicate in the nasal turbinate (Table S7), but were not detected in the other tissues. These reassortants were sorted into three groups according to their pathogenicity in mice:
i) Reassortants of higher pathogenicity than both parental viruses (pathogenicity group I). A total of 8 reassortants (~11% of the 73 tested reassortants) with LD50 102.8 to 104.5 pfu belonged to this group (Table 1). The mean survival time (MST) following infection with 105 pfu virus was 5–6.7 d. Obvious clinical signs, such as ruffled coat, lethargy, and dyspnea could be observed. Hematoxylin and eosin (H&E) staining showed that viruses of this group caused severe interstitial pneumonia and bronchopneumonia, characterized by edema, hemorrhages, serious dropout of epithelial cells, and extensive infiltration of inflammatory cells including lymphocytes, neutrophils, and plasma cells (Fig. 1 A and B). To determine if there was any mutation contributing to the high-pathogenicity phenotype, the viruses of this group were fully sequenced. No mutations were found, indicating that the high-pathogenicity phenotype is an intrinsic property of these reassortants.
Table 1.
Reassortants of higher pathogenicity than both parental viruses (pathogenicity group I)
“r” in virus names denotes reassortant. The numbers in the virus names indicate gene segments derived from the BJ09 virus as follows: 1, PB2; 2, PB1; 3, PA; 4, HA; 5, NP; 6, NA; 7, M; and 8, NS. The virus segments derived from the HB08 virus were not assigned numbers. Segments derived from BJ09 virus are in yellow, and those derived from HB08 virus are in blue. The pathogenicity of the reassortant viruses was determined as described in Materials and Methods. Maximum mean weight loss was determined from three mice (percentage weight loss relative to day 0 p.i.) following infection with 105 pfu of virus. MST, mean survival time of mice infected with 105 pfu.
Representative histopathological changes in H&E-stained lung tissues. (A) r3/5/6/8 virus and (B) r3/6/7/8 virus showing severe bronchopneumonia and interstitial pneumonia; edema and bronchial epithelial cell desquamation (thick solid arrow); massive immune cell infiltrates around bronchi and blood vessels including lymphocytes, neutrophils, and plasma cells (thick white arrow); alveolar wall thickening (▲); and alveolar epithelial cells, erythrocytes, and inflammatory cells within alveolar spaces (
). (C) WTBJ09 virus and (D) r1/2/3/5/6/8 virus showing moderate bronchopneumonia; edema, severe desquamation, and lesion of bronchial epithelial cells (thick solid arrow), and presence of inflammatory cells around bronchi and blood vessels (thick open arrow). (E) WTHB08 virus. Mild bronchopneumonia. Mild desquamation of bronchial epithelial cells (thick solid arrow). (F) r1 virus. Except for congestion, no obvious lesions. (Scale bar: 50 μm.)
ii) Reassortants with pathogenicity similar to that of the BJ09 virus (pathogenicity group II). A total of 11 reassortants (~15% of the tested reassortants) with pathogenicity comparable to wild-type BJ09 virus were tested in vivo. Typically, one to three of the four mice inoculated died after infection with 104 pfu of these viruses. The LD50 was determined to be between 104.8 and 105.3 pfu and the MST 7.7–12.3 d (Table 2). These viruses caused moderate bronchopneumonia similar to both parental viruses (Fig. 1 C and D).
Table 2.
Reassortants with pathogenicity similar to BJ09 virus (pathogenicity group II)
Symbols and nomenclature of the viruses are as described in the legend in Table 1.
iii) Reassortants with pathogenicity similar to or lower than that of the HB08 virus (pathogenicity group III). This group contained eight reassortants (~11% of the tested reassortants). Similar to wild-type HB08 virus, they did not cause any death, but resulted in >5% body weight loss at a 105-pfu inoculation dose (Table 3). Mild to moderate bronchopneumonia was observed in the H&E-stained lung tissues of mice inoculated with these viruses (Fig. 1E).
Table 3.
Reassortants with pathogenicity similar to HB08 virus (pathogenicity group III)
Symbols and nomenclature of the viruses are as described in the legend in Table 1. None of these viruses killed mice at 105 pfu. Therefore, the LD50 was assigned as ≥105.5 pfu and the MST was >14 d.
iv) Reassortants of lower pathogenicity than that of parental viruses (pathogenicity group IV). A total of 46 reassortants (~63% of the tested reassortants) belonged to this group (Table 4). All mice inoculated with viruses of this group survived with no obvious weight loss or other clinical signs. H&E staining showed that lesions were mild or absent in the lung tissues of mice infected with this group of viruses (Fig. 1F).
Table 4.
Reassortants of lower pathogenicity than parental viruses (pathogenicity group IV)
Symbols and nomenclature of the viruses are as described in the legend in Table 1. None of these viruses killed mice at 105 pfu. Therefore, the LD50 was assigned as ≥105.5 pfu and the MST was >14 d.
It is noteworthy that all eight reassortant viruses belonging to pathogenicity group I possessed the PA gene derived from the pandemic BJ09 virus, indicating that BJ09 PA is a prerequisite for the emergence of highly virulent reassortant viruses. To evaluate the contributions to pathogenicity made by the H1N1/2009 virus genes of the reassortant viruses, we analyzed pathogenicity data of single-gene reassortant viruses, each containing one gene segment from the H1N1/2009 virus. We found that only reassortants with BJ09 PA (r3 virus in Table 1) had much higher pathogenicity compared with parental viruses. The single reassortant with BJ09 NA (r6 virus in Table 2) exhibited similar pathogenicity to those of the HB08 virus, whereas the pathogenicity of the other five single reassortants (r1, r2, r5, r7, and r8 viruses in Table 4) was lower than the parental viruses. Analysis of the paired viruses with or without BJ09 PA in the same genetic background included a total of 24 pairs of viruses. Among these viruses, 11 reassortants possessing BJ09 PA had higher pathogenicity than the corresponding viruses with a PA gene derived from the HB08 virus, whereas only two viruses with BJ09 PA had lower pathogenicity than the corresponding reassortants without BJ09 PA (Table S8). The other 11 pairs of viruses had no pathogenic difference with or without the BJ09 PA gene. These results emphasize the important effect of BJ09 PA on the pathogenicity of reassortants in mice.
By comparing the 24 pairs of viruses with or without BJ09 PB1 in the same genetic background, we found that 12 viruses with BJ09 PB1 had increased pathogenicity after introducing HB08 PB1, and only one had reduced pathogenicity (Table S9). The other 11 pairs of viruses had no pathogenic difference with or without the BJ09 PB1 gene. These results suggested that the BJ09 PB1 gene usually attenuated the pathogenicity of reassortants in mice. By analyzing other segments in the same way, we found that BJ09 NA typically increased the pathogenicity of reassortants in mice (Table S10), whereas the influence of BJ09 PB2, NP, M, or NS was not obvious.
Taken together, the pathogenicity analyses indicated that the PA gene of H1N1/2009 virus origin is indispensable for reassortant viruses to be more virulent in mice. The NA gene from the pandemic H1N1/2009 virus also typically increased the pathogenicity of reassortant viruses in mice. In contrast, the PB1 gene from H1N1/2009 virus attenuated viruses. Other pandemic virus gene segments, including the PB2, NP, M, and NS genes, did not significantly influence the pathogenicity of reassortant viruses.
In Vitro Viral Polymerase Activity.
Previous studies demonstrated that the viral ribonucleoprotein (RNP) complex had an important correlation with viral generation, replication, and pathogenicity (27, 28). To study the mechanisms underlying the differences in the replication and pathogenicity phenotypes of the H9-pandemic reassortants, the activity of 16 RNP combinations of PB2, PB1, PA, and NP from either HB08 or BJ09 viruses was determined by measuring the activity of luciferase at 33 °C or 37 °C, which mimics the temperature of the upper and lower respiratory tract as previously described (25, 26). As shown in Fig. 2, the avian HB08 RNP (HPB2HPB1HPAHNP; “H” stands for HB08 virus) activity at 33 °C was ~40% lower than that at 37 °C. In contrast, the RNP (BPB2BPB1BPABNP; “B” stands for BJ09 virus) activity of pandemic BJ09 at 33 °C was comparable to that at 37 °C. All 13 viruses in replication group IV (nonviable reassortants) possessed RNP complexes with low activity (<20%) in the minigenome assay, with only two exceptions (r2/3/6/7 and r3/5/6/7 viruses), indicating that RNP activity plays an important role in the emergence and replication ability of reassortants. Although RNP activity for most reassortant viruses in the high replication group was high or moderate, some reassortants with a low RNP complex were also include in this group, suggesting that other factors could influence viral replication, such as HA, NA, M, and NS genes that were not included in this assay. The RNP constituted by PA from BJ09 virus resulted in high polymerase activities at 37 °C. For example, six of seven hybrid RNP combinations containing BJ09 PA protein showed higher activity than the BPB2BPB1BPABNP. Consistently, all of the viruses in pathogenicity group I contained the BJ09 PA gene. Furthermore, all three RNP combinations (HPB2HPB1BPAHNP, HPB2HPB1BPABNP, and BPB2BPB1BPABNP) included in pathogenicity group I also showed high activities at 33 °C.
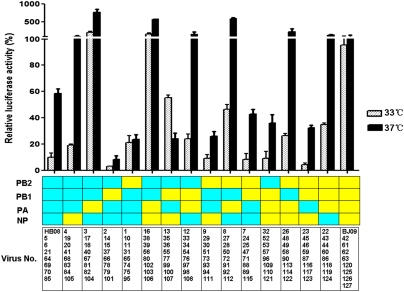
Polymerase activity of 16 RNP combinations between the HB08 and BJ09 viruses. The 293T cells were transfected in duplicate with luciferase reporter plasmid p-Luci and internal control plasmid Renilla, together with plasmids expressing PB2, PB1, PA, and NP from either HB08 or BJ09 viruses. Segments derived from the BJ09 virus are in yellow, and those derived from HB08 virus are in blue. Virus number corresponding to the RNP combination of viruses is listed. Cells were incubated at 33 °C (stippled bars) or at 37 °C (solid bars) for 24 h, and cell lysates were analyzed to measure Firefly and Renilla luciferase activities. Values shown are the mean ± SD of the three independent experiments and are standardized to those of BJ09 measured at 37 °C (100%).
Discussion
Reassortment is an important mechanism for the evolution of influenza viruses that could lead to antigenic shift and the generation of pandemic strains (16). The coexistence of pandemic H1N1/2009 influenza virus and some avian influenza viruses (such as avian H5N1 and H9N2 viruses) in humans and pigs provides an opportunity for the incorporation of avian virus genes into mammalian-adapted viruses that results in the emergence of novel viruses with considerable potential threat to public health. Several studies focused on the reassortment between highly pathogenic avian H5N1 and pandemic H1N1 viruses (29, 30). However, all previous influenza pandemic strains evolved from viruses with low pathogenicity (16). To evaluate the potential public risk of avian H9N2–pandemic H1N1 reassortants, we systematically studied the 127 combinations of reassortant viruses derived from an avian H9N2 influenza virus and a 2009 pandemic H1N1 influenza virus. All these reassortant viruses had the HA gene of H9 origin. High genetic compatibility was observed between avian H9N2 and pandemic H1N1 influenza viruses, and eight reassortants possessed enhanced virulence in mice compared with parental viruses.
Using reverse genetics, previous studies systematically generated avian H5N1–human H3N2 influenza reassortants and evaluated their genetic compatibility (25, 26). Chen et al. (26) used reverse genetics to generate 63 reassortant viruses derived from A/Thailand/16/04 (H5N1) and A/Wyoming/3/03 (H3N2) viruses, containing the H5N1 surface protein genes and one extra single-gene reassortant virus, r6, possessing the A/Wyoming/3/03 NA in the background of the A/Thailand/16/04 virus. Among the 64 reassortants, ~44% of the reassortants yielded wild-type or near-wild-type replication efficiency in MDCK cell culture, and ~20% of the reassortants were nonviable or marginally viable. Li et al. (25) attempted to generate all 254 combinations of reassortant viruses between A/chicken/South Kalimantan/UT6028/06 (H5N1) and A/Tokyo/Ut-Sk-1/07 (H3N2) influenza viruses. Among the 127 reassortants with HA genes of H5 origin, ~48% viruses grew comparable to their parental viruses and ~21% reassortants produced no visible CPE. In the present study, among the 127 H9 reassortants between avian H9N2 and 2009 pandemic H1N1 viruses, up to ~57.5% of these viruses had a high replication ability similar to their parental viruses, and only ~10.2% reassortants had no visible CPE. The higher percentage of H9 reassortants with efficient replication ability and the lower percentage of nonviable viruses compared with reassortants derived from avian H5N1 and seasonal human H3N2 influenza viruses suggested that avian H9N2 and pandemic H1N1 influenza viruses have a high genetic compatibility.
The pathogenicity of all 73 reassortants with high replication ability (replication group I) was evaluated in a BALB/c mouse model. All viruses tested readily infected mice and could be detected in nasal turbinate. Importantly, eight of these viruses possessed higher pathogenicity in mice than their parental viruses. These results indicated that the reassortment between avian H9N2 and pandemic H1N1 influenza viruses could generate reassortants that were more virulent for mammals than their parental viruses. Additionally, the other less virulent reassortants also readily infected mice, suggesting that these viruses were able to circulate in a mammalian host that could easily be ignored and evolve by adaptive mutation or further reassortment capable of posing a potential threat for humans. Thus, the present study highlights the potential risk of such reassortants derived from avian H9N2 and pandemic H1N1 viruses to public health.
Our findings demonstrated that the PA gene from the BJ09 virus may significantly contribute to the pathogenicity of avian–human reassortants. H9N2 virus that was replaced only by a single PA gene from pandemic H1N1 virus could substantially increase its pathogenicity in mice. Although the introduction of the PA gene from pandemic H1N1/2009 virus into avian H9N2 virus did not necessarily result in a more pathogenic phenotype, our results revealed that the PA gene of H1N1/2009 origin is a prerequisite for the emergence of reassortants with increased pathogenicity. It is known that the PA gene of pandemic H1N1 (2009) virus was of North American avian origin (16, 31). Thus, the pandemic PA gene may be compatible with the other gene segments from avian H9N2 viruses as a prerequisite for the increase of viral pathogenicity. Furthermore, a previous study found that the introduction of a human influenza PA gene into an avian polymerase gene could overcome restriction of avian polymerase in human cells (32). Similarly, in the present study, the PA gene of pandemic H1N1/2009 origin could increase the polymerase activity of avian-pandemic RNP, especially for the HPB2HPB1BPAHNP combination. Therefore, the PA gene of pandemic H1N1/2009 origin that was adapted to humans might contribute to the high polymerase activity leading to enhanced virulence of the reassortants in mice.
Pathogenicity studies in mice demonstrated that the effect of a single gene segment on pathogenicity was influenced by the other seven segments. For example, although the single PA gene replaced by those of 2009 pandemic virus origin in the background of H9N2 influenza virus could increase virulence in mice, not all of the H9 reassortants possessing the PA gene from the pandemic H1N1/2009 virus had a higher virulence than their parental viruses, suggesting that other gene segments influenced the effect of the pandemic-origin PA gene. Indeed, the pathogenesis of influenza virus includes polygenic virulence factors (33). Li et al. (25) reported that reassortment between two influenza viruses results in the emergence of reassortant viruses with higher pathogenicity than parental viruses. Their study evaluated the pathogenicity of 75 reassortant viruses derived from avian H5N1 and human H3N2 influenza viruses and found that 10 reassortants were more virulent than parental viruses.
Although some H9-pandemic reassortant viruses (pathogenicity group I viruses) possessed higher virulence than parental viruses, none resulted in systemic spread in mice, as observed for the highly pathogenic H5N1 influenza virus. These reassortants were restricted to the respiratory system. However, we found that all reassortants replicated efficiently in the lungs of mice and showed high polymerase activity. These results suggested that a high virus load and high polymerase activity were important factors for the virulence of the reassortants in mice. Additionally, we observed that inflammatory cell infiltration in the lungs of infected mice by pathogenicity group I viruses was significantly greater than that of less virulent reassortant viruses, suggesting that a severe inflammatory response is likely to be induced by the reassortant viruses with higher pathogenicity.
In summary, we found that the contemporary avian H9N2 influenza virus and pandemic H1N1 virus showed high genetic compatibility and could generate reassortants with higher pathogenicity than parental viruses, indicating that H9N2 influenza virus could contribute to the evolution of viruses with an increased threat for public health by reassorting with the pandemic H1N1/2009 virus. Our findings also demonstrate that the PA gene of pandemic origin plays an important role in determining the high pathogenicity of H9 reassortants and high RNP activity, which could potentially serve as an indicator for recognizing reassortants with high risk to public health.
Materials and Methods
Viruses and Cells.
A/chicken/Hebei/LC/2008 (H9N2) (HB08) virus was isolated from diseased chicken in Hebei, China, in January 2008 and propagated in 10-d-old SPF embryonated chicken eggs. The phylogenetic and genetic properties of HB08 virus have been described previously (4). A/Beijing/16/2009 (H1N1) (BJ09) virus was isolated from a patient with flu-like symptoms in Beijing, China, in November 2009 and grown in MDCK cells. Human embryonic kidney cells (293T) and MDCK cells were grown in DMEM (Invitrogen) containing 10% FBS (Invitrogen). Viral infectivity was determined by plaque assay on MDCK cells, as previously described (34).
Generation of Reassortant Viruses by Reverse Genetics.
Reverse transcription-PCR (RT-PCR) amplicons of the eight viral genes from HB08 and BJ09 viruses were cloned into a dual-promoter plasmid, PHW2000. MDCK and 293T cells were cocultured and transfected with 0.5 μg of each of the eight plasmids and 10 μL lipofectamine 2000 (Invitrogen) in a total volume of 1 mL of Opti-MEM (Invitrogen). After incubation at 37 °C for 6 h, the transfection mixture was removed from the cells and 2 mL of Opti-MEM containing 1 μg/mL of tosylphenylalanine chloromethyl ketone treated trypsin (TPCK-trpsin, Worthington Biochemical Corporation) was added. After 72 h, the supernatant was inoculated in 10-d-old SPF embryonated chicken eggs to produce stock viruses. The titers of the stock viruses were determined by plaque assay on MDCK cells. Viral RNA was extracted and analyzed by RT-PCR, and each viral segment was sequenced to confirm the identity of the virus. All experiments with live viruses and transfectants generated by reverse genetics were performed in a biosafety level 3 containment laboratory approved by the Ministry of Agriculture of the People's Republic of China.
Pathogenicity Test in Mice.
Groups of 6- to 8-wk-old female BALB/c mice (Vital River Laboratory) were anesthetized with Zoletil (tiletamine-zolazepam; Virbac; 20 μg/g) and intranasally inoculated with 50 μL of serial 10-fold dilutions of infectious virus in PBS. For reassortant viruses, 105 pfu virus was the highest dose used for inoculation. Three mice from each group were euthanized on day 4 postinfection (p.i.) and tissues, including nasal turbinate, lung, brain, liver, spleen, kidney, and colon, were harvested for plaque assay in MDCK. Additionally, a portion of each lung tissue was immersed in 10% neutral buffered formalin solution, routinely processed, and embedded in paraffin. Five-micrometer sections were stained with H&E. The remaining three mice in the 105 and 104 pfu groups were monitored daily for 14 d for weight loss and mortality. Mice that lost >20% of their body weight were euthanized. MID50 and LD50 were calculated and expressed as the pfu value corresponding to 1 MID50 or LD50.
RNP Minigenome Luciferase Assay.
Four expression plasmids of PB2, PB1, PA, and NP (50ng each) from either HB08 or BJ09 viruses were cotransfected into 293T cells together with the luciferase reporter plasmid p-Luci (10 ng) and internal control plasmid Renilla (5 ng). The assay was performed at both 33 and 37 °C. At 24 h posttransfection, cell lysate was prepared with Dual-Luciferase Reporter Assay System (Promega) and luciferase activity was measured using GloMax 96 microplate luminometer (Promega).
Statistical Analysis.
To determine the effect of a single genomic segment on virus replication in vitro, viruses with a different origin from this segment but in the same background were paired. For each gene segment, the statistical significance of a group of 64 virus pairs was determined by paired-sampled t test. If the P value (two-tailed) of the t test was <0.05, the mean titers of viruses with or without this segment were compared in order to evaluate the effect of this segment for virus replication. If P > 0.05, we concluded there was no significant effect of this segment on virus replication.
To evaluate the influence of one segment on another in virus replication, viruses with one segment and with or without another segment in the same background were paired. The statistical significance of a group of 32 virus pairs was determined by paired-sampled t test. If the P value (two-tailed) of the t test was <0.05, the mean titers of these viruses with or without another segment were compared in order to evaluate the effect of another segment in virus replication. If P > 0.05, we concluded that there was no significant effect of another segment on this segment in virus replication.
Acknowledgments
We thank Huijie Gao, Honglei Sun, and Yuan Ma for excellent technical assistance. This work was supported by the National Science Fund for Distinguished Young Scholars (31025029), the National Basic Research Program (973 Program, 2011CB504702), the National Natural Science Foundation of China (30901072), the Fundamental Research Funds for the Central Universities (2009JC02), the Specialized Research Fund for the Doctoral Program of Higher Education (20090008120007), and the Program for Cheung Kong Scholars and Innovative Research Teams in Chinese Universities (IRT0866). J.L. was funded by the Taishan Scholar Foundation.
Footnotes
The authors declare no conflict of interest.
This article is a PNAS Direct Submission.
The sequences reported in this paper have been deposited in the GenBank database [accession nos. GQ373026 (HB08, PB2 gene), GQ373043 (HB08, PB1 gene), GQ373060 (HB08, PA gene), GQ373077 (HB08, HA gene), GQ373094 (HB08, NP gene), GQ373111 (HB08, NA gene), GQ373128 (HB08, M gene), GQ373144 (HB08, NS gene), HQ698624 (BJ09, PB2 gene), HQ698625 (BJ09, PB1 gene), HQ698626 (BJ09, PA gene), HQ698627 (BJ09, HA gene), HQ698628 (BJ09, NP gene), HQ698629 (BJ09, NA gene), HQ698630 (BJ09, M gene), and (BJ09, NS gene)].
This article contains supporting information online at www.pnas.org/lookup/suppl/10.1073/pnas.1019109108/-/DCSupplemental.
References
Articles from Proceedings of the National Academy of Sciences of the United States of America are provided here courtesy of National Academy of Sciences
Full text links
Read article at publisher's site: https://doi.org/10.1073/pnas.1019109108
Read article for free, from open access legal sources, via Unpaywall:
https://www.pnas.org/content/pnas/108/10/4164.full.pdf
Free after 6 months at www.pnas.org
http://www.pnas.org/cgi/content/full/108/10/4164
Free after 6 months at www.pnas.org
http://www.pnas.org/cgi/reprint/108/10/4164.pdf
Free after 6 months at www.pnas.org
http://www.pnas.org/cgi/content/abstract/108/10/4164
Citations & impact
Impact metrics
Citations of article over time
Alternative metrics
Article citations
Effectively Evaluating a Novel Consensus Subunit Vaccine Candidate to Prevent the H9N2 Avian Influenza Virus.
Vaccines (Basel), 12(8):849, 28 Jul 2024
Cited by: 0 articles | PMID: 39203975 | PMCID: PMC11359011
The Chinese Hamster Ovary Cell-Based H9 HA Subunit Avian Influenza Vaccine Provides Complete Protection against the H9N2 Virus Challenge in Chickens.
Viruses, 16(1):163, 22 Jan 2024
Cited by: 2 articles | PMID: 38275973 | PMCID: PMC10821000
Phosphorylation of PB2 at serine 181 restricts viral replication and virulence of the highly pathogenic H5N1 avian influenza virus in mice.
Virol Sin, 39(1):97-112, 14 Dec 2023
Cited by: 0 articles | PMID: 38103645 | PMCID: PMC10877443
Mixed selling of different poultry species facilitates emergence of public-health-threating avian influenza viruses.
Emerg Microbes Infect, 12(1):2214255, 01 Dec 2023
Cited by: 4 articles | PMID: 37191631 | PMCID: PMC10215028
Increased public health threat of avian-origin H3N2 influenza virus caused by its evolution in dogs.
Elife, 12:e83470, 06 Apr 2023
Cited by: 11 articles | PMID: 37021778 | PMCID: PMC10147381
Go to all (121) article citations
Data
Data behind the article
This data has been text mined from the article, or deposited into data resources.
BioStudies: supplemental material and supporting data
Nucleotide Sequences (Showing 15 of 15)
- (1 citation) ENA - GQ373128
- (1 citation) ENA - HQ698628
- (1 citation) ENA - HQ698629
- (1 citation) ENA - GQ373094
- (1 citation) ENA - GQ373060
- (1 citation) ENA - HQ698630
- (1 citation) ENA - GQ373043
- (1 citation) ENA - GQ373077
- (1 citation) ENA - HQ698624
- (1 citation) ENA - GQ373026
- (1 citation) ENA - HQ698625
- (1 citation) ENA - GQ373111
- (1 citation) ENA - GQ373144
- (1 citation) ENA - HQ698626
- (1 citation) ENA - HQ698627
Show less
Similar Articles
To arrive at the top five similar articles we use a word-weighted algorithm to compare words from the Title and Abstract of each citation.
Differences in transmissibility and pathogenicity of reassortants between H9N2 and 2009 pandemic H1N1 influenza A viruses from humans and swine.
Arch Virol, 159(7):1743-1754, 09 Feb 2014
Cited by: 5 articles | PMID: 24510170
High frequency of reassortment after co-infection of chickens with the H4N6 and H9N2 influenza A viruses and the biological characteristics of the reassortants.
Vet Microbiol, 222:11-17, 18 Jun 2018
Cited by: 16 articles | PMID: 30080665
Genetic Compatibility of Reassortants between Avian H5N1 and H9N2 Influenza Viruses with Higher Pathogenicity in Mammals.
J Virol, 93(4):e01969-18, 05 Feb 2019
Cited by: 17 articles | PMID: 30463961 | PMCID: PMC6363993
Virulence of pandemic (H1N1) 2009 influenza A polymerase reassortant viruses.
Virulence, 2(5):422-426, 01 Sep 2011
Cited by: 4 articles | PMID: 21921678
Review