Abstract
Free full text

A dual role of H4K16 acetylation in the establishment of yeast silent chromatin
Abstract
Discrete regions of the eukaryotic genome assume heritable chromatin structure that is refractory to transcription. In budding yeast, silent chromatin is characterized by the binding of the Silent Information Regulatory (Sir) proteins to unmodified nucleosomes. Using an in vitro reconstitution assay, which allows us to load Sir proteins onto arrays of regularly spaced nucleosomes, we have examined the impact of specific histone modifications on Sir protein binding and linker DNA accessibility. Two typical marks for active chromatin, H3K79me and H4K16ac decrease the affinity of Sir3 for chromatin, yet only H4K16ac affects chromatin structure, as measured by nuclease accessibility. Surprisingly, we found that the Sir2-4 subcomplex, unlike Sir3, has higher affinity for chromatin carrying H4K16ac. NAD-dependent deacetylation of H4K16ac promotes binding of the SIR holocomplex but not of the Sir2-4 heterodimer. This function of H4K16ac cannot be substituted by H3K56ac. We conclude that acetylated H4K16 has a dual role in silencing: it recruits Sir2-4 and repels Sir3. Moreover, the deacetylation of H4K16ac by Sir2 actively promotes the high-affinity binding of the SIR holocomplex.
Introduction
Heterochromatin is a heritable, specialized chromatin structure that silences discrete regions in eukaryotic genomes. Among other features, gene silencing within heterochromatic regions is thought to involve compaction of the chromatin fibre in order to structurally limit DNA accessibility. In budding yeast, silent chromatin requires the binding of Silent Information Regulatory (Sir) proteins to unmodified nucleosomes. Work of many laboratories has identified Sir2, Sir3 and Sir4 proteins as the core components of silent chromatin at telomeres and silent mating type loci (Rine and Herskowitz, 1987; reviewed in Rusche et al (2003)). Two-hybrid and protein binding studies suggested that they form a complex, with Sir4 being a scaffold protein that bridges between Sir2 and Sir3 (Moazed et al, 1997; Strahl-Bolsinger et al, 1997; Rudner et al, 2005; Cubizolles et al, 2006). Although initial attempts to purify the Sir proteins from yeast yielded only an Sir2-4 heterodimer (Ghidelli et al, 2001; Hoppe et al, 2002), a stable Sir2-Sir3-Sir4 heterotrimer with 1:1:1 stoichiometry (hereafter SIR complex) was purified from insect cells (Cubizolles et al, 2006). A fourth Sir protein, Sir1, is important for the establishment of silencing at the silent mating type loci, but is not required for repression at telomeres (Pillus and Rine, 1989; Aparicio et al, 1991).
Sir proteins do not bind DNA in a sequence-specific manner, yet zones of silencing are restricted to specific domains in the yeast genome. To achieve targeted silencing, Sir proteins are recruited by bifunctional DNA binding factors, such as Rap1, Abf1 and Orc1, which bind yeast silencer elements. The SIR complex then spreads from this nucleation site for 3–20 kb, depending on the abundance and balance of available Sir proteins (reviewed in Gasser and Cockell (2001) and Rusche et al (2003)). SIR complex association decreases the ability of enzymes, like DNA methylases or endonucleases, to access the DNA (Gottschling, 1992; Loo and Rine, 1994). Transcription in these regions is repressed, most likely by reducing RNA polymerase II occupancy at promoters (Chen and Widom, 2005; Lynch and Rusche, 2009), although other studies suggest that Sir protein binding interferes with RNA polymerase II elongation (Sekinger and Gross, 2001; Gao and Gross, 2008).
All three Sir proteins, Sir2, Sir3 and Sir4, are essential for transcriptional repression. Sir3 and Sir4 are primarily thought to be structural proteins of silent chromatin (Gasser and Cockell, 2001). SIR3 arose from a duplication of the ORC1 gene, with which it shares an N-terminal BAH domain and a related AAA+ ATPase domain (Hickman and Rusche, 2010). Sir4 is found only in related ascomycetes species, while Sir2 is a NAD-dependent histone deacetylase conserved from bacteria to man. Its enzymatic activity is required for gene silencing (Tanny et al, 1999; Imai et al, 2000; Smith et al, 2000).
The key substrate of Sir2 is histone H4 acetylated on lysine 16 (H4K16ac; Imai et al, 2000; Smith et al, 2000; Borra et al, 2004). This mark is found on transcriptionally active chromatin in most species and marks early firing origins in yeast and flies (Kimura et al, 2002; Suka et al, 2002; Schwaiger et al, 2010). It has been shown that unmodified H4K16 promotes compaction of the chromatin fibre in vitro and in vivo (Smith et al, 2003; Shogren-Knaak et al, 2006; Robinson et al, 2008). Consistently, in budding yeast H4K16ac is found throughout the genome except at silent loci (Suka et al, 2001; Smith et al, 2003).
Recombinant fragments of Sir3 and Sir4 were shown to bind to the histone H4 tail in vitro, in a manner sensitive to mutations near K16 and to lysine acetylation (Hecht et al, 1995; Carmen et al, 2002). Using recombinant proteins and nucleosomal substrates, it was found that the H4K16A mutation can decrease binding of Sir3 in vitro (Johnson et al, 2009), while mutations of H4K16 to glycine or glutamate, and to a lesser extent arginine, diminished mating efficiency in vivo (Johnson et al, 1990; Megee et al, 1990; Park and Szostak, 1990). Finally it was shown that the H4K16G phenotype could be suppressed by a compensatory mutation in Sir3, suggesting that Sir3 contacts the H4 tail in an acetylation-sensitive manner (Johnson et al, 1990).
In yeast, H4K16 is acetylated primarily by the histone acetyltransferase (HAT) Sas2 (Kimura et al, 2002; Suka et al, 2002) and secondarily by the essential HAT Esa1, which also targets H4K5 and H4K12 (Suka et al, 2001, 2002; Chang and Pillus, 2009). Similar to conservative mutations in H4K16, the deletion of SAS2 impairs repression of a reporter gene at telomeres or the HML locus, although the same mutation favours silencing of a reporter at HMR, which has much stronger silencer elements (Reifsnyder et al, 1996; Ehrenhofer-Murray et al, 1997; Meijsing and Ehrenhofer-Murray, 2001). However, the rate of Sir3 recruitment to HMR was slower in cells that lack Sas2 (Katan-Khaykovich and Struhl, 2005), as was the establishment of silencing at HML (Osborne et al, 2009). This, together with the observation that the catalytic activity of Sir2 is required for silencing (Tanny et al, 1999; Imai et al, 2000; Smith et al, 2000; Yang and Kirchmaier, 2006; Yang et al, 2008a), suggests that Sir-mediated deacetylation of H4K16ac might have an active role in the formation of silent chromatin.
The H4K16ac mark is also required for efficient methylation of lysine 79 on the histone H3 (H3K79me) by the methyltransferase Dot1 (Altaf et al, 2007). In vivo H3K79me appears to impair the spreading of the Sir proteins, and is thought to act by reducing association of Sir3 to chromatin (Ng et al, 2002, 2003; van Leeuwen et al, 2002; Altaf et al, 2007; Onishi et al, 2007). Consistently, a recent study with recombinant proteins has suggested that both Sir3 and the SIR holocomplex have lower affinities for reconstituted chromatin bearing H3K79me (Martino et al, 2009). This shows that, in addition to the histone tails, the Sir3 protein also interacts with the nucleosomal core, a property that has been assigned both to the N-terminal BAH domain (Onishi et al, 2007; Buchberger et al, 2008; Norris et al, 2008; Sampath et al, 2009) as well as the Sir3 C-terminal region (Altaf et al, 2007).
A further histone modification that interferes with SIR-mediated repression is the acetylation of K56 on histone H3. In budding yeast, H3K56ac is deposited by Rtt109 during S phase before the loading of the histones onto DNA, and therefore serves as a marker for newly assembled nucleosomes (Hyland et al, 2005; Masumoto et al, 2005; Han et al, 2007; Li et al, 2008). A large number of studies have also linked H3K56ac to gene transcription from yeast to man (Xu et al, 2005, 2007; Schneider et al, 2006; Williams et al, 2008; Yang et al, 2008b; Michishita et al, 2009; Xie et al, 2009). In yeast, amino-acid substitutions at H3K56 severely disrupt silencing without completely displacing the SIR complex (Xu et al, 2007; Yang et al, 2008b). Moreover, elimination of the histone deacetylases responsible for removal of H3K56ac, Hst3 and Hst4, disrupts SIR-mediated repression as well (Yang et al, 2008b). A recent report has shown that acetylation of H3K56 favours transcriptional elongation through yeast heterochromatin, generating speculation that H3K56ac promotes the displacement of the Sir proteins (Varv et al, 2010). However, there is as yet no direct evidence that the affinity of Sir proteins for nucleosomes is lowered by H3K56ac.
To gain insight into the role played by these histone modifications in the assembly of silent chromatin, we reconstituted SIR-bound chromatin in vitro using nucleosomes homogeneously modified on only one residue. Our system recapitulates many of the characteristics of silent chromatin (Martino et al, 2009) and allows us to probe both Sir protein binding and accessibility of the linker DNA to micrococcal nuclease (MNase). We find that both H3K79me and H4K16ac decrease the affinity of Sir3 for chromatin, while only H4K16ac has an effect on MNase accessibility. Surprisingly, we found that Sir2-4 prefers to bind to chromatin acetylated on H4K16. The binding of Sir2-4, in presence of NAD and Sir3, leads to the removal of the H4K16ac mark and couples stable binding of the Sir2-3-4 complex with a significant decrease in linker DNA accessibility. On the other hand, H3K56ac slightly increases MNase accessibility and reduces the interaction of chromatin with the SIR complex. Importantly, we find that H3K56ac is not a substrate for Sir2-mediated deacetylation. We, thus, show how the anti-silencing properties of different histone modifications differentially affect silent chromatin. Of particular interest are the two contradictory roles played by H4K16ac, which reduces the binding of Sir3 and favours the recruitment of Sir2-4. The acetylation and deacetylation of H4K16 thus appear to orchestrate the sequential binding of Sir proteins in order to establish a stable silent chromatin.
Results
The H4K16ac mark differentially affects the binding of Sir2-4 and Sir3 to chromatin
It is generally accepted that the H4K16ac mark plays an important role in silent chromatin by preventing the ectopic spread of the Sir proteins from the non-acetylated silent domains (Kimura et al, 2002; Suka et al, 2002; Millar et al, 2004; Yang et al, 2008a). However, accumulating evidence suggests that not only the absence of the acetyl mark but its Sir2-dependent removal may be required for efficient establishment of silencing (Liou et al, 2005; Yang and Kirchmaier, 2006; Yang et al, 2008a; Martino et al, 2009; Osborne et al, 2009). To shed light on this matter, we analysed in detail the binding of SIR subcomplexes to nucleosomal arrays bearing a fully acetylated H4K16.
Nucleosomes were reconstituted with recombinant histones that were either unmodified or fully acetylated on H4K16. These were generated by expressing a truncated version of H4 and adding the N-terminal tail by native chemical ligation (NCL) using a synthetic peptide (Shogren-Knaak et al, 2006; Supplementary Figure S1). Nucleosomal arrays were then reconstituted by salt dialysis using a DNA template containing repeated arrays of a 167-bp histone octamer positioning sequence (Widom 601) as described previously (Huynh et al, 2005; Martino et al, 2009). Recombinant Sir proteins were purified from insect cells (Figure 2F; Cubizolles et al, 2006; Martino et al, 2009).
We first compared acetylated and non-acetylated arrays of a 6mer of nucleosomes by monitoring the accessibility of linker DNA to MNase in the absence of Sir proteins. Previous studies have shown that acetylation of H4K16 inhibits chromatin compaction both in vitro and in vivo (Shogren-Knaak et al, 2006; Robinson et al, 2008). By challenging this chromatin with increasing amounts of MNase, we found that H4K16ac enhances linker DNA accessibility of a chromatin template as short as six nucleosomes (Figure 1A).
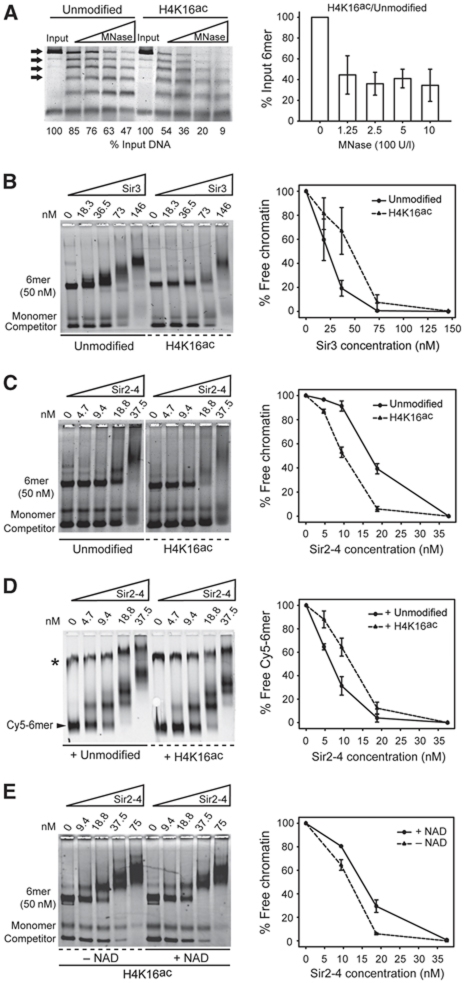
Acetylation of H4K16 decreases the binding affinity of Sir3 but favours the loading of Sir2-4 onto chromatin. (A) Equally saturated 6mer of 601 nucleosomes with either unmodified or acetylated H4K16 was digested with increasing amount of MNase, as indicated. After protein digestion, the denatured DNA was separated by electrophoresis and visualized by SYBR® Safe staining. The bands showed by an arrow (6-, 5-, 4- and 3mers) were quantified and normalized to input. The histograms show the ratio between the amount of intact 6-3mers of H4K16ac over unmodified chromatin for the indicated MNase titration point. The Sir3 protein (B) or the Sir2-4 heterodimer (C) were titrated into a constant amount of unmodified or H4K16ac 6mer of 601 nucleosomes. Samples were separated by native agarose gel electrophoresis and visualized by SYBR® Safe staining. (D) The binding of increasing amounts of Sir2-4 to 8 nM of unmodified Cy5-labelled 6mer of nucleosomes (indicated by the arrowhead) was competed with 32 nM of either unlabelled unmodified or unlabelled H4K16ac 6mer of nucleosomes. Cy5 fluorescence was used to monitor the binding of Sir2-4 to the unmodified labelled chromatin. The asterisk indicates a Cy5-labelled contaminant DNA. (E) The Sir2-4 heterodimer was titrated into a constant amount of H4K16ac 6mer of 601 nucleosomes. Deacetylation is allowed by the addition of 150 μM NAD where indicated. Samples were separated and visualized as in (B, C). The images are representative of at least three independent experiments, quantifications show the mean value±s.e.m. of the % of unbound chromatin compared with the input.
Sir3 has been reported to be more susceptible than Sir4 to modifications on histone tails (Carmen et al, 2002; Johnson et al, 2009). Therefore, we examined first the effect of H4K16ac on the binding of Sir3 to nucleosomal arrays. Increasing amounts of Sir3 were titrated onto unmodified or H4K16ac arrays of nucleosomes. The binding was analysed by gel shift and quantified by scoring the loss of the unbound 6mer. In agreement with previous studies (Carmen et al, 2002; Johnson et al, 2009), we found that H4K16ac reduces the binding affinity of Sir3 to an in vitro reconstituted nucleosomal array by roughly two-fold (Figure 1B). In contrast, the binding affinity of the Sir2-4 heterodimer to chromatin was increased nearly two-fold by the presence of the H4K16ac mark (Figure 1C). Superficially, this appears to contradict the fact that silent chromatin is depleted for this mark, although it is consistent with the notion that H4K16ac is a key substrate of Sir2-4 (see also Johnson et al (2009)). Therefore, we decided to perform competition experiments in order to reinforce this observation. The binding of increasing amounts of Sir2-4 to an unmodified Cy5-labelled array was competed with a four-fold excess of either unlabelled unmodified or unlabelled H4K16ac chromatin. Confirming our previous results, H4K16ac chromatin competed roughly two-fold more efficiently for the binding of Sir2-4 compared with unmodified chromatin (Figure 1D).
We previously showed that our recombinant Sir2-4 has efficient histone deacetylase activity in the presence of its co-factor NAD (Cubizolles et al, 2006). We reasoned that if Sir2-4 bound H4K16ac with higher affinity because it is a preferred substrate of Sir2, then the complex should have less affinity once H4K16ac had been deacetylated. To test this, we quantified the binding of the Sir2-4 heterodimer to H4K16ac chromatin in the absence or presence of NAD. Confirming our hypothesis, Sir2-4 bound more readily to acetylated chromatin and less readily following deacetylation (Figure 1E). Surprisingly, this shows an enhanced affinity of Sir2-4 for acetylated H4K16, while the opposite is true for Sir3.
Sir2-dependent deacetylation of H4K16ac stabilizes the association of Sir2-3-4 to chromatin
Removal of H4K16ac through the catalytic activity of Sir2 has been reported to be important for silencing (Johnson et al, 1990; Suka et al, 2001, 2002; Carmen et al, 2002; Kimura et al, 2002). However, it is not clear whether this is due exclusively to the generation of unmodified H4K16 or whether it additionally involves a conformational change coupled to O-acetyl-ADP-ribose (O-AADPR) production (Liou et al, 2005; Martino et al, 2009). To gain insight into the molecular consequences of H4K16ac deacetylation on the establishment of silencing, we compared the binding of the SIR complex with unmodified or H4K16ac chromatin in the presence or absence of NAD. We first examined the effect of H4K16ac on the binding of the Sir2-3-4 heterotrimer in absence of NAD (Figure 2A) and found that, similar to Sir2-4 (Figure 1C) but in a less pronounced manner, the SIR holocomplex bound slightly better to acetylated chromatin. We then confirmed that our purified Sir2-3-4 complex was able to efficiently deacetylate H4K16ac within chromatin in the presence of NAD (Figure 2E), as shown previously for chemically acetylated histone octamers (Cubizolles et al, 2006). In the following experiments, the term ‘deacetylated chromatin’ will be used whenever H4K16ac marks were actively removed by Sir2 in the presence of NAD, to distinguish it from chromatin assembled from unmodified histones.
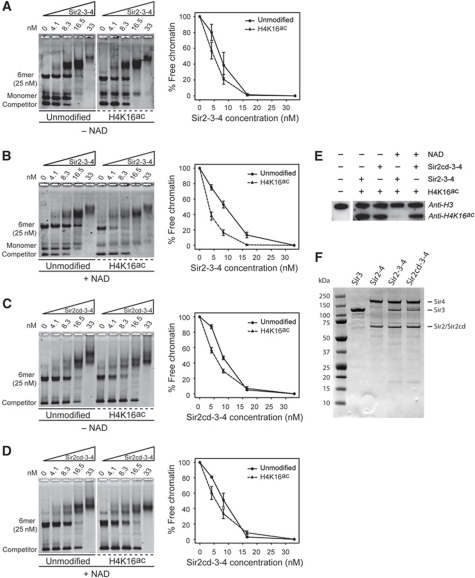
Sir2-dependent deacetylation of H4K16ac stabilizes the association of Sir2-3-4 to chromatin. The Sir2-3-4 complex (A, B) or the catalytically dead Sir2cd-3-4 mutant (C, D) was titrated into a constant amount of unmodified or H4K16ac 6mer of 601 nucleosomes. Where indicated, 150 μM NAD was added to the samples. Scatter plot quantifications show the mean value±s.e.m. of the % of unbound chromatin compared with the input for at least three experiments. (E) Reconstituted chromatin fully acetylated on H4K16 was subjected to NAD-dependent deacetylation in presence of a 2.5-fold molar excess of the Sir2-3-4 complex or the Sir2cd-3-4 mutant. The acetylation state was then determined by immunoblotting using acetylation mark-specific antibodies and H3 for loading. (F) Two micrograms of the indicated Sir protein were denatured in sample buffer and run on a 4–12% NuPAGE® Novex® Bis-Tris Gel.
We next compared the binding affinity of Sir2-3-4 with unmodified or H4K16ac chromatin in the presence of NAD. We found that the active removal of the H4K16ac mark increased the binding affinity of the SIR complex to chromatin by roughly two-fold (Figure 2B). This effect is not caused by NAD alone as enhanced binding was not observed when H4K16ac chromatin was replaced with unmodified chromatin (Supplementary Figure S2A). Indeed, in absence of Sir2, NAD does not affect the acetylation state of chromatin (Supplementary Figure S2B). This shows that the binding affinity of the SIR complex for deacetylated chromatin is higher compared with chromatin assembled from unmodified histones.
In order to reinforce this finding, we tested whether the deacetylase activity of Sir2 itself was required for the enhanced binding of acetylated template in the presence of NAD. We generated a catalytic inactive Sir2 (Sir2cd) by introducing the point mutation N345A, which maps to the nucleotide binding motif (Rossman fold). This mutation disrupts Sir2 enzymatic activity in vitro and in vivo (Imai et al, 2000; Armstrong et al, 2002). The N345A substitution, however, did not affect the stability of Sir2 or its interaction with Sir3 and Sir4 and we were able to purify the mutated Sir2cd-3-4 from insect cells with the same efficiency as for the Sir2-3-4 complex (Figure 2F). We could furthermore confirm that this mutant did not retain significant deacetylase activity (Figure 2E).
In order to confirm that the Sir2cd-3-4 mutant was still able to recognize its substrate, we monitored the binding of Sir2cd-3-4 to unmodified or H4K16ac chromatin in the absence of NAD. We found that, like Sir2-3-4, Sir2cd-3-4 has a slight preference for H4K16ac chromatin (compare Figure 2A and C). We then monitored the loading of the Sir2cd-3-4 mutant onto unmodified or H4K16ac chromatin in the presence of NAD. Unlike the Sir2-3-4 complex, the catalytic dead Sir2cd-3-4 showed the same slight preference for the H4K16ac chromatin, as it did in the absence of NAD (compare Figure 2C and D). This data reinforce our observation that the deacetylation reaction has a positive role on the loading of the SIR complex onto chromatin (Figure 2B). Given that the deacetylation of H4K16ac chromatin reduced the binding affinity of the Sir2-4 heterodimer (Figure 1E), these results suggest that Sir3 and the deacetylation of H4K16ac by Sir2 jointly promote the binding of the SIR holocomplex to chromatin.
The Sir3 protein and Sir2-dependent deacetylation of H4K16ac are both required to decrease nuclease accessibility of the linker DNA
SIR complex bound chromatin is thought to have a more compact structure in vivo as it is less accessible to enzymatic attack (Gottschling, 1992; Loo and Rine, 1994). The SIR complex could compact chromatin in two ways: first by deacetylating H4K16, and second by binding to chromatin. We observed that loading of the SIR complex onto unmodified chromatin greatly reduces the accessibility of the linker DNA to MNase and the restriction enzyme AvaI in vitro, consistent with a direct role for binding (Supplementary Figure S3A and B; Martino et al, 2009). In order to test the impact of H4K16ac deacetylation on compaction in vitro, we first incubated H4K16ac chromatin with the Sir2-4 subcomplex in the presence or absence of NAD and then challenged it with increasing amounts of MNase. We found that the presence of NAD did not significantly change the accessibility of the linker DNA (Figure 3A). Since acetylation of chromatin usually results in greater accessibility (Figure 1A), this result is likely a combination of changed accessibility due to removal of H4K16ac and reduced binding affinity of Sir2-4 for deacetylated chromatin (Figure 1E).
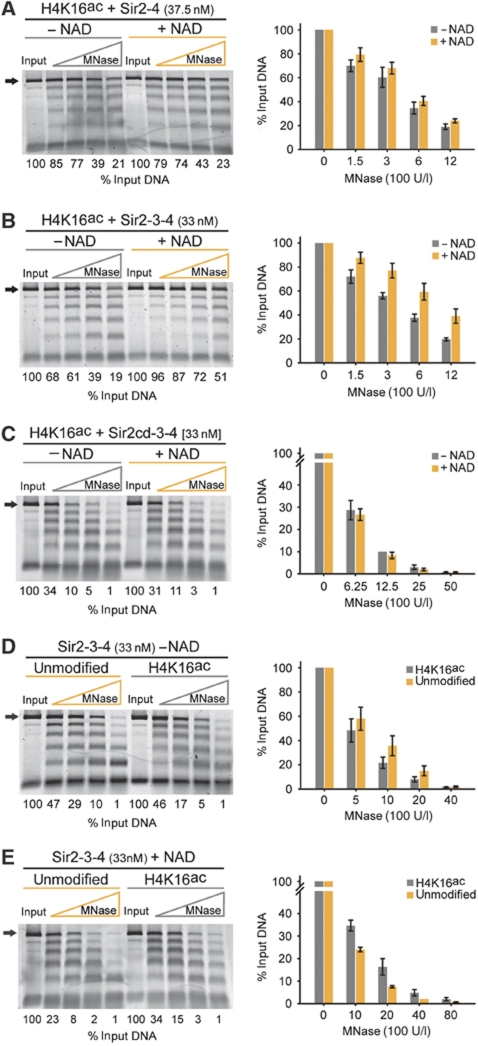
Sir3 is required to translate the Sir2-dependent deacetylation of H4K16ac into a decrease of nuclease accessibility of the linker DNA. Unmodified or H4K16ac 6mer of 601 nucleosomes (50 nM) was incubated with the indicated amount of Sir2-4 (A), Sir2-3-4 (B, D and E) or Sir2cd-3-4 (C) and was challenged with increasing amounts of MNase. Where indicated, SIR-bound chromatin was supplemented with 150 μM NAD and incubated for 15 min at 30°C before MNase digestion. Deproteinated samples were separated by electrophoresis and the amount of intact 6mer DNA (black arrow) was quantified and normalized to input. Quantification of at least three experiments was used to generate the vertical bar charts, data represent mean value±s.e.m.
We then performed the same experiment but replaced Sir2-4 with the SIR holocomplex. The concentration of Sir2-3-4 used resulted in a complete upshift of both acetylated and unmodified chromatin in a binding assay, ruling out differential accessibility due to incomplete ligand occupancy. Interestingly, we observed that in the presence of NAD the linker DNA was more protected from MNase than SIR-bound chromatin in the absence of NAD (Figure 3B). Importantly, the addition of NAD did not change the protection of linker DNA of an array bound by the catalytic inactive Sir2cd-3-4 (Figure 3C). The same analysis in the absence of Sir3 did not increase protection against MNase attack (Figure 3A), arguing that the protective effect of NAD-dependent deacetylation of H4K16ac by Sir2 requires Sir3 (compare Figures 3A and B). Finally, the increased linker DNA protection observed in Figure 3B was not caused by the NAD molecule per se, as no NAD-dependent differences were scored for linker DNA accessibility when Sir proteins were bound to unmodified chromatin (Supplementary Figure S3C). These results suggested that the deacetylation of H4K16ac by the SIR complex promotes linker DNA protection.
When comparing unmodified with acetylated chromatin bound by Sir2-3-4 in the absence of NAD, we found that the linker DNA is slightly more protected (Figure 3D), indicating that at least some of the protection observed in Figure 3B is due to loss of H4K16ac per se. However, given our previous observation that deacetylated chromatin is bound with higher affinity than unmodified chromatin (Figure 2B), we decided to explore the possibility that the deacetylation reaction itself may also contribute to the increased linker DNA protection observed in Figure 3B. Therefore, we compared the linker DNA accessibility of unmodified and H4K16ac chromatin in the presence of Sir2-3-4 and NAD. We found that deacetylated chromatin is reproducibly more protected from MNase attack compared with chromatin assembled from unmodified histones (Figure 3E). Together, these results show that both Sir2-dependent deacetylation of H4K16ac and Sir3 are required to decrease the nuclease accessibility of linker DNA, which presumably reflects the tighter binding of the SIR holocomplex to chromatin. In addition, there may be a conformational change that enhances linker DNA protection.
H3K56ac loosens Sir protein binding to chromatin, slightly increasing linker DNA accessibility
To ask if our observation for H4K16ac can be generalized to other acetylation marks we tested the effects of H3K56 acetylation, which is found on newly assembled nucleosomes in S phase. Since there are contradictory reports about which enzyme deacetylates H3K56ac (Xu et al, 2007; Yang et al, 2008b) we first tested if Sir2 can deacetylate H3K56ac as suggested earlier. We incubated H3K56ac chromatin with SIR complex in the presence or absence of NAD. Chromatin homogenously acetylated at H3K56 was obtained by purifying acetylated H3 from E. coli using an expanded genetic code strategy (Neumann et al, 2008). Probing the histones with H3K56ac antibodies after incubation showed that, unlike for H4K16ac, the level of H3K56 acetylation remained unchanged (Figure 4B). We conclude that H3K56ac is not a substrate of the NAD-dependent deacetylase activity of Sir2. This supports previous work reporting that two Sir2-related enzymes, Hst3 and Hst4, are required for H3K56ac deacetylation in vivo (Celic et al, 2006; Maas et al, 2006; Yang and Kirchmaier, 2006) and suggests that Hst3 and Hst4 are the exclusive deacetylases for this residue.
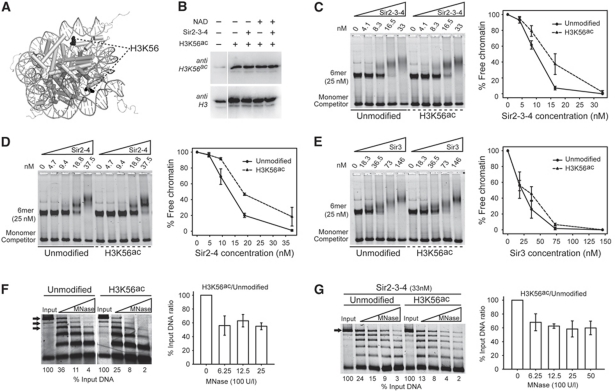
H3K56ac decreases Sir protein binding affinity and slightly increases linker DNA accessibility. (A) Cartoon representation of the nucleosome core particle (NCP147; Davey et al, 2002) highlighting the position of H3K56 (black) at the entry/exit point of the DNA around the histone octamer. (B) Reconstituted chromatin fully acetylated on H3K56 was subjected to NAD-dependent deacetylation in presence of a 2.5-fold molar excess of the SIR complex. The acetylation state was then determined by immunoblotting using acetylation mark-specific antibodies and H3 for loading. The SIR complex (C), Sir2-4 heterodimer (D) or Sir3 (E) were titrated into a constant amount of unmodified or H3K56ac 6mer of 601 nucleosomes. Samples were analysed as in Figure 2. Unmodified or H3K56ac 6mer of nucleosomes were challenged with an increasing amount of MNase in absence (F) or presence (G) of the SIR complex. The 6mer, 5mer and 4mer bands (F) or the band corresponding to the intact 6mer alone (G), shown by black arrows, were quantified and normalized to the input. The histograms show the ratio between the amounts of quantified DNA from H4K16ac chromatin over unmodified for the indicated MNase titration point±s.e.m.
To address whether acetylation of H3K56 has an effect on Sir protein loading, we compared the binding of the SIR holocomplex with unmodified and H3K56ac chromatin. We found that H3K56ac reduces the affinity of the SIR holocomplex for chromatin by roughly two-fold (Figure 4C). The binding affinity of the Sir2-4 heterodimer was also reduced in presence of the H3K56ac mark (Figure 4D), while the binding affinity of the Sir3 protein alone was mostly unchanged (Figure 4E). To explore whether the slight affinity decrease observed here for the SIR complex could be responsible for the silencing defects seen in vivo, we investigated whether the SIR complex efficiently protects linker DNA in chromatin bearing the H3K56ac mark. The acetylation on H3K56 per se has been shown to increase transient unwrapping of the DNA from the histone octamer but not to change the higher order structure of a 61mer nucleosomal array (Neumann et al, 2009). Consistently we show, by means of an MNase digestion assay, a slight increase in linker DNA accessibility for the chromatin bearing H3K56ac over the unmodified control (Figure 4F). Subsequently, after adding the SIR complex in saturating concentrations (Supplementary Figure S4A), the H3K56ac chromatin continued to show slightly higher linker DNA accessibility as compared with unmodified chromatin (Figure 4G). This is consistent with a previous in vivo study indicating that H3K56ac chromatin is more sensitive to DNA methylation by an ectopically expressed bacterial dam methylase (Xu et al, 2007). Moreover, H3K56 point mutations disrupted silencing at telomeres without affecting Sir protein spreading (Xu et al, 2007). We conclude that H3K56ac does not have a role similar to that of H4K16ac, neither in the recruitment of Sir2-4, nor by being a substrate for Sir2.
Methylation of H3K79 by Dot1 neither increases linker DNA accessibility nor reduces Sir2-4 loading
Another mark associated with active chromatin in yeast is methylation of lysine 79 of histone H3 (H3K79). This methylation is exclusively catalysed by Dot1 and is thought to act as a boundary for the inappropriate spreading of the Sir proteins on chromatin (van Leeuwen et al, 2002; Frederiks et al, 2008; Martino et al, 2009; Verzijlbergen et al, 2009). Moreover, in vitro studies showed that interaction of the Sir3 protein with histone peptides was sensitive to the methylation of H3K79 (Altaf et al, 2007; Onishi et al, 2007). Previous work from our laboratory showed that we can make use of recombinant Dot1 in order to methylate reconstituted nucleosomal arrays in vitro (Martino et al, 2009). We have previously shown that even partial methylation of H3K79 decreases the binding affinity of both the SIR complex and the Sir3 protein alone to chromatin (Martino et al, 2009). We now provide further evidence that the lowered affinity indeed affects Sir3 binding, since the Sir2-4 heterodimer associates with unmodified and H3K79me chromatin with nearly equal affinity (Figure 5A), while Sir3 clearly prefers unmodified chromatin (Figure 5B).
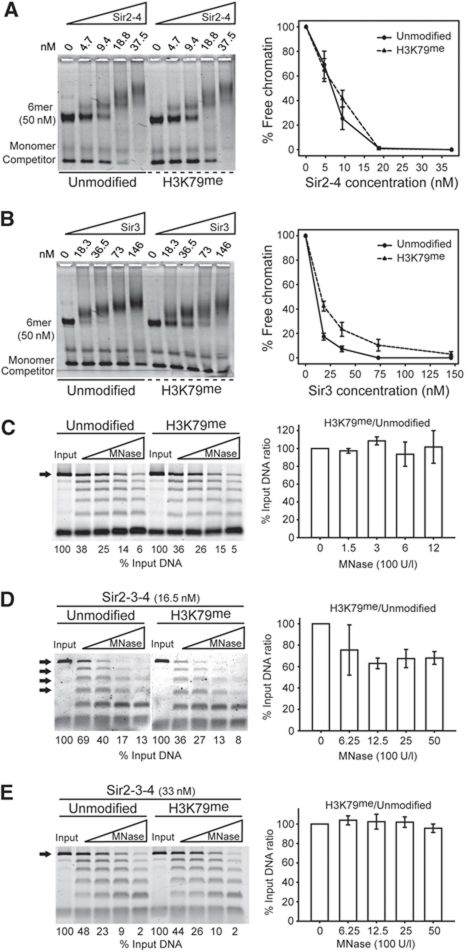
Methylation of H3K79 by Dot1 affects neither linker DNA accessibility nor Sir2-4 loading onto chromatin. The Sir2-4 heterodimer (A) or Sir3 (B) was titrated into a constant amount of unmodified or H3K79me 6mer of nucleosomes. Samples were analysed as in Figure 2. Unmodified or H3K79me chromatin were challenged with an increasing amount of MNase in absence (C) or presence (D, E) of the indicated amount of the SIR complex. The 6mer DNA band alone (C, E) or the 3mer to 6mer bands (D), shown by black arrows, were quantified and normalized to the input. The histograms show the ratio between the amount of quantified DNA from H3K79me over unmodified chromatin for the indicated MNase titration point.
We then decided to test whether H3K79me also impacts the structure of SIR-bound or SIR-depleted chromatin. To examine the potential impact of H3K79me on linker DNA protection, we challenged in vitro methylated chromatin lacking Sir proteins with increasing amounts of MNase. Unlike the case for H3K56ac, the accessibility of the linker DNA in the absence of SIR complex was unaffected by H3K79me (Figure 5C). However, in the presence of substoichiometric amounts of Sir2-3-4 (Supplementary Figure S4B), the accessibility of the linker DNA was higher for H3K79me chromatin than for unmodified chromatin (Figure 5D), consistent with notion that better SIR complex binding enhances linker DNA protection. When we added additional Sir2-3-4 such that we score an equal degree of binding on both substrates (Supplementary Figure S4C), we observed no difference in accessibility of linker DNA (Figure 5E). This suggests that H3K79me neither changes chromatin structure nor prevents the SIR complex from compacting it, but decreases the affinity of the SIR complex for chromatin. Thus, it antagonizes silencing through a mechanism distinct from H3K56ac.
Discussion
Silent chromatin in Saccharomyces cerevisiae is the best studied system of heterochromatic gene silencing, yet we still do not fully understand the molecular mechanisms of its assembly and the role of histone modifications in this process. In vitro binding analysis between Sir protein domains and histone peptides have been informative, yet they only reflect a small part of the chromatin template. To examine the molecular basis of SIR-dependent silencing, we have established a fully recombinant system that recapitulates key features of silent chromatin in budding yeast (Martino et al, 2009). Here, we extend this system to examine how histone modifications participate in the formation of stable silent and active domains.
We show here that the H4K16ac mark has both a positive and a negative role in SIR binding in a sequential manner (see Figure 6). Importantly, we show that H4K16ac decreases the binding affinity of Sir3, but, in contrast, promotes the association of the Sir2-4 heterodimer to chromatin. Even the binding affinity of the SIR holocomplex is slightly increased by the presence of H4K16ac in the absence of NAD (see also Johnson et al (2009)). This result, while initially counterintuitive, helps elucidate the dual role of H4K16ac in heterochromatin formation. On one hand, H4K16ac prevents the dispersion of its key ligand, Sir3, into euchromatic chromatin. On the other hand, the high affinity of Sir2-4 for H4K16ac may help nucleate silent chromatin, since it is likely in yeast that the targeted nucleosomes are acetylated before SIR complex loading.
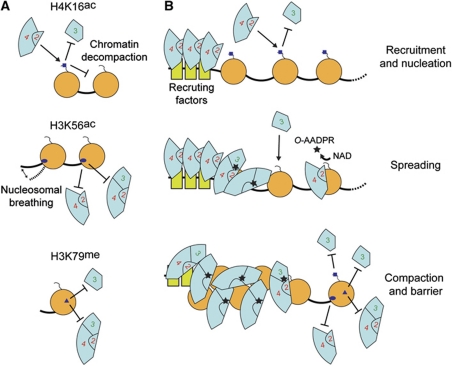
Combinatorial histone modifications distinguish silent and active chromatin regions. (A) Outline of the role played by different histone modifications on Sir protein loading and chromatin structure. (B) The Sir proteins are recruited onto chromatin by protein–protein interactions and bind tightly unmodified nucleosomes driving gene silencing. Spreading of the SIR complex is promoted by H4K16ac that recruits the Sir2-4 heterodimer yet prevents the ectopic spreading of Sir3 alone. The NAD-dependent deacetylation reaction of H4K16ac by Sir2 generates a high-affinity binding substrate for Sir3 and the synthesis of O-AADPR favours the tight association of the SIR complex to unmodified nucleosomes. H3K56ac and H3K79me generate a boundary to the spreading of the SIR complex mainly by reducing the binding affinity of the Sir2-4 heterodimer and the Sir3 protein, respectively. The acetylation of H3K56 and H4K16 also enhance the accessibility of the chromatin fibre, unlike methylation of H3K79.
In support of this dual role, it was shown that the substitution of H4K16 by not only an acetyl-mimicking residue, but also unacetylatable amino acids, disrupts silencing at telomeres and mating type loci (Johnson et al, 1990, 1992; Megee et al, 1990; Park and Szostak, 1990; Aparicio et al, 1991; Millar et al, 2004). Moreover, the deletion of SAS2, which encodes the HAT responsible for most H4K16ac in yeast, impaired the repression of reporter genes at certain loci, such as TelVIIL or within the HML locus (Reifsnyder et al, 1996; Meijsing and Ehrenhofer-Murray, 2001) and led to the spreading of Sir proteins into subtelomeric regions that usually lack SIR-mediated repression (Kimura et al, 2002; Suka et al, 2002). In contrast, the repression of a reporter at HMR was enhanced, probably because this locus has much stronger silencers, which dominate over an indiscriminate spreading of Sirs (Ehrenhofer-Murray et al, 1997). Importantly, both the kinetics of Sir3 recruitment to HMR and the establishment of silencing at HML were slower in cells that lack the H4K16-specific HAT, Sas2 (Katan-Khaykovich and Struhl, 2005; Osborne et al, 2009), suggesting a positive role for H4K16 acetylation. Collectively, these results support the model that Sas2-mediated acetylation of H4K16 has more than one role in silent chromatin formation (see also Zou and Bi (2008)). Reporter context appears to determine which role is rate limiting: the recruitment of Sir2-4, or the assembly and propagation of the Sir3-containing holocomplex along nucleosomes.
Sequential assembly of nuclease-resistant SIR-bound chromatin requires H4K16ac deacetylation
In vivo the absence of H4K16ac from silent chromatin suggests that it is removed by Sir2 as soon as the SIR complex is loaded. Moreover, it was shown that in the absence of Sir2 catalytic activity, H4K16ac prevents the formation of silent domains (Yang and Kirchmaier, 2006). On the other hand, as mentioned above, even conservative substitutions at H4K16 decrease silencing efficiency at HML and at telomeres (Johnson et al, 1990; Meijsing and Ehrenhofer-Murray, 2001; Yang and Kirchmaier, 2006). This supports the notion that not only the recruitment of Sir2-4 by H4K16ac, but the deacetylation reaction itself helps to seed repression (Johnson et al, 1992; Imai et al, 2000; Millar et al, 2004; Liou et al, 2005; Yang et al, 2008a; Martino et al, 2009).
Why is the deacetylation reaction important for silencing? The recapitulation of these steps in vitro helped us to address this question. Indeed, by adding NAD to SIR-bound H4K16ac chromatin, we catalysed deacetylation of H4K16 and increased the binding affinity of the SIR holocomplex to chromatin. This shows in a well-defined recombinant system that removal of the single H4K16ac mark by Sir2 increases SIR complex binding. Even more importantly, we found that the linker DNA was better protected from MNase digestion when the SIR complex was assembled on chromatin in the presence of H4K16ac and NAD, as compared with its being loaded onto unmodified chromatin. This protection nicely mimics the DNA shielding observed in SIR-silenced chromatin regions in vivo (Gottschling, 1992; Loo and Rine, 1994; Xu et al, 2007) and argues that the SIR complex may associate with chromatin in more than one conformation. It was previously proposed that a by-product of Sir2 NAD-dependent deacetylation, O-AADPR, might trigger a conformational change of the SIR–chromatin complex to favour repression (Liou et al, 2005; Onishi et al, 2007; Martino et al, 2009).
Importantly, the deacetylation-dependent increase in affinity of the SIR holocomplex for chromatin, and the increase in linker DNA protection, depends crucially on the presence of Sir3. This is consistent with previous results which showed that exogenously added O-AADPR enhances the binding of both Sir3 and the SIR holocomplex to chromatin (Martino et al, 2009). A further study showed that addition of an excess of acetylated peptides and NAD to SIR–chromatin assemblies generated a structure that appeared more compact by electron microscopy (Johnson et al, 2009), perhaps reflecting a conformational change in the SIR complex (Liou et al, 2005). Nevertheless, O-AADPR is probably neither absolutely required for SIR complex loading nor for silencing, since repression can be achieved in a strain devoid of NAD-dependent deacetylases if an ectopic HDAC is fused to Sir3 (Chou et al, 2008) or if Sir3 is overexpressed in a H4K16R background (Yang and Kirchmaier, 2006). Taken together, our data support a scenario in which the sequential loading of Sir2-4 onto nucleosomes containing H4K16ac, its NAD-dependent deacetylation and the loading of Sir3, sequentially promote a stable assembly that protects linker DNA from exogenous factors (Figure 6).
Boundary formation and reduction of SIR holocomplex affinity by histone modifications
The observation that H4K16ac might provide a boundary for heterochromatin spreading (Kimura et al, 2002; Suka et al, 2002) seems counterintuitive given the results described above. However, H4K16ac does affect other processes beyond SIR complex association to chromatin, most notably, the recruitment of the histone methyltransferase Dot1 to chromatin (Altaf et al, 2007). Given that Sir2-4 preferentially binds chromatin carrying H4K16ac, we propose that Dot1 competes with the recruitment of Sir2-4, and not as proposed earlier, with Sir3 (Altaf et al, 2007). On the other hand, the anti-silencing role of the methylation mark itself, H3K79me, is most likely a reflection of reduced interaction between Sir3 and methylated chromatin (Ng et al, 2002, 2003; van Leeuwen et al, 2002; Altaf et al, 2007; Onishi et al, 2007; Martino et al, 2009). Consistently, we found that nucleosomes bearing H3K79me neither affect the binding of the Sir2-4 heterodimer, nor was there an inherent change in structural properties of H3K79me-containing chromatin. This is consistent with crystallographic analyses which argue that H3K79me does not alter the structure of the nucleosome (Lu et al, 2008). Given that no enzyme has been found so far that removes the H3K79me mark, depletion of this mark may depend on histone eviction or on sequential dilution through rounds of DNA replication. Its slow removal renders H3K79me a more stable barrier to the spreading of the SIR complex than H4K16ac, which instead recruits Sir2-4 and promotes the spread of repression (Figure 6).
The third histone mark correlated with active chromatin in yeast is the acetylation of H3 on K56. In contrast with H3K79me, H3K56ac is clearly subject to active deacetylation. Here, we show that Sir2 is unable to remove the H3K56ac mark in vitro, which indirectly supports previous work showing that H3K56ac is primarily deacetylated by two Sir2-related enzymes: Hst3 and Hst4 (Celic et al, 2006; Maas et al, 2006). Indeed, Sir-mediated repression cannot be established in the absence of these two enzymes, although Sir proteins still bind telomeres in an hst3Δhst4Δ mutant (Yang et al, 2008b). Consistent with our work, this suggests that the H3K56ac mark does not completely block SIR–chromatin interaction.
How then does H3K56ac impair the formation of silent chromatin? Using our in vitro system, we found that H3K56ac affects both the affinity with which SIR complexes bind chromatin and the formation of a chromatin structure that is less accessible to MNase attack. The observed drop in affinity of SIR holocomplex for chromatin agrees with an in vivo study, which suggested that H3K56ac facilitates Sir protein displacement and RNA polymerase II elongation within heterochromatin regions (Varv et al, 2010). Our observation that H3K56ac increases accessibility of the linker DNA is consistent with an increase in spontaneous (but transient) unwrapping of the DNA from the histone octamer, which may reflect the position of H3K56 at the entry/exit point of the nucleosomal DNA (Figure 4A; Neumann et al, 2009). It is striking that even SIR-saturated arrays showed increased linker DNA accessibility in the presence of H3K56ac, indicating that SIR binding cannot overcome the effect of H3K56ac on nucleosomal structure. Although it is unclear why this modification reduces SIR complex binding, this and the increased linker DNA exposure are likely to account for the anti-silencing effect of the H3K56ac mark.
To conclude, we propose that the euchromatic mark H4K16ac is required for the formation of both active and silent chromatin. The process of creating stable silent and active states is not an one-step event, but requires positive feedback loops. H4K16ac may be the starting point for silent domains, which are reinforced by the Sir2 deacetylation reaction and possibly the generation of O-AADPR, and active domains, where it promotes H3K79 methylation. These interdependent pathways are conserved throughout evolution and mathematical modelling clearly shows that such networks are required to establish a stable binary switch (Dodd et al, 2007; Mukhopadhyay et al, 2010). Here, we have demonstrated that H4K16ac is actively implicated in the establishment of yeast silent chromatin, being the first histone mark shown to recruit Sir proteins to chromatin.
Materials and methods
SIR purification and chromatin reconstitution
In vitro reconstitution of SIR-bound chromatin was carried out essentially as described (Cubizolles et al, 2006; Martino et al, 2009). Briefly, the Sir proteins were expressed in sf21 insect cells with baculoviruses generated using BD BaculoGold™, BD-Biosciences. Co-infection was used to produce the Sir2-3-4 complex, the catalytic dead Sir2cd-3-4 and the Sir2-4 heterodimer, a single infection was used to produce the Sir3 protein alone (Cubizolles et al, 2006). Recombinant X. laevis histones were use to reconstitute histone octamers as described previously (Luger et al, 1997). Chromatin was assembled in vitro by adding increasing amounts of purified histone octamer to a constant amount of DNA arrays containing six 601-Widom positioning elements separated by 20 bp of linker DNA, referred as 601-167-6mer (Lowary and Widom, 1998). An unspecific DNA sequence of 147 bp (referred as ‘competitor’ on the figures) was added to the mix in order to bind the excess of histone octamers subsequent to the saturation of the 601-167-6mer (Huynh et al, 2005). Cy5-labelled 601-167-6mer was generated by filling the 5′ overhang-ends of an EcoRI site with Klenow enzyme (NEB, accordingly to manufacturer's instruction), using d-CTP-Cy5 (GE Healthcare). The free nucleotides were then separated from the Cy5-labelled array using small Bio-spin columns (Bio-Rad). DNA and histones were mixed in 40 μl of buffer A (10 mM TEA pH 7.4 and 1 mM EDTA) and 2 M NaCl on ice, and chromatin was reconstituted by step dialysis in buffer A containing 1.2, 1, 0.8 or 0.6 M NaCl for 2 h at 4°C and in buffer A overnight (Lee and Narlikar, 2001). The 601-167-6mer was routinely prepared at a final nucleosomal concentration of 10−6 M. Increasing amounts of Sir proteins were added to the 601-167-6mer diluted to 5 × 10−8 M or 2.5 × 10−8 M in 10 mM TEA pH 8, 25 mM NaCl, 0.05% Tween-20 on ice and after 10 min incubation the samples were fixed with 0.0025% glutaraldehyde for 10 min on ice. The fixation yields slightly sharper bands but the results are very similar without fixation. When chromatin deacetylation was coupled to Sir protein loading, SIR-bound chromatin was incubated with or without 150 μM NAD for 15 min at 30°C before incubation on ice for a 10 min fixation as above. The samples were routinely run at 80 V for 90 min at 4°C in a 0.7% agarose gel 0.2 × TB: 18 mM Tris, 18 mM Boric acid. The gel was soaked for 20 min in 1 × SYBR® Safe and the DNA was visualized in a Typhoon 9400 scanner.
Preparation of the histone modifications
Methylation of H3K79 was carried out on reconstituted chromatin as described before (Martino et al, 2009). Briefly, 0.8 pmol of recombinant Dot1 was incubated with 8 pmol of reconstituted 601-167-6mer in 25 mM Tris pH 7.9, 20 mM NaCl, 0.4 mM EDTA, with or without 160 pmol of S-adenosylmethionine (SAM) at 30°C for 30 min, then 160 pmol of SAM was added and the reaction was continued for 30 min. Mass spectrometry analysis showed that H3K79 is mono-, di- and, to a lesser extent, tri-methylated on at least 50% of the available K79 residues (Frederiks et al, 2008; Martino et al, 2009). The chromatin was then stored at 4°C.
Homogeneous acetylated histone H3 at the lysine 56 was obtained using an aminoacyl-tRNA synthetase and tRNACUA pair created by directed evolution in E. coli (Neumann et al, 2008). An unmodified control was prepared in parallel. Histone octamers were assembled as described previously (Luger et al, 1997) and kept at 4°C before chromatin reconstitution.
Full acetylation of H4K16 was obtained by NCL as described previously (Shogren-Knaak et al, 2006). Briefly, the H4 N-terminal peptide containing residues 1–22 and acetylated lysine at position 16 was synthesized using Fmoc (N-(9-fluorenyl)methoxycarbonyl)-based solid-phase synthesis and activated at the C-terminus by thioesterification. Subsequently, the globular X. laevis H4Δ1-22,R23C was ligated to the activated H4 peptide and the ligation product was purified as described previously (Shogren-Knaak et al, 2006). Identity and purity of the histones were verified by SDS–PAGE as well as ESI-MS (Supplementary Figure S1). Histone octamers were assembled as described previously (Luger et al, 1997) and kept at 4°C before chromatin reconstitution.
MNase digestion assay
MNase digestion was carried out in 20 μl of 10 mM TEA pH 8, 1.5 mM CaCl2, 25 mM NaCl, 0.05% Tween-20. In all, 1 pmol of 601-167-6mer was digested with increasing amounts of MNase, as detailed in the figures, for 12 min on ice. The digestion was stopped by adding 10 mM EGTA, proteins were removed by proteinase K digestion for 15 min at 30°C and the samples were run at 65 V for 60 min in a 1.2% agarose gel 1 × TBE: 90 mM Tris, 90 mM Boric acid, 2 mM EDTA. Digestion of SIR-bound chromatin was performed on 601-167-6mer pre-incubated with the indicated amount of Sir proteins for 10 min on ice. MNase digestion of deacetylated chromatin was performed on 601-167-6mer incubated with 0.66 pmol of Sir2-3-4, Sir2cd-3-4 or Sir2-4 for 15 min at 30°C with or without 150 μM NAD and recovered on ice. Concerning the Sir2-3-4 complex, similar results were obtained by incubating the nucleosomal array with 0.66 pmol of Sir2-4 at first and adding 0.66 pmol of Sir3 before the recovery on ice. In order to strengthen our observations, different batches of modified and unmodified chromatins were compared.
Deacetylation reaction
Deacetylation of 2 pmol of reconstituted chromatin was performed in 30 μl of 25 mM Tris pH 8, 50 mM NaCl in presence of 5 pmol of the Sir2-3-4 complex, the Sir2cd-3-4 mutant or Sir2-4 and 150 μM NAD for 30 min at 30°C and stopped by addition of 4 × Laemmli buffer. Similar results were obtained in 25 mM Tris pH 8, 137 mM NaCl, 2.7 mM KCl and 1 mM MgCl2. The acetylation state was determined by immunoblotting using acetylation mark-specific antibodies (anti-H3K56ac Upstate #07-677, anti-H4K16ac Serotec AHP417) and H3 for loading (anti-H3 Abcam ab1791-100).
Supplementary Material
Acknowledgments
We would like to thank the Gasser laboratory and in particular Helder Ferreira for discussion and support as well as Simon Lattman for assistance in analysing the data. We thank Heinz Neumann for providing a preliminary batch of H3K56ac histone octamers. The Gasser laboratory is supported by the Novartis Research Foundation and the EU network Nucleosome 4D. SK was supported by an EMBO long-term fellowship and an FWF Schroedinger fellowship.
Author contributions: MO, SK and SMG designed the experiments and interpreted results. MO performed the experiments. SK and FM contributed reagents. SS and WF contributed the H4K16ac histone octamers. SMH and JC contributed the H3K56ac histone octamers. MO, SK and SMG wrote the manuscript. SMG supervised the work.
References
- Altaf M, Utley RT, Lacoste N, Tan S, Briggs SD, Cote J (2007) Interplay of chromatin modifiers on a short basic patch of histone H4 tail defines the boundary of telomeric heterochromatin. Mol Cell 28: 1002–1014 [Europe PMC free article] [Abstract] [Google Scholar]
- Aparicio OM, Billington BL, Gottschling DE (1991) Modifiers of position effect are shared between telomeric and silent mating-type loci in S. cerevisiae. Cell 66: 1279–1287 [Abstract] [Google Scholar]
- Armstrong CM, Kaeberlein M, Imai SI, Guarente L (2002) Mutations in Saccharomyces cerevisiae gene SIR2 can have differential effects on in vivo silencing phenotypes and in vitro histone deacetylation activity. Mol Biol Cell 13: 1427–1438 [Europe PMC free article] [Abstract] [Google Scholar]
- Borra MT, Langer MR, Slama JT, Denu JM (2004) Substrate specificity and kinetic mechanism of the Sir2 family of NAD+-dependent histone/protein deacetylases. Biochemistry 43: 9877–9887 [Abstract] [Google Scholar]
- Buchberger JR, Onishi M, Li G, Seebacher J, Rudner AD, Gygi SP, Moazed D (2008) Sir3-nucleosome interactions in spreading of silent chromatin in Saccharomyces cerevisiae. Mol Cell Biol 28: 6903–6918 [Europe PMC free article] [Abstract] [Google Scholar]
- Carmen AA, Milne L, Grunstein M (2002) Acetylation of the yeast histone H4 N terminus regulates its binding to heterochromatin protein SIR3. J Biol Chem 277: 4778–4781 [Abstract] [Google Scholar]
- Celic I, Masumoto H, Griffith WP, Meluh P, Cotter RJ, Boeke JD, Verreault A (2006) The sirtuins hst3 and Hst4p preserve genome integrity by controlling histone h3 lysine 56 deacetylation. Curr Biol 16: 1280–1289 [Abstract] [Google Scholar]
- Chang CS, Pillus L (2009) Collaboration between the essential Esa1 acetyltransferase and the Rpd3 deacetylase is mediated by H4K12 histone acetylation in Saccharomyces cerevisiae. Genetics 183: 149–160 [Europe PMC free article] [Abstract] [Google Scholar]
- Chen L, Widom J (2005) Mechanism of transcriptional silencing in yeast. Cell 120: 37–48 [Abstract] [Google Scholar]
- Chou CC, Li YC, Gartenberg MR (2008) Bypassing Sir2 and O-acetyl-ADP-ribose in transcriptional silencing. Mol Cell 31: 650–659 [Europe PMC free article] [Abstract] [Google Scholar]
- Cubizolles F, Martino F, Perrod S, Gasser SM (2006) A homotrimer-heterotrimer switch in Sir2 structure differentiates rDNA and telomeric silencing. Mol Cell 21: 825–836 [Abstract] [Google Scholar]
- Davey CA, Sargent DF, Luger K, Maeder AW, Richmond TJ (2002) Solvent mediated interactions in the structure of the nucleosome core particle at 1.9 a resolution. J Mol Biol 319: 1097–1113 [Abstract] [Google Scholar]
- Dodd IB, Micheelsen MA, Sneppen K, Thon G (2007) Theoretical analysis of epigenetic cell memory by nucleosome modification. Cell 129: 813–822 [Abstract] [Google Scholar]
- Ehrenhofer-Murray AE, Rivier DH, Rine J (1997) The role of Sas2, an acetyltransferase homologue of Saccharomyces cerevisiae, in silencing and ORC function. Genetics 145: 923–934 [Europe PMC free article] [Abstract] [Google Scholar]
- Frederiks F, Tzouros M, Oudgenoeg G, van Welsem T, Fornerod M, Krijgsveld J, van Leeuwen F (2008) Nonprocessive methylation by Dot1 leads to functional redundancy of histone H3K79 methylation states. Nat Struct Mol Biol 15: 550–557 [Abstract] [Google Scholar]
- Gao L, Gross DS (2008) Sir2 silences gene transcription by targeting the transition between RNA polymerase II initiation and elongation. Mol Cell Biol 28: 3979–3994 [Europe PMC free article] [Abstract] [Google Scholar]
- Gasser SM, Cockell MM (2001) The molecular biology of the SIR proteins. Gene 279: 1–16 [Abstract] [Google Scholar]
- Ghidelli S, Donze D, Dhillon N, Kamakaka RT (2001) Sir2p exists in two nucleosome-binding complexes with distinct deacetylase activities. EMBO J 20: 4522–4535 [Europe PMC free article] [Abstract] [Google Scholar]
- Gottschling DE (1992) Telomere-proximal DNA in Saccharomyces cerevisiae is refractory to methyltransferase activity in vivo. Proc Natl Acad Sci USA 89: 4062–4065 [Europe PMC free article] [Abstract] [Google Scholar]
- Han J, Zhou H, Horazdovsky B, Zhang K, Xu RM, Zhang Z (2007) Rtt109 acetylates histone H3 lysine 56 and functions in DNA replication. Science 315: 653–655 [Abstract] [Google Scholar]
- Hecht A, Laroche T, Strahl-Bolsinger S, Gasser SM, Grunstein M (1995) Histone H3 and H4 N-termini interact with SIR3 and SIR4 proteins: a molecular model for the formation of heterochromatin in yeast. Cell 80: 583–592 [Abstract] [Google Scholar]
- Hickman MA, Rusche LN (2010) Transcriptional silencing functions of the yeast protein Orc1/Sir3 subfunctionalized after gene duplication. Proc Natl Acad Sci USA 107: 19384–19389 [Europe PMC free article] [Abstract] [Google Scholar]
- Hoppe GJ, Tanny JC, Rudner AD, Gerber SA, Danaie S, Gygi SP, Moazed D (2002) Steps in assembly of silent chromatin in yeast: Sir3-independent binding of a Sir2/Sir4 complex to silencers and role for Sir2-dependent deacetylation. Mol Cell Biol 22: 4167–4180 [Europe PMC free article] [Abstract] [Google Scholar]
- Huynh VA, Robinson PJ, Rhodes D (2005) A method for the in vitro reconstitution of a defined ‘30 nm’ chromatin fibre containing stoichiometric amounts of the linker histone. J Mol Biol 345: 957–968 [Abstract] [Google Scholar]
- Hyland EM, Cosgrove MS, Molina H, Wang D, Pandey A, Cottee RJ, Boeke JD (2005) Insights into the role of histone H3 and histone H4 core modifiable residues in Saccharomyces cerevisiae. Mol Cell Biol 25: 10060–10070 [Europe PMC free article] [Abstract] [Google Scholar]
- Imai S, Armstrong CM, Kaeberlein M, Guarente L (2000) Transcriptional silencing and longevity protein Sir2 is an NAD-dependent histone deacetylase. Nature 403: 795–800 [Abstract] [Google Scholar]
- Johnson A, Li G, Sikorski TW, Buratowski S, Woodcock CL, Moazed D (2009) Reconstitution of heterochromatin-dependent transcriptional gene silencing. Mol Cell 35: 769–781 [Europe PMC free article] [Abstract] [Google Scholar]
- Johnson LM, Fisher-Adams G, Grunstein M (1992) Identification of a non-basic domain in the histone H4 N-terminus required for repression of the yeast silent mating loci. EMBO J 11: 2201–2209 [Europe PMC free article] [Abstract] [Google Scholar]
- Johnson LM, Kayne PS, Kahn ES, Grunstein M (1990) Genetic evidence for an interaction between SIR3 and histone H4 in the repression of the silent mating loci in Saccharomyces cerevisiae. Proc Natl Acad Sci USA 87: 6286–6290 [Europe PMC free article] [Abstract] [Google Scholar]
- Katan-Khaykovich Y, Struhl K (2005) Heterochromatin formation involves changes in histone modifications over multiple cell generations. EMBO J 24: 2138–2149 [Europe PMC free article] [Abstract] [Google Scholar]
- Kimura A, Umehara T, Horikoshi M (2002) Chromosomal gradient of histone acetylation established by Sas2p and Sir2p functions as a shield against gene silencing. Nat Genet 32: 370–377 [Abstract] [Google Scholar]
- Lee KM, Narlikar G (2001) Assembly of nucleosomal templates by salt dialysis. Curr Protoc Mol Biol Chapter 21: Unit 21.6 [Abstract] [Google Scholar]
- Li Q, Zhou H, Wurtele H, Davies B, Horazdovsky B, Verreault A, Zhang Z (2008) Acetylation of histone H3 lysine 56 regulates replication-coupled nucleosome assembly. Cell 134: 244–255 [Europe PMC free article] [Abstract] [Google Scholar]
- Liou GG, Tanny JC, Kruger RG, Walz T, Moazed D (2005) Assembly of the SIR complex and its regulation by O-acetyl-ADP-ribose, a product of NAD-dependent histone deacetylation. Cell 121: 515–527 [Abstract] [Google Scholar]
- Loo S, Rine J (1994) Silencers and domains of generalized repression. Science 264: 1768–1771 [Abstract] [Google Scholar]
- Lowary PT, Widom J (1998) New DNA sequence rules for high affinity binding to histone octamer and sequence-directed nucleosome positioning. J Mol Biol 276: 19–42 [Abstract] [Google Scholar]
- Lu X, Simon MD, Chodaparambil JV, Hansen JC, Shokat KM, Luger K (2008) The effect of H3K79 dimethylation and H4K20 trimethylation on nucleosome and chromatin structure. Nat Struct Mol Biol 15: 1122–1124 [Europe PMC free article] [Abstract] [Google Scholar]
- Luger K, Mader AW, Richmond RK, Sargent DF, Richmond TJ (1997) Crystal structure of the nucleosome core particle at 2.8 A resolution. Nature 389: 251–260 [Abstract] [Google Scholar]
- Lynch PJ, Rusche LN (2009) A silencer promotes the assembly of silenced chromatin independently of recruitment. Mol Cell Biol 29: 43–56 [Europe PMC free article] [Abstract] [Google Scholar]
- Maas NL, Miller KM, DeFazio LG, Toczyski DP (2006) Cell cycle and checkpoint regulation of histone H3 K56 acetylation by Hst3 and Hst4. Mol Cell 23: 109–119 [Abstract] [Google Scholar]
- Martino F, Kueng S, Robinson P, Tsai-Pflugfelder M, van Leeuwen F, Ziegler M, Cubizolles F, Cockell MM, Rhodes D, Gasser SM (2009) Reconstitution of yeast silent chromatin: multiple contact sites and O-AADPR binding load SIR complexes onto nucleosomes in vitro. Mol Cell 33: 323–334 [Abstract] [Google Scholar]
- Masumoto H, Hawke D, Kobayashi R, Verreault A (2005) A role for cell-cycle-regulated histone H3 lysine 56 acetylation in the DNA damage response. Nature 436: 294–298 [Abstract] [Google Scholar]
- Megee PC, Morgan BA, Mittman BA, Smith MM (1990) Genetic analysis of histone H4: essential role of lysines subject to reversible acetylation. Science 247: 841–845 [Abstract] [Google Scholar]
- Meijsing SH, Ehrenhofer-Murray AE (2001) The silencing complex SAS-I links histone acetylation to the assembly of repressed chromatin by CAF-I and Asf1 in Saccharomyces cerevisiae. Genes Dev 15: 3169–3182 [Europe PMC free article] [Abstract] [Google Scholar]
- Michishita E, McCord RA, Boxer LD, Barber MF, Hong T, Gozani O, Chua KF (2009) Cell cycle-dependent deacetylation of telomeric histone H3 lysine K56 by human SIRT6. Cell Cycle 8: 2664–2666 [Europe PMC free article] [Abstract] [Google Scholar]
- Millar CB, Kurdistani SK, Grunstein M (2004) Acetylation of yeast histone H4 lysine 16: a switch for protein interactions in heterochromatin and euchromatin. Cold Spring Harb Symp Quant Biol 69: 193–200 [Abstract] [Google Scholar]
- Moazed D, Kistler A, Axelrod A, Rine J, Johnson AD (1997) Silent information regulator protein complexes in Saccharomyces cerevisiae: a SIR2/SIR4 complex and evidence for a regulatory domain in SIR4 that inhibits its interaction with SIR3. Proc Natl Acad Sci USA 94: 2186–2191 [Europe PMC free article] [Abstract] [Google Scholar]
- Mukhopadhyay S, Nagaraj VH, Sengupta AM (2010) Locus dependence in epigenetic chromatin silencing. Biosystems 102: 49–54 [Europe PMC free article] [Abstract] [Google Scholar]
- Neumann H, Hancock SM, Buning R, Routh A, Chapman L, Somers J, Owen-Hughes T, van Noort J, Rhodes D, Chin JW (2009) A method for genetically installing site-specific acetylation in recombinant histones defines the effects of H3 K56 acetylation. Mol Cell 36: 153–163 [Europe PMC free article] [Abstract] [Google Scholar]
- Neumann H, Peak-Chew SY, Chin JW (2008) Genetically encoding N(epsilon)-acetyllysine in recombinant proteins. Nat Chem Biol 4: 232–234 [Abstract] [Google Scholar]
- Ng HH, Ciccone DN, Morshead KB, Oettinger MA, Struhl K (2003) Lysine-79 of histone H3 is hypomethylated at silenced loci in yeast and mammalian cells: a potential mechanism for position-effect variegation. Proc Natl Acad Sci USA 100: 1820–1825 [Europe PMC free article] [Abstract] [Google Scholar]
- Ng HH, Feng Q, Wang H, Erdjument-Bromage H, Tempst P, Zhang Y, Struhl K (2002) Lysine methylation within the globular domain of histone H3 by Dot1 is important for telomeric silencing and Sir protein association. Genes Dev 16: 1518–1527 [Europe PMC free article] [Abstract] [Google Scholar]
- Norris A, Bianchet MA, Boeke JD (2008) Compensatory interactions between Sir3p and the nucleosomal LRS surface imply their direct interaction. PLoS Genet 4: e1000301. [Europe PMC free article] [Abstract] [Google Scholar]
- Onishi M, Liou GG, Buchberger JR, Walz T, Moazed D (2007) Role of the conserved Sir3-BAH domain in nucleosome binding and silent chromatin assembly. Mol Cell 28: 1015–1028 [Abstract] [Google Scholar]
- Osborne EA, Dudoit S, Rine J (2009) The establishment of gene silencing at single-cell resolution. Nat Genet 41: 800–806 [Europe PMC free article] [Abstract] [Google Scholar]
- Park EC, Szostak JW (1990) Point mutations in the yeast histone H4 gene prevent silencing of the silent mating type locus HML. Mol Cell Biol 10: 4932–4934 [Europe PMC free article] [Abstract] [Google Scholar]
- Pillus L, Rine J (1989) Pigenetic inheritance of transcriptional states in S. cerevisiae. Cell 59: 637–647 [Abstract] [Google Scholar]
- Reifsnyder C, Lowell J, Clarke A, Pillus L (1996) Yeast SAS silencing genes and human genes associated with AML and HIV-1 Tat interactions are homologous with acetyltransferases. Nat Genet 14: 42–49 [Abstract] [Google Scholar]
- Rine J, Herskowitz I (1987) Four genes responsible for a position effect on expression from HML and HMR in Saccharomyces cerevisiae. Genetics 116: 9–22 [Europe PMC free article] [Abstract] [Google Scholar]
- Robinson PJ, An W, Routh A, Martino F, Chapman L, Roeder RG, Rhodes D (2008) 30 nm chromatin fibre decompaction requires both H4-K16 acetylation and linker histone eviction. J Mol Biol 381: 816–825 [Europe PMC free article] [Abstract] [Google Scholar]
- Rudner AD, Hall BE, Ellenberger T, Moazed D (2005) A nonhistone protein-protein interaction required for assembly of the SIR complex and silent chromatin. Mol Cell Biol 25: 4514–4528 [Europe PMC free article] [Abstract] [Google Scholar]
- Rusche LN, Kirchmaier AL, Rine J (2003) The establishment, inheritance, and function of silenced chromatin in Saccharomyces cerevisiae. Annu Rev Biochem 72: 481–516 [Abstract] [Google Scholar]
- Sampath V, Yuan P, Wang IX, Prugar E, van Leeuwen F, Sternglanz R (2009) Mutational analysis of the Sir3 BAH domain reveals multiple points of interaction with nucleosomes. Mol Cell Biol 29: 2532–2545 [Europe PMC free article] [Abstract] [Google Scholar]
- Schneider J, Bajwa P, Johnson FC, Bhaumik SR, Shilatifard A (2006) Rtt109 is required for proper H3K56 acetylation: a chromatin mark associated with the elongating RNA polymerase II. J Biol Chem 281: 37270–37274 [Abstract] [Google Scholar]
- Schwaiger M, Kohler H, Oakeley EJ, Stadler MB, Schubeler D (2010) Heterochromatin protein 1 (HP1) modulates replication timing of the Drosophila genome. Genome Res 20: 771–780 [Europe PMC free article] [Abstract] [Google Scholar]
- Sekinger EA, Gross DS (2001) Silenced chromatin is permissive to activator binding and PIC recruitment. Cell 105: 403–414 [Abstract] [Google Scholar]
- Shogren-Knaak M, Ishii H, Sun JM, Pazin MJ, Davie JR, Peterson CL (2006) Histone H4-K16 acetylation controls chromatin structure and protein interactions. Science 311: 844–847 [Abstract] [Google Scholar]
- Smith CM, Gafken PR, Zhang Z, Gottschling DE, Smith JB, Smith DL (2003) Mass spectrometric quantification of acetylation at specific lysines within the amino-terminal tail of histone H4. Anal Biochem 316: 23–33 [Abstract] [Google Scholar]
- Smith JS, Brachmann CB, Celic I, Kenna MA, Muhammad S, Starai VJ, Avalos JL, Escalante-Semerena JC, Grubmeyer C, Wolberger C, Boeke JD (2000) A phylogenetically conserved NAD+-dependent protein deacetylase activity in the Sir2 protein family. Proc Natl Acad Sci USA 97: 6658–6663 [Europe PMC free article] [Abstract] [Google Scholar]
- Strahl-Bolsinger S, Hecht A, Luo K, Grunstein M (1997) SIR2 and SIR4 interactions differ in core and extended telomeric heterochromatin in yeast. Genes Dev 11: 83–93 [Abstract] [Google Scholar]
- Suka N, Luo K, Grunstein M (2002) Sir2p and Sas2p opposingly regulate acetylation of yeast histone H4 lysine16 and spreading of heterochromatin. Nat Genet 32: 378–383 [Abstract] [Google Scholar]
- Suka N, Suka Y, Carmen AA, Wu J, Grunstein M (2001) Highly specific antibodies determine histone acetylation site usage in yeast heterochromatin and euchromatin. Mol Cell 8: 473–479 [Abstract] [Google Scholar]
- Tanny JC, Dowd GJ, Huang J, Hilz H, Moazed D (1999) An enzymatic activity in the yeast Sir2 protein that is essential for gene silencing. Cell 99: 735–745 [Abstract] [Google Scholar]
- van Leeuwen F, Gafken PR, Gottschling DE (2002) Dot1p modulates silencing in yeast by methylation of the nucleosome core. Cell 109: 745–756 [Abstract] [Google Scholar]
- Varv S, Kristjuhan K, Peil K, Looke M, Mahlakoiv T, Paapsi K, Kristjuhan A (2010) Acetylation of H3 K56 is required for RNA polymerase II transcript elongation through heterochromatin in yeast. Mol Cell Biol 30: 1467–1477 [Europe PMC free article] [Abstract] [Google Scholar]
- Verzijlbergen KF, Faber AW, Stulemeijer IJ, van Leeuwen F (2009) Multiple histone modifications in euchromatin promote heterochromatin formation by redundant mechanisms in Saccharomyces cerevisiae. BMC Mol Biol 10: 76. [Europe PMC free article] [Abstract] [Google Scholar]
- Williams SK, Truong D, Tyler JK (2008) Acetylation in the globular core of histone H3 on lysine-56 promotes chromatin disassembly during transcriptional activation. Proc Natl Acad Sci USA 105: 9000–9005 [Europe PMC free article] [Abstract] [Google Scholar]
- Xie W, Song C, Young NL, Sperling AS, Xu F, Sridharan R, Conway AE, Garcia BA, Plath K, Clark AT, Grunstein M (2009) Histone h3 lysine 56 acetylation is linked to the core transcriptional network in human embryonic stem cells. Mol Cell 33: 417–427 [Europe PMC free article] [Abstract] [Google Scholar]
- Xu F, Zhang K, Grunstein M (2005) Acetylation in histone H3 globular domain regulates gene expression in yeast. Cell 121: 375–385 [Abstract] [Google Scholar]
- Xu F, Zhang Q, Zhang K, Xie W, Grunstein M (2007) Sir2 deacetylates histone H3 lysine 56 to regulate telomeric heterochromatin structure in yeast. Mol Cell 27: 890–900 [Europe PMC free article] [Abstract] [Google Scholar]
- Yang B, Britton J, Kirchmaier AL (2008a) Insights into the impact of histone acetylation and methylation on Sir protein recruitment, spreading, and silencing in Saccharomyces cerevisiae. J Mol Biol 381: 826–844 [Abstract] [Google Scholar]
- Yang B, Kirchmaier AL (2006) Bypassing the catalytic activity of SIR2 for SIR protein spreading in Saccharomyces cerevisiae. Mol Biol Cell 17: 5287–5297 [Europe PMC free article] [Abstract] [Google Scholar]
- Yang B, Miller A, Kirchmaier AL (2008b) HST3/HST4-dependent deacetylation of lysine 56 of histone H3 in silent chromatin. Mol Biol Cell 19: 4993–5005 [Europe PMC free article] [Abstract] [Google Scholar]
- Zou Y, Bi X (2008) Positive roles of SAS2 in DNA replication and transcriptional silencing in yeast. Nucleic Acids Res 36: 5189–5200 [Europe PMC free article] [Abstract] [Google Scholar]
Articles from The EMBO Journal are provided here courtesy of Nature Publishing Group
Full text links
Read article at publisher's site: https://doi.org/10.1038/emboj.2011.170
Read article for free, from open access legal sources, via Unpaywall:
https://onlinelibrary.wiley.com/doi/pdfdirect/10.1038/emboj.2011.170
Citations & impact
Impact metrics
Citations of article over time
Article citations
RNA Polymerase II Dependent Crosstalk between H4K16 Deacetylation and H3K56 Acetylation Promotes Transcription of Constitutively Expressed Genes.
Mol Cell Biol, 43(11):596-610, 17 Nov 2023
Cited by: 0 articles | PMID: 37937370 | PMCID: PMC10761024
Regulation of the heterochromatin spreading reaction by trans-acting factors.
Open Biol, 13(11):230271, 08 Nov 2023
Cited by: 2 articles | PMID: 37935357 | PMCID: PMC10645111
Review Free full text in Europe PMC
Sirtuins: Promising Therapeutic Targets to Treat Ischemic Stroke.
Biomolecules, 13(8):1210, 01 Aug 2023
Cited by: 4 articles | PMID: 37627275 | PMCID: PMC10452362
Review Free full text in Europe PMC
Inheritance of epigenetic transcriptional memory through read-write replication of a histone modification.
Ann N Y Acad Sci, 1526(1):50-58, 30 Jun 2023
Cited by: 6 articles | PMID: 37391188 | PMCID: PMC11216120
Review Free full text in Europe PMC
Sir2 and Reb1 antagonistically regulate nucleosome occupancy in subtelomeric X-elements and repress TERRAs by distinct mechanisms.
PLoS Genet, 18(9):e1010419, 22 Sep 2022
Cited by: 2 articles | PMID: 36137093 | PMCID: PMC9531808
Go to all (68) article citations
Data
Data behind the article
This data has been text mined from the article, or deposited into data resources.
BioStudies: supplemental material and supporting data
Similar Articles
To arrive at the top five similar articles we use a word-weighted algorithm to compare words from the Title and Abstract of each citation.
Reconstitution of heterochromatin-dependent transcriptional gene silencing.
Mol Cell, 35(6):769-781, 01 Sep 2009
Cited by: 65 articles | PMID: 19782027 | PMCID: PMC2842978
Reconstitution of yeast silent chromatin: multiple contact sites and O-AADPR binding load SIR complexes onto nucleosomes in vitro.
Mol Cell, 33(3):323-334, 01 Feb 2009
Cited by: 89 articles | PMID: 19217406
A nonhistone protein-protein interaction required for assembly of the SIR complex and silent chromatin.
Mol Cell Biol, 25(11):4514-4528, 01 Jun 2005
Cited by: 67 articles | PMID: 15899856 | PMCID: PMC1140625
SIR-nucleosome interactions: structure-function relationships in yeast silent chromatin.
Gene, 527(1):10-25, 18 Jun 2013
Cited by: 28 articles | PMID: 23791651
Review
Funding
Funders who supported this work.
Medical Research Council (2)
Systematic genetic code reprogramming
Professor Jason Chin, MRC Laboratory of Molecular Biology
Grant ID: MC_U105181009
Centre for Chemical and Synthetic Biology
Professor Jason Chin, MRC Laboratory of Molecular Biology
Grant ID: MC_UP_A024_1008