Abstract
Free full text

Expeditious oligosaccharide synthesis via selective, semi-orthogonal, and orthogonal activation
Abstract
Traditional strategies for oligosaccharide synthesis often require extensive protecting and/or leaving group manipulations between each glycosylation step, thereby increasing the total number of synthetic steps while decreasing the efficiency of the synthesis. In contrast, expeditious strategies allow for the rapid chemical synthesis of complex carbohydrates by minimizing extraneous chemical manipulations. Oligosaccharide synthesis by selective activation of one leaving group over another is one such expeditious strategy. Herein, the significant improvements that have recently emerged in the area of the selective activation are discussed. The development of orthogonal strategy further expands the scope of the selective activation methodology. Surveyed in this article, are representative examples wherein these excellent innovations have been applied to the synthesis of various oligosaccharide sequences.
1 Introduction. Chemical glycosylation and conventional oligosaccharide synthesis
Carbohydrates are the most abundant biomolecules on Earth, nevertheless, their chemistry and biology has been a relatively slowly developing field. To ensure the availability of pure complex carbohydrates, their chemical1,2 or enzymatic3,4 synthesis has become the topic of prime importance. The development of stereoselective methods and efficient strategies for the synthesis of complex carbohydrates has become critical to the emerging areas of glycosciences. The monomeric units in oligosaccharides are connected via O-glycosidic linkages. The glycosidic linkage is obtained by means of the glycosylation reaction that is commonly based on the nucleophilic displacement of the anomeric leaving group (LG) of the glycosyl donor with a hydroxyl moiety of the glycosyl acceptor (Scheme 1). The remaining hydroxyls of both units are typically masked with temporary protecting groups (P). The achievement of high yields and complete stereocontrol is difficult due to the complexity of the glycosylation process that often proceeds along with a variety of side reactions.5,6 However, a single-step glycosylation is not the only challenge researchers working on oligosaccharide synthesis face. Traditional linear approaches for the synthesis of oligosaccharides imply conversion of the disaccharide into second-generation glycosyl donors or acceptors. This transformation typically involves protecting group manipulations or leaving group installation between each glycosylation step (Scheme 1). Such additional manipulations increase the number of total synthetic steps and decrease the efficiency of the oligosaccharide assembly as reflected in dramatic drop in yield. This called for revisiting existing linear strategies for oligosaccharide synthesis and some significant improvements have already emerged.7
2. Expeditious oligosaccharide synthesis: concepts of selective and chemoselective activations
Recent strategies for oligosaccharide synthesis are typically based on the direct multi-step activation of a series of building blocks.7,8 These expeditious approaches significantly shorten oligosaccharide assembly by minimizing the need for protecting group manipulations between glycosylation steps. Nicolaou’s selective activation,9 Fraser-Reid’s armed-disarmed approach,10 Danishefsky’s glycal assembly,11 Ogawa’s orthogonal technique,12,13 Roy’s14 and Boons’15 active-latent concept, Wong’s and Ley’s programmable strategies,16–19 are only few such strategies to mention. One of the most conceptually attractive and practically efficient procedures, Fraser-Reid’s armed-disarmed approach, is based on the principle of chemoselectivity. According to this principle, a benzylated (electronically activated, armed) glycosyl donor is chemoselectively activated over the acylated (electronically deactivated, disarmed) glycosyl acceptor bearing the same leaving group (Scheme 2).20,21
The availability of a suitable mild promoter is essential to effectively differentiate the reactivity levels of the armed and disarmed species. At this stage, a 1,2-cis-linked disaccharide is preferentially obtained due to the general use of the ether-type arming substituent, a non-participating group. The obtained disaccharide can then be used for 1,2-trans glycosylation directly in the presence of a more potent promoter that can activate the disarmed leaving group. Initially developed for O-pentenyl glycosides,21,22 this approach works with many other classes of stable glycosyl donors.7,23 The traditional armed-disarmed strategy thus offers an efficient tool for the synthesis of oligosaccharides with cis-trans glycosylation sequences.
In principle, the use of the selective activation concept offers a more flexible oligosaccharide assembly because this approach does not rely on the nature of the protecting groups. Instead, it requires a few leaving groups (LGa, LGb, LGc, etc., Scheme 3) that can be sequentially activated. Unfortunately, the application of this relatively simple concept to large oligosaccharide is still limited, so is the number of leaving groups compatible with the principle of sequential activation. This in part can be addressed by related semi-orthogonal and orthogonal strategies (vide infra).
This chapter is dedicated to a thorough overview of strategies making use of the principle of selective activation of one leaving group of another. The accumulated knowledge has translated into more advanced semi-orthogonal and orthogonal strategies that are also discussed below. Other general concept for oligosaccharide synthesis involves two-step activation or pre-activation. This approach allows for using the same leaving group in both donor and acceptor counterparts and since this approach is practically independent on the nature of the protecting groups used, a selective approach-like flexibility can be achieved. Due to the scope of this review, the two-step activations and pre-activation-based protocols will not be thoroughly discussed. The reader is referred to the original literature, which is rather abundant and is not limited to the references listed.24–26
3 Selective activation
3.1 Glycosyl chlorides, bromides, and iodides
Being relatively unstable derivatives, glycosyl chlorides and bromides can be selectively activated over other, more stable leaving groups, including thio, seleno and n-pentenyl glycosides. The first application of glycosyl bromides in selective activation strategy was reported in 1973. Zen et al.27 demonstrated that glycosyl bromide donor can be selectively activated over a thioglycoside acceptor in the presence of silver carbonate (AgCO3). Herein this principle is illustrated by a more recent example of a two-step synthesis reported by Lönn (Scheme 4).28,29 Accordingly, fucosyl bromide 1 was selectively activated over the S-ethyl glycosyl acceptor 2. The resulting disaccharide 3 was then coupled directly with acceptor 4 in the presence of MeOTf to afford trisaccharide 5. This example clearly illustrates the advantage of the selective activation strategy over the traditional approach that would imply additional protecting/leaving group manipulations after the first coupling step.
Stronger promoters for bromide activation, such as AgOTf, can be also successfully employed in selective activations over thioglycosides.30,31 For example, as reported by Oscarson et al., the synthesis of a tetrasaccharide of Vibrio cholerae O139 began with AgOTf-promoted activation of glycosyl bromide 6 over S-ethyl acceptor 7 (Scheme 5).32 The resulting disaccharide 8 was then subjected to deprotection (like in conventional strategy) and the resulting diol 10 was glycosylated with glycosyl bromide 11 to afford tetrasaccharide 12. All three glycosidic linkages were constructed using selective activation strategy although some interim protecting group manipulations were necessary.
Kovac et al. showed that glycosyl bromides and chlorides can be selectively activated with AgOTf in presence of the anomeric acetate.33 Pinto et al. reported selective activation of glycosyl bromide donors over phenyl selenoglycoside acceptors, which was realized in the presence of AgOTf and γ-collidine.34 Selective activation of glycosyl bromide over n-pentenyl glycoside acceptor in presence of AgOTf was demonstrated during the synthesis of an elicitor-active analog35 and in the synthesis of glycophosphatidylinositol.36 Mukaiyama and Kobashi introduced tri(1-pyrrolidino)phosphine oxide as an activator for glycosyl bromide suitable for conducting selective activations.37 The activation takes place via glycosyl phosphonium bromide intermediate. Scheme 6 illustrates the activation of glycosyl bromide 13 over thioglycoside 14 and glycosyl fluoride 15 leading to the respective disaccharides 16 and 17 in good yields.
Glycosyl chlorides can be also selectively activated with AgOTf in presence of thioglycosides.38 For example, this type of activation was employed in the synthesis of dodecasaccharide described by Ito et al.39,40 Selective activation of glycosyl chloride over glycosyl fluoride was also employed in activation of sialyl donor 18 over acceptor 19 to afford 2→8-linked disialoside 20 in 49% yield (Scheme 7).41,42
Gervay-Hague et al. described the selective activation of glycosyl iodide 21 over glycosyl acceptor 22 equipped with the anomeric acetate under neutral conditions (TBAI/DIPEA, Scheme 8).43,44 Oscarson et al.45 activated iodide donor 21 over thioglycoside acceptor 24 in the presence of triphenylphosphine oxide (Ph3PO) to afford the corresponding disaccharide 25.
3.2 Glycosyl fluorides
Glycosyl fluorides46 are very versatile building blocks because they can be used both as glycosyl donors and glycosyl acceptors in selective activations. Nicolaou and his co-workers showed that glycosyl fluorides can be activated in the presence of thioglycosides with AgClO4 and SnCl2. An example of the total synthesis of the tumor-associated Lex morif involving such principle is depicted in Scheme 9.47
As shown in Scheme 10, a similar concept was applied to the synthesis of tetrasaccharide 33 containing two sialic acid units, which were also connected by selective activation (vide supra). Thus, the activation of glycosyl fluoride donor 20 (see Scheme 7) over thioglycoside acceptor 32 was achieved in presence of AgOTf/SnCl2 and resulted in the formation of tetrasaccharide 33.41,42
In 2002, Mukaiyama et al. reported that a catalytic amount of protic acid, such as TfOH, HClO4 or C4F9SO3H is also suitable for the activation of fluoride donors over thioglycoside acceptors.48 Danishefsky demonstrated that glycals also can tolerate activation of glycosyl fluoride in the presence of Sn(OTf)2.49,50 Nicolaou and co-workers have described a related glycosylation strategy based on two-stage activation concept, whereby thioglycoside is first converted into a glycosyl fluoride, which is then used as a glycosyl donor for coupling with a thioglycoside acceptor.24,51
3.3 O-Leaving groups
3.3.1 Hemiacetals (1-OH derivatives)
The direct activation of 1-hydroxyl glycosyl donors is undoubtedly the most direct method of glycosylation as it does not require the use of a formal leaving group.52 In 1976, Leroux and Perlin developed the direct dehydrative glycosylation using triflic anhydride in the presence of a sterically hindered base, γ-collidine, and Bu4NBr53,54 The reaction proceeded via a stepwise activation of glycosyl donor 34 via the formation of the anomeric triflate and bromide intermediates. Resultantly, glycosylation of glycosyl acceptor 35 bearing an anomeric acetate group afforded disaccharide 36 in 60% yield (Scheme 11a). In the recent years, Gin and co-workers have developed a sulfonium-based electrophilic activation of hemiacetal donor via the activated anomeric oxosulfonium intermediate by the treatment with Ph2SO and Tf2O.55 Van der Marel et al. utilized the diphenyl sulfoxide protocol for selective glycosylation of hemiacetal with thioglycosides.56,57 Thus, Ph2SO/Tf2O-mediated dehydrative condensation of hemiacetal donor 37 with thioglycoside 38 afforded disaccharide 39 in 91%. This approach was then extended to the synthesis of a heparin oligosaccharide (vide infra).57
3.3.2 Acyl and carbonyl derivatives
In general, Lewis acids (SnCl4, FeCl3, BF3-OEt2, TsOH) are used as promoters to activate glycosyl esters and carbonates.52 Mukaiyama et al. utilized a preactivation-based glycosidation of glycosyl acetate 40 using iodotrimethylsilane followed by the addition of thioglycoside glycosyl acceptor 41 and phosphine oxide (Scheme 12a).58 Another example involves selective activation of anomeric carbonate 43 over thioglycoside 44 in the presence of TrB(C6F5)4 (Scheme 12b).59,60 Recently, Yu et al. introduced o-alkynylbenzoates as efficient glycosyl donors, which can be activated with catalytic amount of Ph3PAuOTf.61,62 The utility of glycosyl donor 46 was demonstrated by selective activation over thioglycoside acceptor 47 to afford the disaccharide 48 (Scheme 12c).61
3.3.3. O-Imidates
Glycosyl trichloroacetimidates, which over the years have become the most commonly used glycosyl donors, were introduced by Schmidt and co-workers.63 The selective activation of trichloroacetimidates over other glycosyl donors can be straightforwardly executed with TMSOTf64 or BF3-OEt2.65 For example, as a part of the synthesis of glycolipid of Mycobacterium smegmatis, TMSOTf-promoted coupling of trichloroacetimidate 49 and thioglycoside acceptor 50 afforded β(1→4) linked trisaccharide 51 in 71% yield (Scheme 13a).64
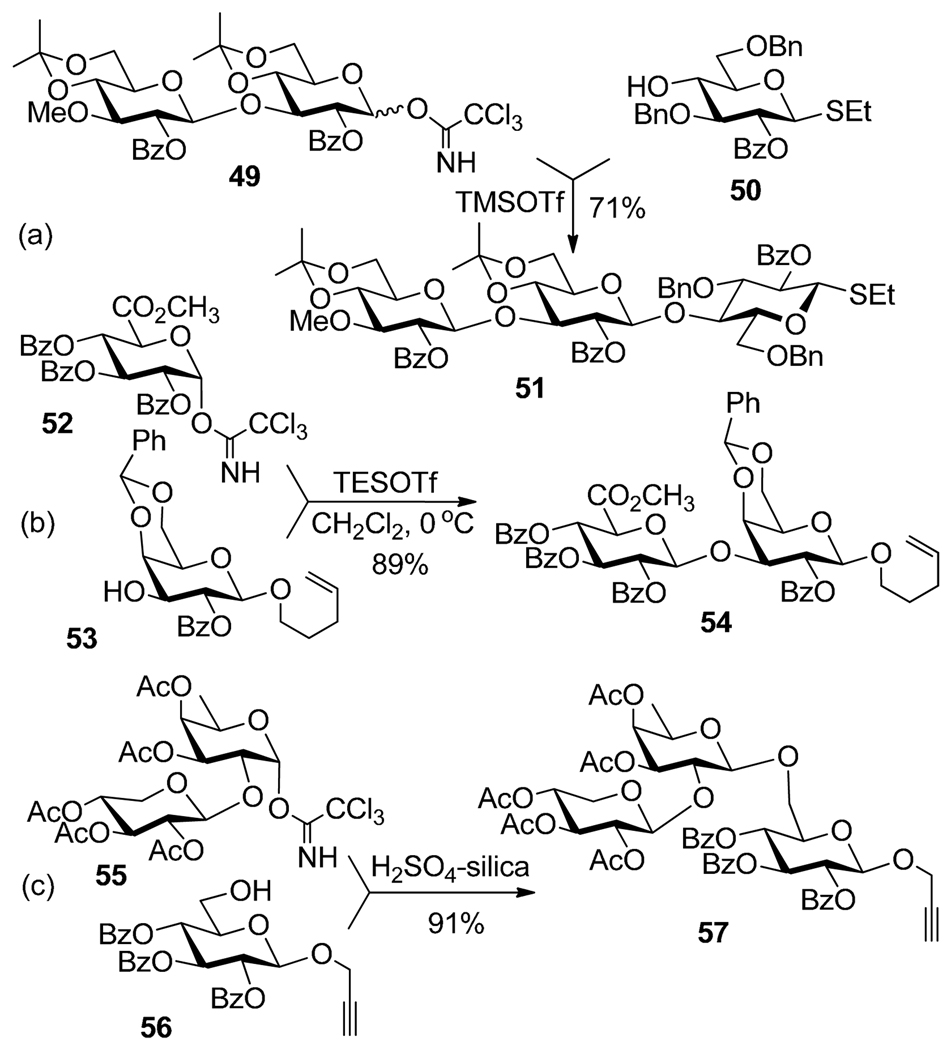
Selective activation of trichloroacetimidates over thioglycoside 50 (a), n-pentenyl 53 (b) and propargyl glycoside 56 (c).
Pinto et al. showed that glycosyl trichloroacetimidate donor can be activated with TESOTf over selenoglycoside acceptor.34 A number of syntheses involving the activation of trichloroacetimidates over n-pentenyl glycosides also emerged.66–68 For example, trichloroacetimidate glycosyl donor 52 was selectively activated over with n-pentenyl glycosyl acceptor 53 in the presence of TESOTf (Scheme 13b).67 Recently, Mukhopadhyay et al. showed that H2SO4-silica can be used to activate trichloroacetimidate 55 over propargyl glycosyl acceptor 56 leading to trisaccharide 57 in 91%.69
Similar glycosyl donors, (N-phenyl)trifluoroacetimidates introduced by Yu can also be selectively activated over a range of glycosyl acceptors bearing stable anomeric leaving groups.70 As reported by Seeberger et al.71 activation of (N-phenyl)trifluoroacetimidate 58 over n-pentenyl glycosyl acceptor 59 was accomplished in the presence of TMSOTf (Scheme 14a). Iadonisi et al. determined that the reactivity of glycosyl trichloro- and (N-phenyl)trifluoroacetimidates can be differentiated.72 It was found that a mild promoter, such as Yb(OTf)3, allows for selective activation of glycosyl trichloroactimidate building block 61 over glycosyl (N-phenyl)trifluoroacetimidate 62 (Scheme 14b). The resulting disaccharide is then coupled with glycosyl acceptor 63 in a one-pot manner. This was affected by using additional quantities of the promoter and led to the formation of trisaccharide 64 in 40% yield over two steps.

Selective activation of (N-phenyl)trifluoroacetimidate 58 over n-pentenyl glycoside 59 (a) and sequential activation of 61 and 62 (b).
Mukaiyama and co-workers reported selective activation of cyclic O-imidate donor, 6-nitro-2-benzothiazoate 65 over thioglycoside 66 or glycosyl fluoride acceptor 15.73 As depicted in Scheme 15, HB(C6F5)4-catalyzed selective activations resulted in the formation of disaccharides 67 and 68, respectively, in good yields. Glycosyl thioformimidates can be activated over fluorides and thioglycosides in the presence of catalytic amount of protic and Lewis acid.74
3.3.4 O-P derivatives
Several glycosyl donors possessing an O-P anomeric motif have been investigated.75 Diethyl and dibenzyl glycosyl phosphites independently introduced by Schmidt76 and Wong,77 respectively, require only catalytic amount of TMSOTf (usually 10–20%), which allows for their selective activations over a range of leaving groups. For example, the coupling of sialyl phosphite donor 69 and thioglycoside acceptor 70 was accomplished using TMSOTf.78 Also demonstrated that the coupling of glycosyl phosphites with glycals can be affected in the presence of TMSOTf.79 Further expansion emerged with the development of glycosyl diphenyl phosphates,80 diphenylphosphineimidates,81 and phosphoroamidates.82 Towards the synthesis of ganglioside GM3, sialyl phosphite 72 could be selectively activated over phosphorodiamidate acceptor 73 in the presence of TMSOTf, BF3-OEt2 or TfOH (Scheme 16b).83
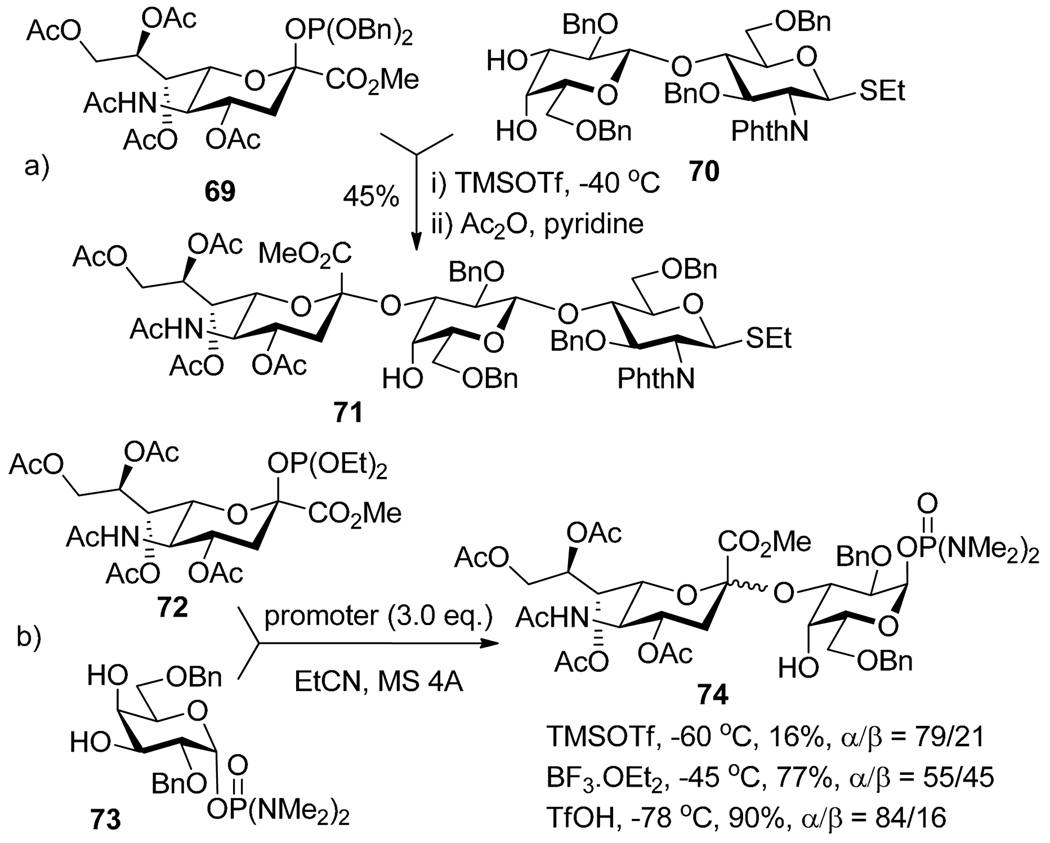
Selective activation of glycosyl phosphites over thioglycoside 70 (a) and phosphorodiamidate 73 (b).
Seeberger and co-workers expanded the use of glycosyl phosphates as glycosyl donors.84,85 TMSOTf, TBSOTf, or BF3.OEt2 were proved to be suitable promoters for selective activation of glycosyl phosphates. For example, selective activation of phosphate 75 over thioglycoside acceptor 76 in the presence of TMSOTf resulted in the formation of disaccharide 77 in 96% yield (Scheme 17a). Similarly, glycosyl phosphate 78 was activated over n-pentenyl acceptor 79 that resulted in the formation of disaccharide 80 in 82% yield (Scheme 17b). Mukaiyama et al. introduced glycosyl diphenylphosphinites as a glycosyl donors that can be activated over thioglycoside and fluoride acceptors in the presence of MeI.58
3.3.5 Transglycosylation (n-pentenyl, propargyl, and hetaryl glycosides as glycosyl donors)
n-Pentenyl glycosides introduced by Fraser-Reid86 can be activated with an electrophilic reagent, most commonly IDCP, NIS/TfOH or NIS/TMSOTf. Since these are essentially the same activation conditions as those for the activation of common thioglycosides, the use of n-pentenyl glycosides as glycosyl donors in selective activations is rare. Although n-pentenyl glycoside 81 could be selectively activated with IDCP over thioglycoside 82 (Scheme 18a)87 this coupling required the enforcement by the electronic effects. Resultantly, only armed n-pentenyl glycosides could be activated over the disarmed thioglycosides. Lopez et al. demonstrated that n-pentenyl glycosides can be selectively activated over glycosyl fluoride acceptors (vide infra).88 More recently, Hotha et al. observed that n-pentenyl glycoside 84 could be activated over propargyl glycosyl acceptor 85 in the presence of NIS/TMSOTf (Scheme 18b).89

n-Pentenyl glycosyl donors in selective glycosylation over disarmed thioglycoside 82 (a) and propargyl glycoside 85 (b).
The utility of 3-methoxy-2-pyridyl glycoside as a glycosyl donor in selective glycosylations was described by Hanessian et al.90 As depicted in Scheme 19a, donor 87 was selectively activated over thioglycoside 88 in the presence of Cu(OTf)2. Very recently, Hotha described that propargyl glycoside 90 can be selectively activated over n-pentenyl glycoside 91 in the presence of AuBr3 at 65°C.89 As depicted in Scheme 19b, disaccharide 92 was isolated in 65 % yield. Chenault et al. discovered that isopropenyl glycosides can be activated over n-pentenyl glycosides in the presence of TMSOTf.91 Jensen and co-workers reported a glycosylation method employing methyl 2-hydroxy-3,5-dinitrobezoate glycosides (methyl 3,5-dinitrosalicylate, DISAL) as a glycosyl donors.92 DISAL glycosyl donor could be selectively activated over thioglycoside acceptor in the presence of LiClO4 and Li2CO3 as the promoter system.93 Kim et al. discovered that 2-(benzyloxycarbonyl)benzyl (BCB) glycosides are perfectly stable, whereas the corresponding 2-(hydroxycarbonyl)benzyl (HCB) counterparts can be activated in presence of Tf2O and DTBMP.94 This phenomenon was applied to selective activation of 2-(hydroxycarbonyl)benzyl donor over 2-(benzyloxycarbonyl)benzyl acceptor. This activation, however, was performed using pre-activation conditions under which HCB was first treated with DTBMP (30 min), followed by the treatment with Tf2O. After that, BCB glycosyl acceptor was added, therefore, formally this cannot be classified as the selective activation.
3.4 S-Leaving groups
3.4.1 Sulfoxides, thiocyanates, xanthates, etc
Glycosyl sulfoxides prepared from the corresponding thioglycosides via oxidation,95 can be glycosidated in the presence of trifluoromethanesulfonic anhydride.96,97 In 1994, van Boom et al. reported that TMSOTf and triethylphosphite (TEP) is an effective promoter system for the selective activation of phenylsulfenyl glycosides over phenylthio glycoside acceptors.98 The synthesis of antigens Lea, Leb and Lex utilizing the glycosyl sulfoxide method was reported by Kahne (Scheme 20).99 Thus, glycosidation of sulfoxide 93 in the presence of Tf2O and 2,6-di-tert-butyl-4-methylpyridine (DTBMP) over S-phenyl glycoside 94 resulted in the formation of disaccharide 95 in 86% yield. The latter was converted into a glycosyl acceptor 96, which was subjected to another round of selective glycosylation with glycosyl sulfoxide 97. The resulting trisaccharide 98 was obtained in 95% yield.
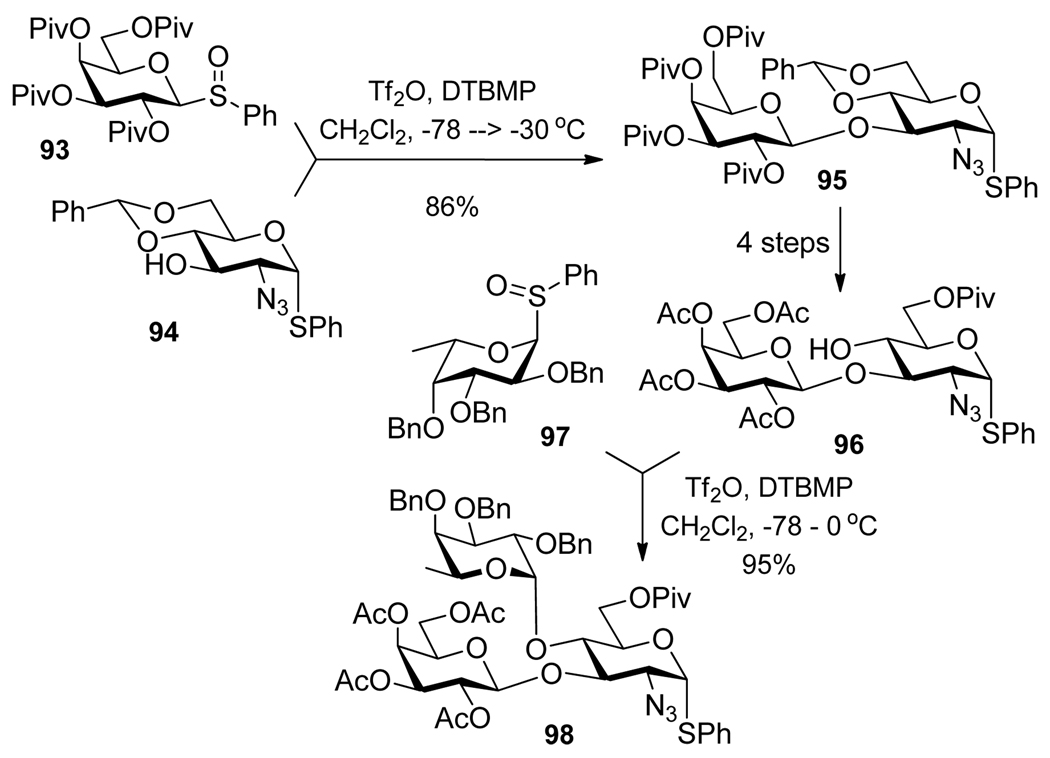
Utilizing glycosyl sulfoxides 93 and 97 in selective activation as a part of the synthesis of the Lea antigen.
Kochetkov and co-workers showed that glycosyl thiocyanates can be selectively activated over thioglycoside acceptors.100 As depicted in Scheme 21a, thiocyanate 99 was selectively activated over 6-O-tritylated thioglycoside 100 in the presence of triphenylmethylium perchlorate (TrClO4) as an activator. Fügedi et al. introduced glycosyl 1-piperidinecarbodithioates as glycosyl donors that can be activated in the presence of AgOTf or TMSOTf.101 As depicted in Scheme 21b, selective activation of 1-piperidinecarbodithioate donor 102 over thioglycosides acceptor 103 was affected in the presence of AgOTf and resulted in the formation of disaccharide 104. Along similar lines, condensation of pentafuranosyl dithiocarbamate with 5-nitro-2-pyridyl thioglycoside in the presence of NIS/TfOH was described by Bogusiak and Szeja.102
3.4.2 Thioimidates
The first wave of glycosyl thioimidates, compounds with a generic leaving group SCR1=NR2, included benzothiazolyl,103 pyridin-2-yl,104–106 pyrimidin-2-yl,104,107 imidazolin-2-yl,104 and 1’-phenyl-1H-tetrazolyl.108 Although all of these derivatives have been tested as glycosyl donors and promising results have been obtained, the application of this class of compounds to selective activations emerged later. A few years ago, Demchenko et al. found that S-benzoxazolyl (SBox)109 and S-thiazolinyl (STaz) glycosides110 can be selectively activated over other classes of leaving groups. For example, AgBF4 – promoted activation of SBox glycoside 105 over n-pentenyl glycoside acceptor 106 resulted in the formation of disaccharide 107 in 86% yield (Scheme 22a).111 De Meo and Parker described that SBox sialyl donor 108 can be activated over SEt glycosyl acceptor 109 in the presence of AgOTf (Scheme 22b).112

SBox glycosides in selective activation over O-pentenyl acceptor 106 (a) and thioglycoside acceptor 109 (b).
STaz glycosyl donor 111 was glycosidated selectively over thioglycoside acceptor 112 in the presence of AgBF4 (Scheme 23a).111 SBox glycosyl donor 114 could be selectively activated over STaz acceptor 115 in the presence of Bi(OTf)3 to afford disaccharide 116 in 69% yield (Scheme 23b).113
3.4.3 S-Alkyl and S-aryl glycosides
Alkyl and aryl thioglycosides have been widely studied as versatile glycosyl donors for oligosaccharide synthesis.114–116 As has been demonstrated by a number of examples (vide supra), thioglycosides can act as glycosyl acceptors. These properties make thioglycosides suitable building blocks for the use in a broad variety of concepts for oligosaccharide synthesis including chemoselective, selective and iterative glycosylations. As shown by Ogawa et al., selective activation of thioglycosides over glycosyl fluorides can be executed in the presence of NIS and AgOTf as a promoter system.12 In addition, other promoters including NIS/TfOH,40 MeOTf,39,117 and DMTST118,119 are also capable of the selective activation of thioglycosides over fluorides. For example, selective activation of thioglycoside 117 over fluoride acceptor 118 in the presence of DMTST led to the formation of trisaccharide 119 in 85% yield (Scheme 24a).119 Selective activation of thioglycoside 120 over S-thiazolinyl glycosyl acceptor 115 could be affected in the presence of NIS and catalytic TfOH (Scheme 24b).110 Boons reported that the glycosidation of thioglycoside 121 with di-O-trityl pentenyl glycosyl acceptor 122 can be achieved in the presence of MeOTf (Scheme 24c).120 The unusual regioselectivity obtained here is due to the observation reported by Kochetkov et al. that trityl ethers reverse the reactivity of alcohols towards glycosylation.121 Boons and co-workers also demonstrated that the increase of steric hindrance around the active site of the leaving group has a dramatic effect on the leaving group ability.122 Thus, it was shown that an S-ethyl glycoside can be selectively activated over dicyclohexylmethyl thioglycoside in the presence of NIS/TMSOTf at low temperature.
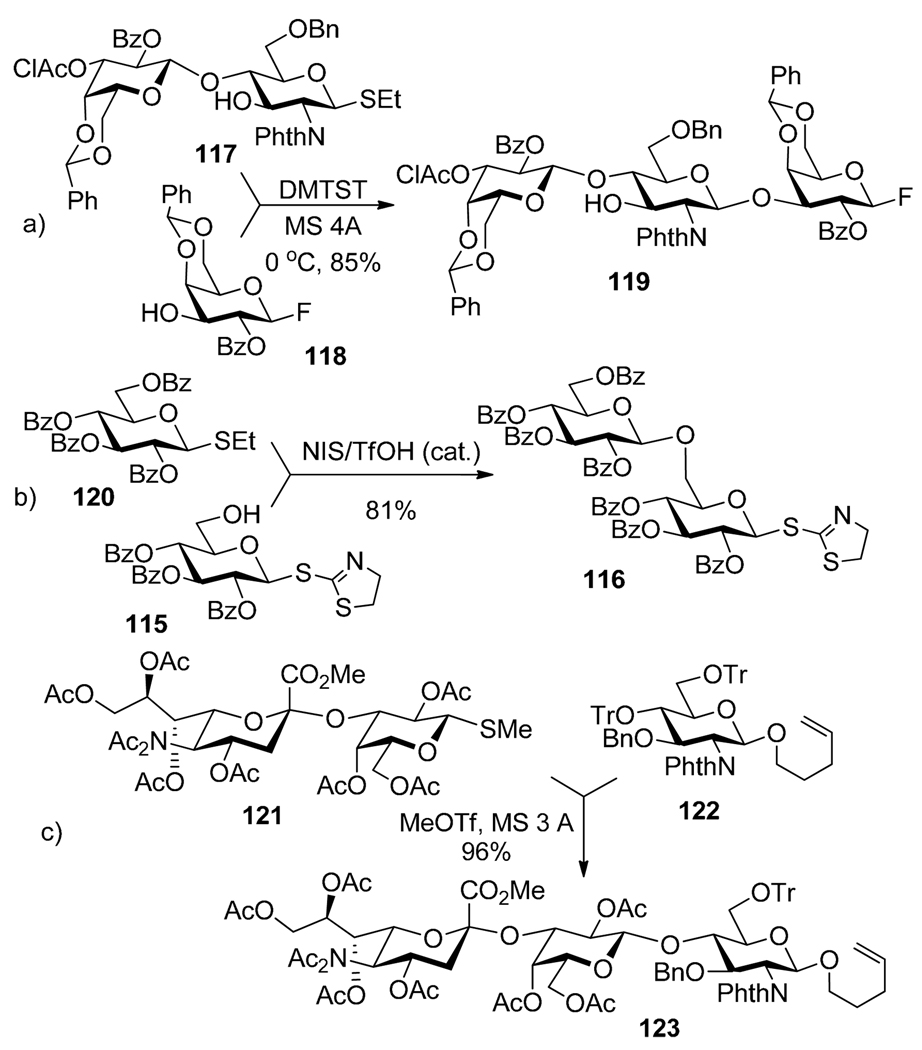
Selective activation of thioglycosides over glycosyl fluoride 118 (a), S-thiazolinyl 115 (b), and n-pentenyl 122 (c) acceptors.
Ensley demonstrated that the activation of thioglycosides over 1-O-acyl glycosyl acceptor can be performed in the presence of NIS/AgOTf.123 It was also reported that glycals are stable under MeOTf-promoted activation of thioglycosides.124 Recently, it was reported that S-tolyl donor 124 can be selectively activated over propargyl glycosyl acceptor 125 in the presence of NIS/H2SO4-silica.69 As shown in Scheme 25, the resulting disaccharide 126 (obtained in 87% yield) was converted into glycosyl acceptor 127 and then subjected to glycosylation with S-tolyl glycosyl donor 128 under the same reaction conditions.
3.5 Other classes of glycosyl donors
Anomeric phenylseleno glycosides introduced by Pinto et al. are very effective glycosyl donors that can be selectively activated over thioglycosides with AgOTf and K2CO3.34,125 Selenoglycosides are also more reactive towards MeOTf or NIS/TfOH than their thioglycoside counterparts.126,127 For example, Ley et al. reported selective activation of phenylseleno glycoside 130 over thioglycoside acceptor 131 in application to convergent synthesis of GPI anchor of Trypanosoma brucei (Scheme 26).126

Convergent synthesis of pentasaccharide 135 using selective activation of selenoglycosides over thioglycosides.
This coupling was performed in the presence of MeOTf and resulted in the formation of trisaccharide 132 in 75% yield. The latter was then converted into glycosyl acceptor 133 which was glycosylated with selenoglycoside 134 in the presence of MeOTf. Recently, electrochemical activation of selenoglycoside donors over thioglycoside acceptor has been demonstrated.128 The use of telluroglycosides as glycosyl donors was reported by Yoshida129,130 and Stick.131,132 These glycosyl donors can be activated either electrochemically129,130 or in the presence of MeOTf, NIS/t-BuOCl, or NBS/TMSOTf.133 Selective activation of telluroglycosides over thioglycosyl133 and selenoglycosyl acceptors132 has been demonstrated.
Orthoesters have been used as useful glycosyl donors for 1,2-trans glycosylation for decades.134 Their activation is typically achieved in the presence of a Lewis acid or a mild electrophilic promoter, and these conditions typically permit their selective activation over a number of leaving groups of different classes. For example, it was demonstrated that ethylthio orthoester 136 can be activated over conventional thioglycoside 137 using NIS/TfOH (Scheme 27a).135 Lopez et al. reported that n-pentenyl 1,2-orthoesters are activated over glycosyl fluoride acceptors in the presence NIS and Yb(OTf)3.88 Recently, Hotha et al. reported that n-pentenyl 1,2-orthoester 139 can be selectively activated over propargyl glycoside 85 in the presence of NIS/Yb(OTf)3 (Scheme 27b).89 Along similar lines, propargyl 1,2-orthoester 141 can be selectively activated over n-pentenyl acceptor 142 in the presence of AuBr3 leading to trisaccharide 143 (Scheme 27c). Cyanoethylidene approach to 1,2-trans-glycosylation of tritylated acceptors has been extensively studied by Kochetkov and co-workers.136,137 As reported by Boons, this approach allows for selective activation of cyanoethylidene donor over n-pentenyl glycosyl acceptor in the presence of TrClO4.120,138 Backinowsky et al. reported that 1,2-O-(1-cyanoethylidene) donors can be activated with TrClO4 over thioglycoside acceptors.139
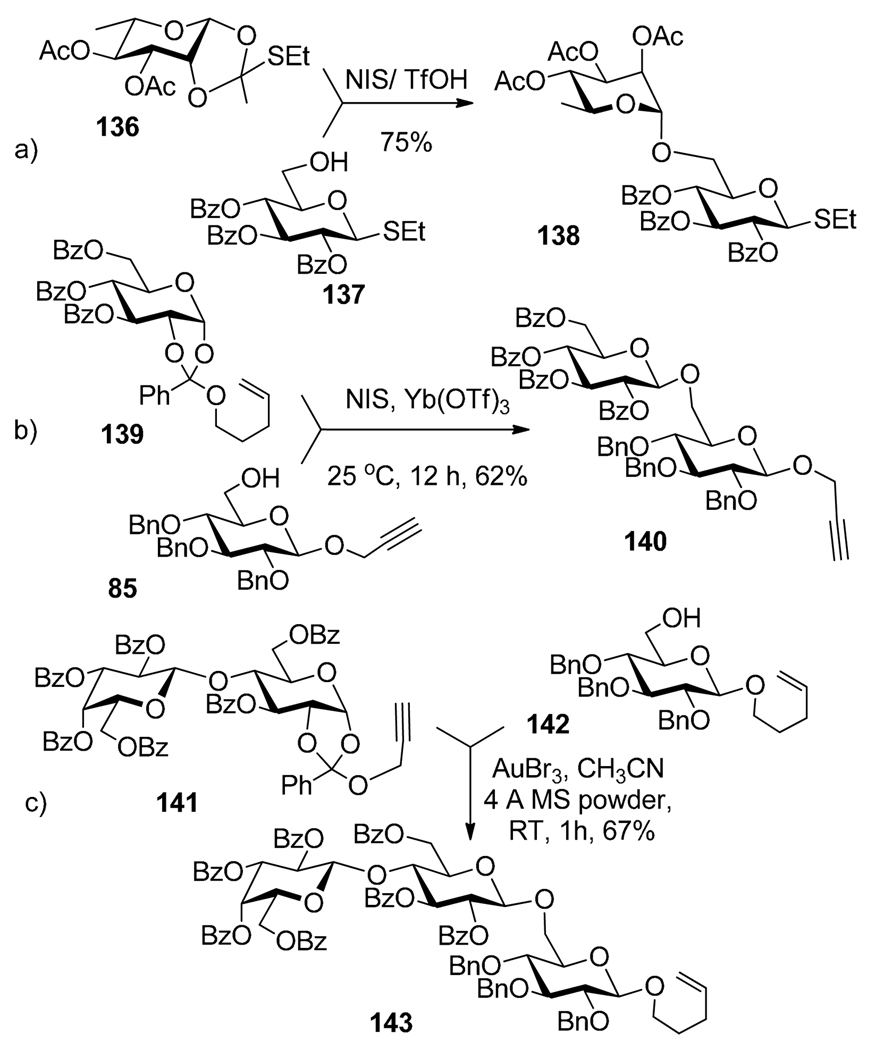
Selective activation of orthoesters over thioglycoside 137 (a), propargyl glycoside 85 (b), and pentenyl glycoside 142 (c).
Danishefsky and co-workers developed a convenient method for the preparation of 1,2-anhydro sugars from glycals using dimethyldioxirane (DMDO)140 as an effective epoxidation reagent.141 This allowed to apply 1,2-epoxides as very effective glycosyl donors.142,143 For example, 1,2-anhydrosugar 145 generated from glycal 144 could be activated over glycal acceptor 146 in the presence of ZnCl2 to afford 1,2-trans-linked disaccharide 147 in 81% (Scheme 28). The epoxidation-glycosylation sequence can be then reiterated to yield larger oligosaccharides, although similarly to other pre-activation type of couplings, this approach cannot be classified as the formal oligosaccharide synthesis via selective activations.
4. Multistep sequences based on selective activations
Studies presented in the previous sections allowed to compile a comprehensive database on reactivity of different classes of glycosyl donors, which led to the development of selective activation-based multistep oligosaccharide synthesis. Continuation of the activation sequence beyond previously discussed couplings of building blocks equipped with LGa and LGb requires the availability of a LGc, which would withstand activation conditions for the LGb, etc. However, these elongated multi-step sequences are not yet routinely available (vide infra). This can be circumvented by performing additional modifications between glycosylations that allow repetition of the sequence involving repetitive LGa and LGb activations. An example of such approach is the synthesis of heparin-like pentasaccharide 157 by utilizing the diphenyl sulfoxide—triflic anhydride dehydrative protocol144 for the activation of the hemiacetal 148 over thioglycoside acceptor 149 (Scheme 29).57
The phenylthio moiety of the resulting disaccharide 150 was then directly coupled with glycosyl acceptor 151 using benzenesulfinyl piperidine (BSP)-triflic anhydride System developed by Crich.145 The resulting trisaccharide 152 was then converted into the corresponding hemiacetal derivative 153, which was selectively activated over thioglycoside acceptor 154 in the presence of diphenyl sulfoxide-triflic anhydride. The resulting tetrasaccharide 155 isolated in 51% yield was glycosidated with glycosyl acceptor 156 in the presence of the BSP/Tf2O promoter system to furnish the target pentasaccharide 157 in 53% yield.
An example with the involvement of three leaving groups is depicted in Scheme 30, in which trichloroacetimidoyl is used as a LGa, phenylselnyl as LGb, and ethylthio as LGc.34 Thus, activation of trichloroacetimidate 158 over selenoglycoside acceptor 159 was achieved in the presence of TMSOTf. The resulting disaccharide 160 isolated in 90% was then coupled with thioglycoside acceptor 112 in the presence of AgOTf and K2CO3 leading to trisacccharide 161 in 81% yield. In principle, the thioethyl moiety of 161 can be activated further. A similar sequence using bromide (LGa), selenoglycoside (LGb) and thioglycoside (LGc) was also reported.34
Another relevant example is the use of the S-benzoxazolyl (SBox) moiety as LGa, S-ethyl as LGb, and O-pentenyl as LGc depicted in Scheme 31.146,147 SBox glycosyl donor 114 was selectively activated over the SEt acceptor 82 in the presence of AgOTf to produce disaccharide 162 in 99% yield. The latter disaccharide was glycosidated with the n-pentenyl acceptor 163 in the presence of MeOTf. Finally, the n-pentenyl moiety of the trisaccharide 164 was activated with NIS/TMSOTf. A similar example included the following three-step activation sequence performed in a one-pot fashion: SBox as LGa, S-thiazolinyl (STaz) as LGb, and S-ethyl as LGc.148
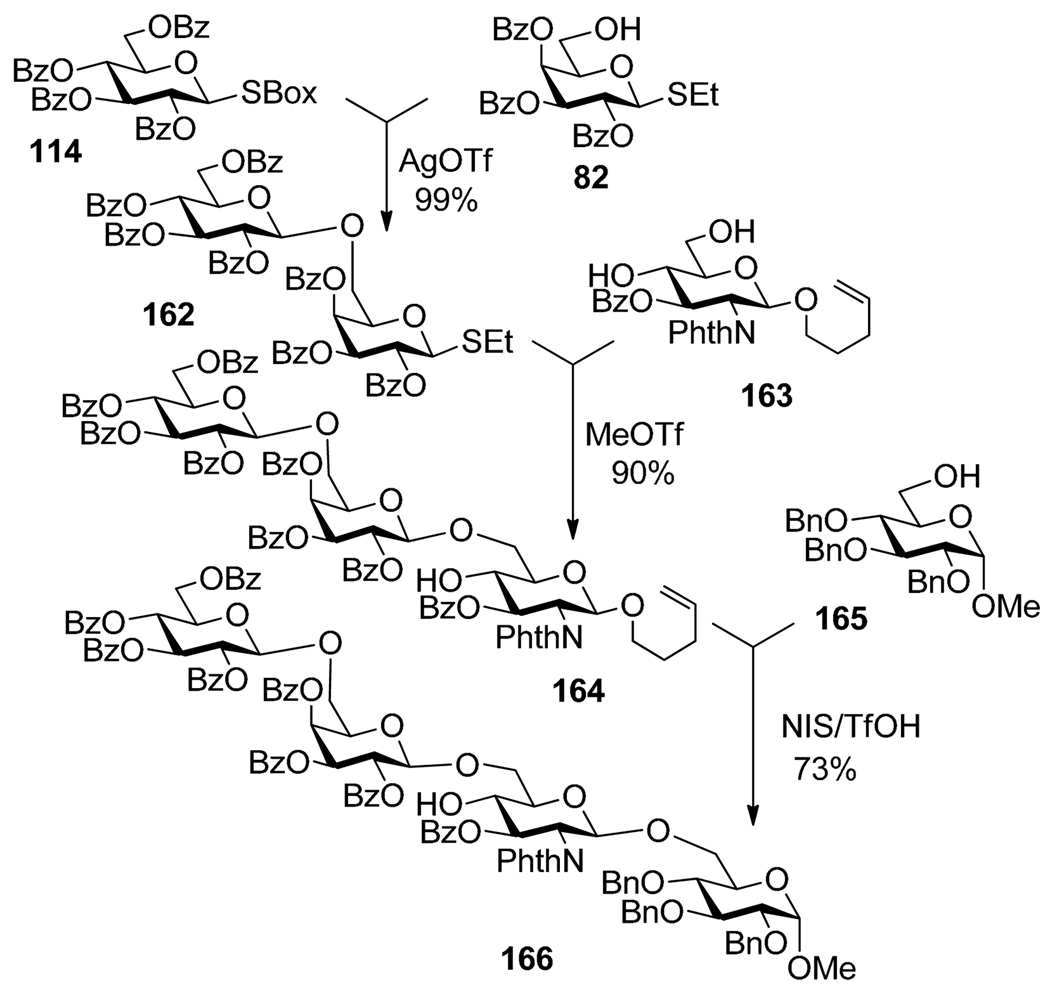
Three-step SBox→ SEt → n-pent sequential selective activation for the synthesis of tetrasaccharide 166.
Very recently, the synthesis of a β-(1→6)-linked hexasaccharide via the five-step selective activation sequence employing six different leaving groups was developed (Scheme 32).149 The synthesis began with the selective activation of thiocyanate donor 167 over STaz glycosyl acceptor 115 using Cu(OTf)2. The STaz leaving group of disaccharide 116 was then directly activated over SBox acceptor 168 with benzyl bromide to give trisaccharide 169. The SBox moiety of 169 was selectively activated over the fluoride acceptor 170 in the in presence of MeOTf. The resulting tetrasaccharide 171 was glycosidated with thioglycoside acceptor 137 in presence of AgClO4/Cp2ZrCl2 to provide pentasaccharide 172. Finally, the S-ethyl leaving group of 172 was selectively activated over n-pentenyl acceptor 91 with MeOTf to furnish the hexasaccharide 173 in 72% yield.149
5. Semi-orthogonal approach
The orthogonal approach makes use of two leaving groups, LGa and LGb, which can be independently activated one over another and vice versa. A semi-orthogonal strategy is based on a similar principle, but it requires the additional enforcement by electronic effects, similarly to that of the “armed-disarmed concept”. Overall, this concept combines advantages of both selective and chemoselective activation; its utility, however, is limited by the protecting groups that also often determine the stereoselectivity. It was demonstrated87 that either armed or disarmed thioglycoside can be selectively activated over either armed or disarmed n-pentenyl glycosides in the presence of MeOTf. However, only armed n-pentenyl could then be activated over a disarmed thioglycoside acceptor using IDCP as a mild promoter. Thus, S-ethyl donor 174 was selectively activated over n-pentenyl acceptor 142 in the presence of MeOTf (Scheme 33). The n-pentenyl moiety of the resulting trisaccharide 175 was then subjected to selective/chemoselective activation over S-ethyl acceptor 82 in the presence of IDCP leading to tetrasaccharide 176 in 92% yield.

Semi-orthogonality of thioglycosides and n-pentenyl glycosides applied to the synthesis of tetrasaccharide 176.
This semi-orthogonal concept was recently extended to another suitable pair of leaving groups, namely fluoride and n-pentenyl.88 While either armed or disarmed glycosyl fluorides underwent glycosylation with armed/disarmed n-pentenyl glycoside in presence of Yb(OTf)3, only armed n-pentenyl glycosides could be activated over disarmed glycosyl fluorides in presence of IDCP. As depicted in Scheme 34, fluoride donor 177 was selectively activated over pentenyl 178 in the presence of Yb(OTf)3. Conversely, the activation of armed n-pentenyl glycoside 180 was achieved selectively over fluoride 181 to afford disaccharide 182. No activation of benzoylated n-pentenyl glycosides could be accomplished under these reaction conditions.
6. Orthogonal activation
The combination of two chemically distinct glycosylation reactions, in which one of the leaving groups is activated while the other one remains intact, and vice versa, has led to the discovery of the orthogonal strategy for oligosaccharide synthesis.12 This unique, and virtually one of the most conceptually attractive techniques for expeditious oligosaccharide synthesis, requires the use of two orthogonal classes of leaving groups.150,151 As with the selective activation strategy, at the first step the glycosyl donor bearing LGa is activated over the glycosyl acceptor bearing LGb. Uniquely to the orthogonal strategy, however, the LGb is then activated over LGa of the glycosyl acceptor (Scheme 35). This activation sequence can then be reiterated to give straightforward access to larger oligosaccharides. Ideally, the orthogonal approach allows for an unlimited number of reiterations of the two orthogonal leaving groups, LGa and LGb, which is conceptually very attractive. On practice, however, the efficiency and yields of glycosidation are usually inversely correlated to the size of the glycosyl donor involved.12,152 And since this approach presumes the elongation of glycosyl donors, the yields are typically decreased dramatically at the later stage of the assembly.
6.1 Fluorides and thioglycosides
The classic variation of the orthogonal activation route introduced by Ogawa et al. involves building blocks bearing S-phenyl and fluoro leaving groups.12 Phenyl thioglycoside 183 can be selectively activated over fluoride acceptor 184 in the presence of NIS/AgOTf (Scheme 36). The fluoro leaving group of disaccharide 185 can then be activated over thioglycoside acceptor 186 in the presence Cp2Hf2Cl2/AgOTf. This selective activation sequence can be then reiterated; to illustrate this, trisaccharide S-phenyl donor 187 was activated over fluoride 184 to provide tetrasaccharide 188 in 66% yield.
6.2 Thioglycosides and thioimidates
A combination of S-ethyl and S-thiazolinyl (STaz) leaving groups also represents a promising orthogonal pair. STaz glycosides can be activated selectively with AgOTf, whereas the ethyl thioglycoside can be activated with NIS and catalytic TfOH.110 This finding was applied to the synthesis of pentasaccharide 193, as depicted in Scheme 37.152 Thioglycoside donor 189 was first activated over the STaz acceptor 115 in the presence of NIS/cat. TfOH. The resulting disaccharide 190 isolated in 98% yield was in turn activated over thioglycoside acceptor 137 with AgOTf to give trisaccharide 191 in 93% yield. The sequence was then reiterated as follows. Thioglycoside donor 191 was activated over STaz acceptor 115 in the presence of NIS/cat. TfOH. The resulting tetrasaccharide 192 was selectively activated over thioglycoside acceptor 137 in the presence of AgOTf to give pentasaccharide 193 in 59% yield.
6.3 Thioimidate-only (SBox and STaz glycosides)
A related approach involving orthogonal activation of STaz and SBox glycosides has also recently emerged.111 SBox glycosides can be selectively activated with Cu(OTf)2152 or Bi(OTf)3113 over the STaz leaving group. As shown in Scheme 38, STaz leaving group that is typically more stable than SBox towards a majority of thiophilic activation conditions, can be also selectively activated over SBox in the presence of relatively mild alkylation reagents, benzyl bromide or methyl iodide. Mechanistic studies showed that the selective activation is based on the different activation modes of these leaving groups. Thus, it was found that preferential glycosidation of a certain thioimidate is not simply determined by the strength of activating reagents; instead, the type of activation – direct (for SBox) vs. indirect (for STaz) – plays the key role. The activation of STaz donor 194 over SBox acceptor 168 in the presence of BnBr afforded disaccharide 195 in 76% yield. The latter could be then activated over STaz acceptor in the presence of Bi(OTf)3 at 0 °C. The resulting trisaccharide 196 was isolated in 62% yield.
6.4 n-Pentenyl and propargyl activation
Recently, Hotha et al. reported a new promising pair of leaving groups: propargyl vs. n-pentenyl.89 According to this study, NIS/TMSOTf served as an efficient activator for the selective activation of n-pentenyl glycoside 84 (see Scheme 18b). On the other hand, propargyl glycosyl donor 90 can be selectively activated over propargyl glycosyl acceptor 91 in the presence of AuBr3 to afford disaccharide in 65% (see Scheme 19b). This activation is particularly efficient for benzoylated glycosyl acceptor 91 as glycosylation of its benzylated counterpart led to a modest yield of the respective disaccharide. This implies a more semi-orthogonal-like activation (vide supra) rather than purely orthogonal character of these two leaving groups.
7 Selective and orthogonal activations in modern high throughput technologies
7.1 Intramolecular glycosylations
The intramolecular aglycone delivery (IAD) approach was introduced by Hindsgaul153 and Stork154 as a reliable method to obtain β-mannosides. Ogawa et al. then reported the use of a p-methoxybenzyl (PMB) group at the C-2 position as a new stereocontrolling tether for β-mannosylation.38,155 The p-methoxybenzyl assisted IAD process was used as the key reaction for the construction of β-mannoside containing fragment that also involved selective activation (Scheme 39).39,117 Accordinly, thioglycoside donor 197 was linked to fluoride acceptor 184 via DDQ-mediated tethering. The resulting construct 198 was subjected to the treatment with MeOTf and DTBMP that allowed for selective activation of the S-methyl group over the fluoro group to afford β-mannoside 199. The free hydroxyl group in 199 was then acetylated, and the resulting fluoride donor 200 was glycosidated with acceptor 201. A similar approach was also investigated using polymer support.156
7.2 One-pot synthesis
Conceptually, one-pot strategies offer the fastest pathway to oligosaccharides, as the sequential glycosylations are performed in a single flask (pot) and do not require purification of the intermediates. Of particular importance to the successful one-pot glycosylation is complete consumption of all building blocks and the high yield and complete stereoselectivity in each glycosylation step. Many variations of the one-pot strategy have been developed and the reader should be referred to the recent review articles.157,158 Herein we focus our discussion on selective activation that requires a set of different leaving groups (LGa, LGb, LGc) as illustrated in Scheme 40.
The first one-pot synthesis based on the leaving group based activation concept was reported by Takahashi and co-workers.35 As depicted in Scheme 41, this synthesis involved the use of trichloroacetimidate donor 203, which was selectively activated over thioglycoside 204 with TMSOTf. The SPh moiety of the intermediate tetrasaccharide was then directly glycosidated by adding glycosyl acceptor 205 and NIS/TfOH to provide hexasaccharide 206 in 50% yield over two steps. This approach incorporates advantages of the convergent block strategy according to which pre-synthesized oligosaccharide building blocks i.e. 203 and 205 are converged together during the final assembly. A number of related two-step one-pot syntheses have emerged, and the most representative examples are surveyed below. As depicted in Scheme 42a, reaction of bromide 207 and S-phenyl acceptor 208 was affected in the presence of silver triflate. The S-phenyl leaving group of the intermediate disaccharide was then glycosidated by adding glycosyl acceptor 209 and NIS. The resulting trisaccharide 210 was isolated in 84% yield over two steps.159 In a similar fashion, tetrasaccharide glycosyl glycerol 214 which represents the core structure of a glycoglycerolipid of M. taiwanesis, was synthesized as depicted in Scheme 42b.160 A trisaccharide intermediate was formed from the glycosyl phosphite 211, which was selectively activated over selenoglycoside acceptor 212 in the presence of TMSOTf. Subsequent addition of glycosyl acceptor 213 and NIS allowed to produce the target tetrasaccharide 214 in 46% yield over-all. Seeberger and co-workers presented an efficient one-pot synthesis of a pentasaccharide 218 shown in Scheme 42c.161
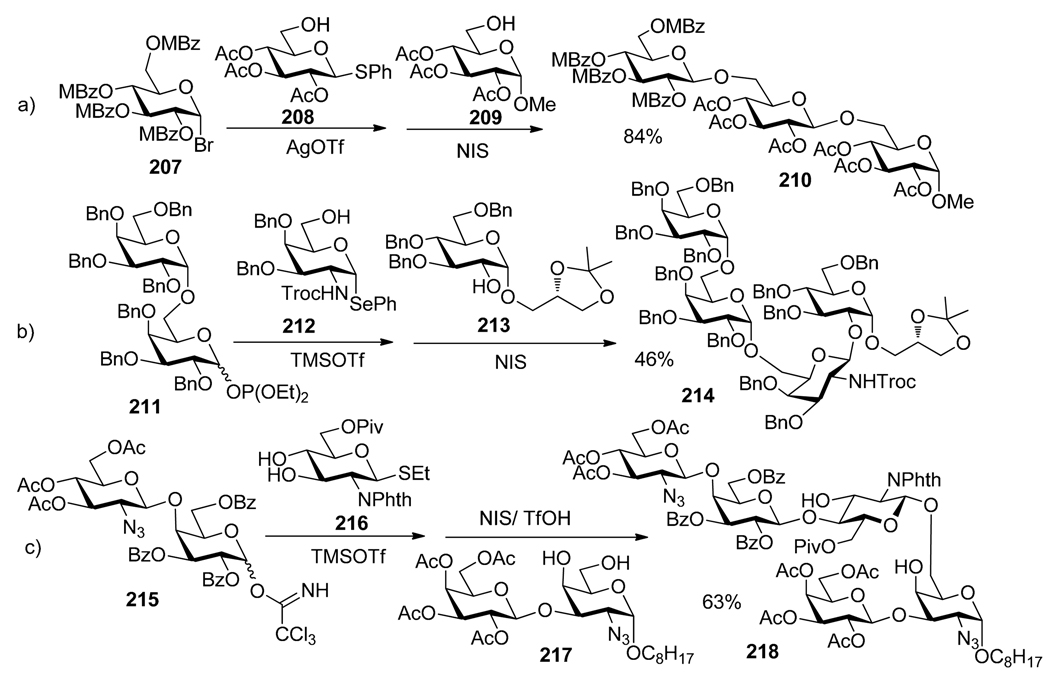
Typical examples of two-step one-pot oligosaccharide syntheses of oligosaccharides 210, 214 and 218.
A representative example involving three sequential glycosylation steps is shown in Scheme 43.148 SBox donor 114 was activated over S-ethyl acceptor 82 with AgOTf. Thioethyl moiety of the intermediate was then activated over the added STaz acceptor 115 by the addition of NIS and catalytic TfOH. Finally, the STaz moiety was reacted with glycosyl acceptor 165 in the presence of additional quantity of AgOTf. As a result, linear tetrasaccharide 219 was obtained in 73% yield over three steps.
Utilizing longer sequences of selective activations are not yet routinely available. Although a number of one-pot syntheses of large oligosaccharides has emerged, all are based on mixed conventions, typically a combination of selective and chemo- or regioselective sequential activations. For example, Ley’s group reported the synthesis of a GPI anchor involving building blocks with well defined levels of reactivity (Scheme 44).162 Thus, armed and disarmed glycosyl fluorides (220, 221), armed and disarmed selenoglycosides (222, 223) and thioglycoside 224 were all sequentially activated to provide the target linear pentamannoside 225 in 8% overall yield in four steps. Evidently, the first and the third steps involved chemoselective activations whereas the second and fourth steps involved selective activations of fluoride over SePh and SePh over SPh, respectively. Mukaiyama reported an elaborate one-pot sequence that resulted in the formation of a phytoalexin elicitor heptasaccharide 230 in 48% yield.163 As depicted in Scheme 45, the synthesis of heptasaccharide 230 involved a combination of sequential selective (fluoride 226 over thioglycoside 227) and chemoselective activations (armed SEt disaccharide intermediate over disarmed acceptor 228), as well as a convergent approach (4 + 3, building block 229), all in one pot.
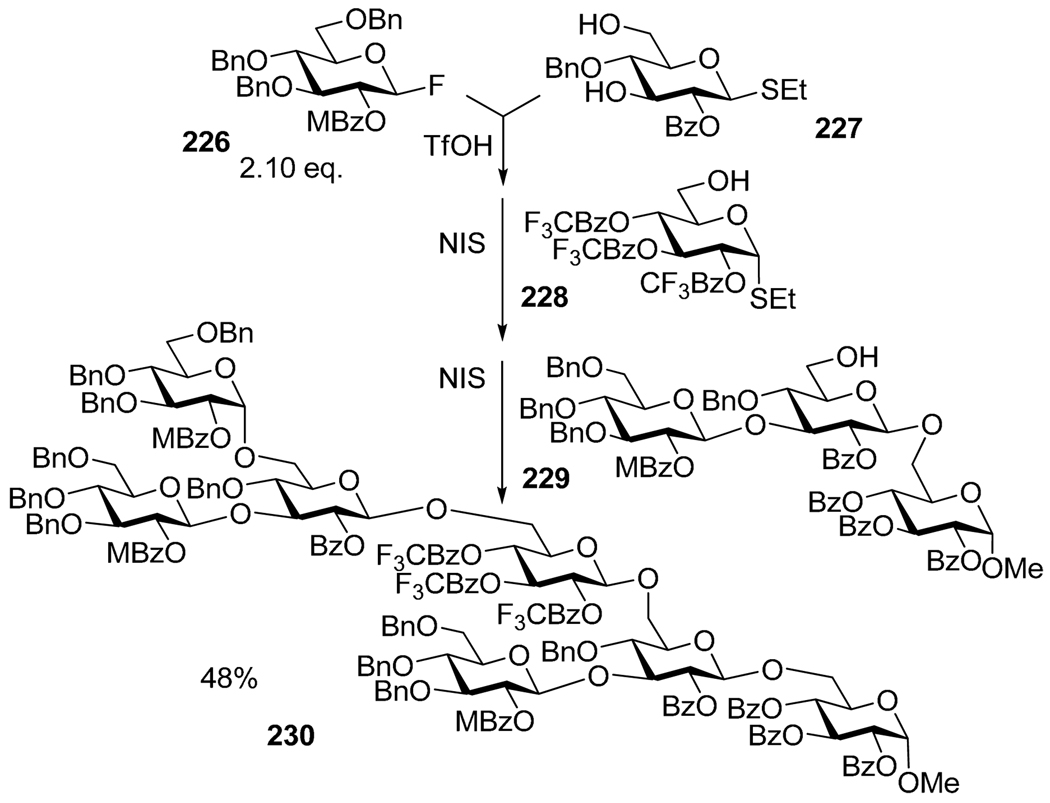
Mukaiyama’s one-pot synthesis involving the elements of selective, chemoselective and convergent concepts.
A similar target was obtained by Takahashi in a six-step one-pot synthesis of heptasaccharide using a Quest 210 manual synthesizer.164 As depicted in Scheme 46, this synthesis required the sequential addition of seven reaction components 231–237 equipped with four different leaving groups. Sequential activations employed herein include: activation of glycosyl bromide 231 (activated with AgOTf over S-ethyl acceptor), thioglycosides 232 and 233 (with MeOTf over fluoride acceptor 234), tetrasaccharide fluoride (with Cp2HfCl2/AgOTf over S-phenyl acceptor 235) and phenyl thioglycosides 236 and hexasaccharide (with DMTST in the armed-disarmed fashion). The resulting protected phytoalexin elicitor heptasaccharide 238 was isolated in 24% yield over six steps. To the best of our knowledge, this is the only selective activation-based synthesis that incorporates the elements of the orthogonal activations in one-pot (steps three and four).
7.3 Polymer-supported synthesis
Solid-phase synthesis has been developed and widely utilized in a routine preparation of oligopeptides and oligonucleotides, and is attracting renewed attention in connection with combinatorial chemistry.165 The use of polymer supports in oligosaccharide synthesis has also been attractive and several intriguing approaches have been reported.166,167 Most of the known syntheses involve glycosyl acceptor-bound approach, but examples involving glycosyl donor bound strategies that allow for selective activations have also emerged. An example of a donor-bound approach utilizing selective activations on solid phase synthesis is the synthesis of the Leb blood group antigen reported by Danishefsky and co-workers (Scheme 47).168 Glycal connected to Merrifield’s resin 239 was subjected to epoxidation with DMDO, followed by coupling with glycal acceptor 240 in the presence of ZnCl2. Fucosyl fluoride donor 241 was then activated over polymer-bound glycal 242 in the presence of Sn(OTf)2 and DTBP. These steps incorporate the elements of a two-directional concept developed by Boons.169,170 The resulting tetrasaccharide glycal 243 was then converted into the corresponding 2-amino thioglycoside 244 that was coupled with glycal acceptor 255. The resulting pentasaccharide was then cleaved off of the polymer support to afford pentasaccharide 246 in 20% over-all. Selective activation of the SBox bound glycosyl donor over solution phase thioglycoside acceptor in the presence of TMSOTf was also reported.171
The application of the orthogonal strategy utilizing polymer support was described by Ogawa.13 To illustrate this concept, a recent representative example is depicted in Scheme 48.172 According to this approach, polymer-bound glycosyl donor 247 was activated selectively over fluoride acceptor 15 in the presence of DMTST. The resulting disaccharide 248 was activated over S-phenyl acceptor 249 in the presence of Cp2Hf(OTf)2. Finally, S-phenyl trisaccharide 250 was glycosidated with octanol in the presence of DMTST.
7.4 Ionic liquid supported synthesis
A promising technique for the tagged oligosaccharide synthesis that makes use of an ionic-liquid support has recently emerged.173,174 As with the polymer-supported166,167 and fluorous tag-supported175,176 syntheses, ionic liquid-supported assembly expedites oligosaccharide synthesis by eliminating the need for chromatographic purification of the intermediates. Herein, this approach is illustrated by an example that incorporates elements of orthogonal strategy making use of alternating activations of STol and F leaving groups and convergent approach (Scheme 49).177,178 (1-Methylimdazoliumhexafluorophospho)acetyl ionic liquid tag is readily introduced via the corresponding 6-chloroacetylated starting material by reaction with N-methylimidazole and potassium hexafluorophosphate.
Very recently, Gouhier and co-workers179 reported efficient 1,2-cis-glycosylations using ionic liquid-supported thioglycoside building block 266 in a two-directional169,170 manner. First, thioglycoside 266 was employed as a glycosyl acceptor for the selective activation of trichloroacetimidate donor 265 in presence of TMSOTf (Scheme 50). The resulting α-(1→4)-linked disaccharide 267 was then used as the glycosyl donor for the coupling with acceptor 268 that resulted in the formation of trisaccharide 269.
8 Conclusions and future directions
It is critical to make complex carbohydrates more accessible to the general chemical, biochemical, and industrial audiences to keep pace with the exploding area of glycobiology. This can be only achieved by the development of methods and strategies for efficient oligosaccharide synthesis that would be applicable for both laboratory and industrial preparation. A number of excellent strategies that offer efficient routes to oligosaccharide assembly have already emerged. Important discoveries surveyed in this article have already allowed scientists to synthesize complex oligosaccharides and glycoconjugates for structural, biological, and medical studies. Nevertheless, carbohydrates of even moderate complexity still present a considerable challenge, even to experts. No expeditious strategy developed to date can offer an easy and reliable access to the full range of compounds of interest to glycoscientists. Some strategies offer a very efficient approach to a certain sequence, but in general each target still requires careful selection of methods, conditions, and strategies. Therefore, examples of conventional oligosaccharide synthesis using additional protecting/leaving group manipulations are still abundant.
The development of efficient and general methods for expeditious synthesis of complex carbohydrates will undoubtedly remain an important and active arena for scientific endeavors during the 21st century. In the coming years, glycoscientists are expected to have developed more simple, efficient, and flexible approaches to oligosaccharide assembly that will complement existing methodologies and bring our ability to obtain complex oligosaccharides to a significantly higher level. The recent innovations surveyed herein make contemporary glycoscientists well positioned to undertake further studies. Future developments may be expected to incorporate existing methodologies, and combinations thereof, while blending in new concepts and technologies.
Acknowledgements
The authors are indebted to the National Institute of General Medical Studies (Award #GM077170) for providing generous support for their research efforts in the area of expeditious oligosaccharide synthesis. SK is grateful to the UM - St. Louis Graduate School for awarding him with the Dissertation Fellowship.
Footnotes
Publisher's Disclaimer: This is a PDF file of an unedited manuscript that has been accepted for publication. As a service to our customers we are providing this early version of the manuscript. The manuscript will undergo copyediting, typesetting, and review of the resulting proof before it is published in its final citable form. Please note that during the production process errors may be discovered which could affect the content, and all legal disclaimers that apply to the journal pertain.
References
Full text links
Read article at publisher's site: https://doi.org/10.1016/j.carres.2011.05.004
Read article for free, from open access legal sources, via Unpaywall:
https://europepmc.org/articles/pmc3129461?pdf=render
Citations & impact
Impact metrics
Article citations
Activation of thioglycosides under mild alkylation conditions.
Carbohydr Res, 531:108872, 10 Jun 2023
Cited by: 0 articles | PMID: 37348387 | PMCID: PMC10528260
Synthesis and Glycosidation of Anomeric Halides: Evolution from Early Studies to Modern Methods of the 21st Century.
Chem Rev, 122(13):11701-11758, 08 Jun 2022
Cited by: 10 articles | PMID: 35675037 | PMCID: PMC9417321
Review Free full text in Europe PMC
Progress and challenges in the synthesis of sequence controlled polysaccharides.
Beilstein J Org Chem, 17:1981-2025, 05 Aug 2021
Cited by: 6 articles | PMID: 34386106 | PMCID: PMC8353590
Review Free full text in Europe PMC
Metal-free glycosylation with glycosyl fluorides in liquid SO2.
Beilstein J Org Chem, 17:964-976, 29 Apr 2021
Cited by: 2 articles | PMID: 33981367 | PMCID: PMC8093551
Palladium(II)-assisted activation of thioglycosides.
Org Biomol Chem, 19(9):2044-2054, 01 Mar 2021
Cited by: 4 articles | PMID: 33599667 | PMCID: PMC8142060
Go to all (27) article citations
Similar Articles
To arrive at the top five similar articles we use a word-weighted algorithm to compare words from the Title and Abstract of each citation.
Superarmed and superdisarmed building blocks in expeditious oligosaccharide synthesis.
Top Curr Chem, 301:189-221, 01 Jan 2011
Cited by: 16 articles | PMID: 21120713 | PMCID: PMC3150466
Review Free full text in Europe PMC
S-Benzimidazolyl (SBiz) Imidates as a Platform for Oligosaccharide Synthesis via Active-Latent, Armed-Disarmed, Selective, and Orthogonal Activations.
J Org Chem, 82(4):1904-1911, 07 Feb 2017
Cited by: 4 articles | PMID: 28135419 | PMCID: PMC5498158
S-thiazolinyl (STaz) glycosides as versatile building blocks for convergent selective, chemoselective, and orthogonal oligosaccharide synthesis.
Chemistry, 12(25):6630-6646, 01 Aug 2006
Cited by: 31 articles | PMID: 16800023
Cap and capture-release techniques applied to solid-phase synthesis of oligosaccharides.
J Org Chem, 71(18):7067-7070, 01 Sep 2006
Cited by: 6 articles | PMID: 16930066
Funding
Funders who supported this work.
NIGMS NIH HHS (3)
Grant ID: R15 GM077170
Grant ID: GM077170
Grant ID: R15 GM077170-02