Abstract
Free full text

Deep sequencing of the human TCRγ and TCRβ repertoires provides evidence that TCRβ rearranges after αβ, γδ T-cell commitment
Abstract
The two main lineages of T lymphocytes develop from multi potent precursors in the human thymus. The most common type in blood are αβ T cells, which bind to antigenic peptides displayed on the surface of cells by human leukocyte antigen (HLA) molecules. Far less well understood are γδ T cells, which do not bind HLA: peptide complexes and are more prevalent in the gut mucosa. For both lineages, their ability to recognize a diverse array of antigens is mediated by a rearranged Y-like receptor on their surface, the T cell receptor (TCR), composed and of an α and β chain for αβ T cells or a γ and δ chain for γδ T cells. The canonical model for commitment from the precursor to one these two lineages assumes that γ, δ, and β chains rearrange prior to commitment to αβ or γδ T cells. A crucial step towards better understanding the role of γδ T cells is to work out the developmental process. To test the standard model and to understand the γδ TCR repertoire, we use high-throughput sequencing to catalog millions of TCRγ and TCRβ chains from peripheral blood αβ and γδ T cells, from three unrelated individuals. Almost all sampled αβ and γδ T cells have rearranged TCRγ sequences. While sampled αβ T cells have a diverse repertoire of rearranged TCRβ chains, less than 10% of γδ T cells in peripheral blood have a rearranged TCRβ chain. Our data indicate that TCRγ rearranges in all T lymphocytes, consistent with TCRγ rearranging prior to T cell lineage commitment, while rearrangement of the TCRβ locus is restricted, and occurs after T cell precursors commit to the αβ T cell lineage. This result explains the conundrum in T cell leukemia and lymphoma that TCRγ is almost always rearranged and TCRβ is only rearranged in a subset of cancers. As high-throughput sequencing of TCRs is translated into the clinic for monitoring minimal residual for leukemia/lymphoma, our data suggests the sequencing target needs to be TCR γ.
Introduction
The ability of T lymphocytes to mount an immune response against a diverse array of pathogens is primarily conveyed by the amino acid sequence of the hypervariable complementary determining region 3 (CDR3) regions of the T cell receptor (TCR). The genes that encode the two primary types of TCRs, αβ and γδ, undergo somatic rearrangement during T cell development. TCRβ and TCRδ genes are assembled via recombination of Variable (V), Diversity (D), and Joining (J) gene segments (VDJ recombination) and similarly, the TCRα and TCRγ genes by recombination of Variable and Joining gene segments (VJ recombination) to form productive αβ and γδ Y-like surface receptors.
The selection, function, and diversity of αβ T cells have been extensively studied. In the thymus (1), αβ T cells are both positively and negatively selected. Once selected, αβ T cells are activated when they recognize and bind non-self peptides that are in a protein complex with HLA and displayed on antigen presenting cells (APC). Due to the combinatorial diversity of αβ TCRs, the adaptive immune system has the potential to recognize an enormous number of antigens. Estimates based on direct sequencing of TCRβ chains indicate that at any one time, an individual carries over 3 million unique TCRβ CDR3 chains (2).
γδ TCRs were discovered four years after αβ TCRs (3, 4) and were predicted to have a different role in T-cell ontogeny based on significant differences in γδ TCR diversity, selection, and distribution (5). While significant discoveries have advanced the field, important basic questions about γδ T cell activation and function remain. Unlike αβ TCRs, γδ TCRs bind self antigens, leading many researchers to suggest that γδ T cells are not negatively selected in the thymus (6). Once the cells emigrate from the thymus, γδ TCRs can bind antigens independently of an HLA scaffold and APCs (7, 8). However, the role of the APC is still unclear; mounting evidence indicates APCs enhance the γδ T-cell response (9). The distribution of γδ T cells also differs substantially from αβ T cells: while αβ T cells are the predominant lymphocyte in the blood, γδ T cells are more common in mucosal tissue (10–12).
While only 5–10% of circulating T cells are γδ, in primates most of the circulating γδ T cells use the same Vγ and Vδ gene segments, Vγ9/Vδ2(13, 14). Following exposure to certain pathogens, including tuberculosis, leprosy, and malaria (13, 14), and tumor cells, including Daudi cells (15) T cells with Vγ9/Vδ2 chains expand rapidly. In some patients, this T-cell population (Vγ9/Vδ2) expands to over 50% of all circulating T cells (including αβ T cells) during bacterial infection (16). This immune response appears to be essential; the circulating γδ T-cell population in AIDS patients is depleted of Vγ9/Vδ2 type T cells, and the reduction of these T-cell types is associated with increased susceptibility to bacterial pathogens and lymphomas (17). Given that this γδ TCR chain type is the most common and responds to a variety of pathogens and tumors, circulating γδ T cells are often considered a bridge between the innate and adaptive immune system (16).
Both αβ and γδ T cells are derived from the same multi-potent precursor cells. These two types of T cells are defined by the expression of T-cell receptors (TCR) on their surface, which are either γδ or αβ heterodimers. The TCR variable regions do not rearrange simultaneously during T cell development; TCRδ rearranges first, followed by TCRγ and TCRβ. The TCRα locus rearranges last, after the surface expression of both pre-TCRα and TCRβ chains (18). The effect of TCR rearrangement and expression on γδ and αβ T-cell lineage commitment remains controversial (1, 19–23). The canonical model proposes that relative expression level of αβ and γδ surface receptors drives precursor T cells to differentiate into αβ or γδ T cells.
To study T cells in depth, we developed a method to sequence millions of TCRβ chains from genomic DNA in parallel from a single sample (24). This method was adapted to sequence TCRγ chains from genomic DNA and uses a multiplex set of PCR primers to simultaneously amplify each possible combination of V and J. In this study, we deeply sequence the TCRγ chains from three unrelated healthy people and elucidate the properties of the TCRγ repertoire. To explore the timing of T-cell differentiation into αβ and γδ T lineages, we sorted peripheral blood samples into αβ and γδ T cells and then sequenced TCRβ and TCRγ from both lineages. While the canonical model (22, 24) suggests that TCRβ rearrangement precedes lineage fate decisions, we find that γδ T cells have few (or possibly no) rearranged TCRβ genes, suggesting that cell fate decision are decided prior to TCRβ rearrangement. TCRγ genes are rearranged in most or all αβ T cells, consistent with the evidence that TCRγ rearranges before cell fate is determined.
Results
Utilizing a high-throughput sequencing methodology, we sequenced both TCRβ and TCRγ repertoires from sorted αβ and γδ T-cell subsets collected from three healthy people’s blood. The TCRγ and TCRβ CDR3 chains were sequenced from genomic DNA extracted from approximately 150,000 T cells for each sample. We over sample from the PCR pool and use redundancy to correct errors inherent to the PCR amplification and sequencing processes (25) (Table 1).
Table 1
Sequenced TCRγ CDR3 chains.
Total TCRγ CDR3 nucleotide sequences | Unique TCRγ CDR3 nucleotide sequences | |||||||
---|---|---|---|---|---|---|---|---|
| ||||||||
αβ cells | γδ cells | αβ cells | γδ cells | |||||
| ||||||||
Total reads | % in-frame | Total reads | % in-frame | Unique reads | % in-frame | Unique reads | % in-frame | |
Sample A | 330,0521 | 31.1 | 5,400,495 | 89.7 | 61,265 | 28.2 | 22,883 | 57.0 |
Sample B | 1,343,052 | 28.8 | 1,931,397 | 82.6 | 42,814 | 28.8 | 13,096 | 50.8 |
Sample C | 873,497 | 37.4 | 3,177,899 | 66.0 | 30,320 | 30.6 | 9,086 | 52.8 |
TCRγ sequences in γδ and αβ T cells
To assess the distribution of TCRγ CDR3 sequences in both γδ and αβ T cells, TCRγ chains were amplified from all samples using a multiplex PCR reaction and sequenced using the Illumina platform. A mean of 1.6 million TCRγ sequences were amplified from αβ T-cell samples and a mean of 3 million TCRγ sequences were amplified from γδ T-cell samples (Table 1). The overall diversity was dramatically different between γδ and αβ T cells: three-fold more unique TCRγ CDR3 sequences were amplified from αβ T cells than γδ T cells, with an average of 44799 and 15021, respectively (Table 1). The TCRγ repertoire of γδ T-cells was dominated by one clone, this most frequent TCRγ CDR3 sequence represented >45% of all amplified TCRγ sequences (Fig. 1A). In all three samples, this most abundant TCRγ CDR3 sequence (TGTGCCTTGTGGGAGGTGCAAGAGTTGGGCAAAA) used Vγ9 and JγP gene segments, and was nucleotide identical across the CDR3 region between individuals (Fig. 2). The majority of the remaining TCRγ sequences from γδ T cells also used the gene segments Vγ9 and JγP (Fig. 3A). The ten most common unique TCRγ sequences account for 80% of the total γδ T cells. The remaining sequences, which are too rare to have been observed with lower throughput technologies, encode a diverse set of sequences and differed substantially between individuals.
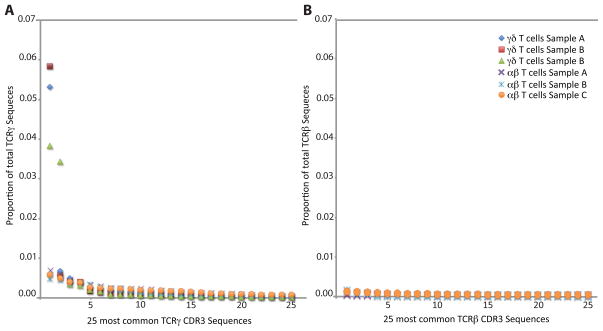
For each sample we plot the proportion of productive sequences accounted for by the 25 most numerous productive TCR sequences.(1A) TCRγ chains amplified from γδ T cells and αβ T cells and (1B) TCRβ chains amplified from αβ T cells.
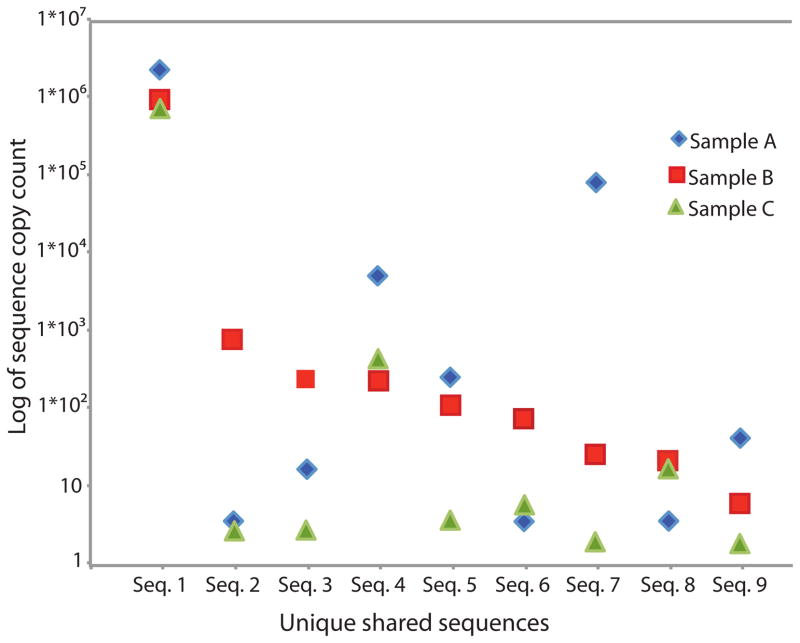
Nine nucleotide identical TCRγ CDR3 sequences amplified from γδ T cells are shared by all three individuals. For each shared sequence, the copy count detected for each individual is indicated on the Y-axis.
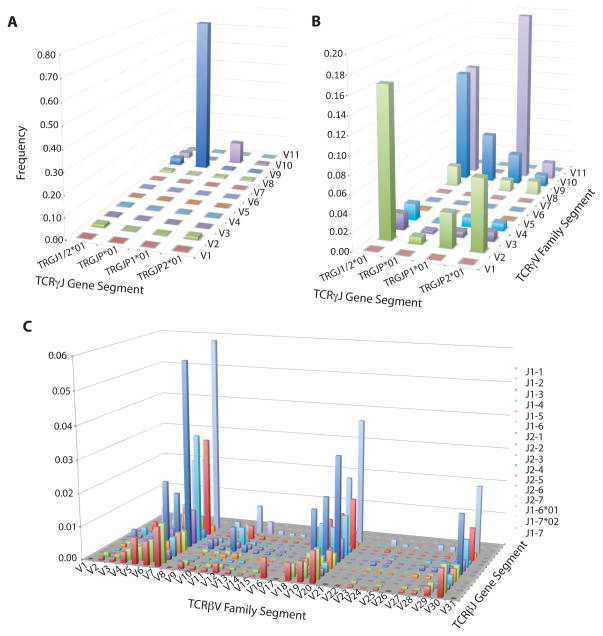
Average V-J utilization of gene segments in TCRγ CDR3 sequences amplified from γδ T cells (3A), TCRγ CDR3 sequences amplified from αβ T cells (3B), and TCRβ sequences amplified from αβ T cells (3C).
The TCRγ chains amplified from αβ T cells were more diverse than those from γδ cells. In the αβ T cells, no single TCRγ sequence represented more than 7% of the overall TCR population (Fig. 1B) and no single Vγ or Jγ gene segment dominated the repertoire (Fig. 3B). The majority of TCRγ sequences utilized only three of the nine Vγ segments, however the assay does capture all possible VJ combinations as detected by the VJ usage of unique out-of-frame TCRγ CDR3 sequences in αβ T cells (Fig. 4). Many TCRγ CDR3 sequences amplified from αβ T cells utilized Vγ10 gene segments, a gene segment predicted to have a non-consensus donor splice site in the first exon, resulting in the absence of splicing of the leader intron and termination of translation of the leader peptide (26, 27).
Previously observed TCRγ sequences
We identified that seven of the TCRγ CDR3 sequences amplified in this study have been previously sequenced and reported in at least seven publications. Over 80% of in-frame PBMC TCRγ amplified by this study use Vγ9JγP gene segments (Fig. 3A), consistent with previous observations (28, 29). Most of these are nucleotide identical and have been reported in five scientific publications to be part of γδ heterodimers that bind biologically important epitopes (15, 29–33). Six additional sequences that use Vγ9JγP gene segments sequences by this study are also reported in one publication to bind biologically important epitopes (33) and one Vγ8 chain shared by two individuals was identified as a public TCR that responds to CMV (34).
Productive vs. non-productive TCRγ rearrangements in γδ T cells and αβ T cells
As TCRs rearrange prior to thymic selection, random insertions and deletions in the CDR3 region would result in approximately one-third in-frame and two-thirds out-of-frame rearrangements. Thymic selection then skews this ratio, as a T cell must contain at least one productive TCR (which has to have both components of the heterodimer in-frame) to survive and leave the thymus. In our data, the observed fraction of unique in-frame TCRγ sequences is 53.5+/−2.6% in γδ T cells and 31 % in αβ T cells (Table 1). In αβ T cells, the observed fraction of unique in-frame TCRβ sequences is 82%, with 18% of TCRβ CDR3 sequences being out-of-frame (Table 2).
Table 2
Sequenced TCRβ CDR3 chains.
Total TCRβ CDR3 nucleotide reads | Unique TCRβ CDR3 nucleotide reads | |||||||
---|---|---|---|---|---|---|---|---|
| ||||||||
αβ cells | γδ cells | αβ cells | γδ cells | |||||
| ||||||||
Total reads | % in-frame | Total reads | % in-frame | Unique reads | % in-frame | Unique reads | % in-frame | |
Sample A | 316,915 | 80.5 | 10,134 | 25.0 | 37,482 | 81.3 | 705 | 36.7 |
Sample B | 233,507 | 83.0 | 12,306 | 53.9 | 30,874 | 82.9 | 1,239 | 69.5 |
Sample C | 201,833 | 80.6 | 21,975 | 30.0 | 27,292 | 82.4 | 628 | 58.6 |
TCRβ sequences in γδ T cells and αβ T cells
To assess the presence and diversity of TCRβ CDR3 chains in both γδ and αβ T cells, the TCRβ chains were amplified at high-throughput for all samples. Over 1.4 million TCRβ CDR3 chains were sequenced from approximately 250,000 haploid genomes. Within this population, there were 95,648 unique TCRβ CDR3 sequences (Table 2). The TCRβ chains amplified from αβ T cells represented a relatively diverse population, with diverse V (D) J usage (Fig 3C), and the most common TCRβ CDR3 sequence representing just 0.3% of the sequenced sample (Fig. 1B). Just 2,582 unique TCRβ CDR3 sequences were sequenced from a corresponding population of 250,000 haploid genomes extracted from γδ T cells. This number of TCRβ chains is within expectations for contamination of the γδ cell population with αβ T cells during cell sorting (<4%).
Discussion
High throughput sequencing technology allows analysis of the TCRγ and TCRβ repertoire at an unprecedented depth. Both circulating αβ and γδ T cells carried rearranged TCRγ chains, and the productive to non-productive ratios suggest that, at least in γδ T cells, both alleles rearrange prior to thymic selection. While the TCRγ repertoire of αβ T cells contains no major clone, the majority of γδ T cells sampled from peripheral blood utilize an identical germline TCRγ rearrangement of Vγ9JγP gene segments that lack insertions at the V-J junction. However, 20% of the circulating TCRγ repertoire of γδ T-cells is diverse. This underlying diverse repertoire was virtually invisible with older detection methods (29). The predominant Vγ9JγP sequence in all γδ T cell samples is identical to a TCRγ receptor with well-studied binding affinities (30). While a few of the sequences from the remainder of the γδ T-cell repertoire have known functions, most have not been previously studied or observed (33, 34). The majority of γδ T cells did not carry a rearranged TCRβ chain, consistent with TCRβ rearranging after T cell lineage bifurcation. The distribution of TCRγ sequences in blood suggests that γδ T cells share qualities with both the innate and adaptive immune system, with universal sequences shared across individuals likely to perform innate functions, while the background repertoire of diversity may play a more adaptive role.
Most models of T-cell lineage decision assume that TCRδ, TCRγ, and TCRβ rearrange prior to T-cell fate commitment (22, 35, 36). However, the majority (>90%) of γδ T cells included in this study lacked a rearranged TCRβ chain. These results are consistent with a previous study that provided evidence that TCRβ chains rearrange after both TCRδ and TCRγ (18), and that TCRβ are not rearranged in γδ T cells (37). These results indicate that TCRβ rearranges after T-cell lineage bifurcation, and that successful TCR rearrangement is an unlikely lineage commitment signal.
However our data indicates that both TCRγ alleles rearrange in the majority of T cells, in agreement with the prior published data (38). Other evidence also suggests that TCRγ rearranges prior to the cell fate determination and, therefore, prior to thymic selection (18, 36). Given that TCRs rearrange prior to thymic selection, random insertions and deletions in the CDR3 region would result in approximately one-third in-frame and two-thirds out-of-frame rearrangements. In γδ T cells thymic selection then would skew this ratio, as a T cell must contain at least one productive TCR to survive and leave the thymus. Our data is consistent with both TCRγ alleles rearranging prior to selection. Since we only observe T cells after thymic selection, all γδ T cells with both TCRγ alleles rearranged out-of-frame would die in the thymus. Therefore, under this model, we expect to observe T cells with one in-frame TCRγ rearrangement with the other allele either in-frame or out-of-frame. With an expectation of two thirds of TCRγ rearrangements being out-of-frame,
Strikingly, the most common TCRγ sequence does not have a corresponding in-frame or out-of-frame TCRγ sequence with similar copy count. This, and that the dominate clone lacks non-template insertions, suggests that the dominant clone developed under different circumstances than the underlying diverse array of γδ T cells. The lack of a corresponding sequence of similar copy count suggests either recombination was restricted to one allele or both alleles were restricted to the same rearrangement and the lack of insertions suggest that the terminal deoxynucleotidyl transferase (TdT) enzyme is not present during the rearrangement. Although indirect, this suggests that this dominant clone is formed very early in human development, likely a prenatal rearrangement prior to gestational week 20 when TdT is first expressed in the fetal thymus. This hypothesis has precedent; during mouse fetal development γδ T cells with determinate TCRs develop and home to specific tissues. While some work indicates that this TCRγ chain is not common in neonates (40), work by McVay and Carding found that this TCRγ chain was present in fetal livers at 11 weeks (41). Later work indicates that this population of γδ T cells develops extra-thymically in the fetal liver and primitive gut (42). This supports the hypothesis that the dominant clone is innate.
Several biologically important epitopes have been reported to bind γδ heterodimers with TCRγ chains amplified by this study. The most abundant TCRγ sequence chain in all three individuals is identical to a TCRγ CDR3 chain reported by Wang et al. to be part of a γδ heterodimer that reacts to a variety of nonpeptide prenyl phosphates including (E)-4-hydroxy-3-methyl-but-2-enyl pyrophosphate (HMBPP), and isopentenyl phosphate (IPP)(30). Non-peptide prenyl phosphates, metabolites common to all organisms, are associated with Vγ9/Vδ2 T-cell expansion (31). Some types are more reactive, like HMBPP, a metabolite of the 2-C-methyl-D-erythritol-4 phosphate pathway for isoprenoid biosynthesis that is more common in Eubacteria and some protozoa. This same TCRγ sequence is found in the TCRs of T cells that respond to Daudi Burkitt lymphoma cells (15, 32, 43) and Molt-4 tumor cells (29). In addition to the dominant sequence, multiple sequences amplified in this study were previously asserted by Chen et al. (2008) to bind epitopes displayed on the surface of SKOV3 tumor cells and HBV infected cells. These epitopes include human mutS homolog 2 (hMSH2) and heat shock protein (HSP) 60(33). Finally, a chain that uses Vγ8, CATWDTTG, that was present in two sampled individuals (Sample B and Sample C), is a public TCRγ chain that reacts with CMV and is expanded in infants infected with CMV (34). Given that the frequency of Vγ9/Vδ2 T-cells is positively correlated with natural repression of the HIV virus (44), and rapid expansion of this population is associated with rapid clearance of Tuberculosis in rhesus monkeys (13), the frequency of the major V9JP clone may be a useful biomarker.
For the analysis presented, we limited each sample to 250,000 haploid genomes due to the relatively small fraction of γδ T cells in human blood. However, the assay presented has the potential to sequence many millions of TCRγ chains. This depth of coverage readily allows detection of a TCR clone at one part in a 100,000 or even lower. All T cells appear to rearrange TCRγ, including αβ T cells, so this assay allows us to track clones with unprecedented sensitivity, and significant potential for clinical applications. Given the most common TCRγ clone has likely important biological functions, the clone’s frequency may be an important indicator of immune health and or immune response. In addition, given that TCRγ rearranges in both γδ and αβ T cells, TCRγ clones can be useful for tracking minimal residual disease in blood cancers. Given the technology we used in this paper, we can follow the clonal expansion and contraction for hundreds of thousands of T-cell clones over time.
Materials & Methods
Samples
We collected 40 ml of blood from three, one male and two female, healthy unrelated adults. PBMC was isolated from whole blood using a Ficoll histoplaque gradient. Following separation the total number of cells was estimated based on the number of cells counted in 1 mm3 of sample.
Sorting αβ and γδ T cells
For each individual, isolated PBMCs were separated into two fractions of approximately 20M cells (18 M–25 M). Cells were labeled with either anit-γδ TCR antibodies conjugated with hapten or anti-αβ TCR antibodies conjugated with phycoerythrin (PE). These labeled cells were treated with either anti-hapten antibodies or anti-PE antibodies coupled to microbeads (Miltenyi Biotech). Micro-bead labeled cells were positively selected using Miltenyi cell separator columns. To achieve maximum purity (>96%) for rare cells, anit-γδ TCR labeled cells were passed over two purification columns. The number of recovered cells was estimated from the number of cells counted in a 1mm3 subset. Between five and ten million αβ T cells and 200 thousand and one million γδ T cells were recovered for each sample. DNA was extracted from circulating γδ T cells, and circulating αβ T cells using Qiagen Maxi DNA isolation kits (QIAGEN Inc., Valencia, CA).
Sequencing CDR3 regions
TCRG and TCRB CDR3 regions were amplified and sequenced from 800 ng of extracted DNA. Amplification and sequencing of TCRβCDR3 regions was carried out as previously described by Robins et al. (25). To amplify the TCRγ template, we designed a muliplex PCR method to amplify all possible rearranged genomic TCRγCDR3 sequences. The method uses nine Forward primers specific to all Vγ gene segments and five reverse primers specific to all Jγ gene segments, including Vγ and Jγ gene segments that are predicted open-reading frames (IMGT). Amplification and sequencing protocols were modified for TCRγ from methods designed for amplifying TCRβ (25).
Identifying CDR3 sequences
Both the TCRβ and TCRγCDR3 region was delineated according to the definition established by the International ImMunoGeneTics collaboration (45). Sequences that did not match CDR3 sequences were removed from the analysis. A standard algorithm was used to identify which V, D, and J segments contributed to each TCRβCDR3 sequence and which V and J segments contributed to each TCRγCDR3 sequence (45). Rearranged CDR3 sequences were classified as non-productive if they included insertions or deletions that resulted in sequences with frame-shifts or premature stop-codons.
Identifying productive rearranged TCRγ and TCRβ in T cells
The number of productive and non-productive rearranged TCRβ and TCRγ in sampled circulating αβ and γδT cells were calculated for each individual. CDR3 sequences, in which translating a functional TCR is impossible, due to insertions or deletions that cause frame-shifts or stop codons, are considered non-productive rearrangements. Whereas CDR3 sequences from which a functional TCR is possible, are considered productive rearrangements.
Literature search for functional data on TCRγ
We searched PubMed for articles that matched the query “(T-cell receptor[Title/Abstract] OR TCR[Title/Abstract]) AND peptide[Title/Abstract]”. This resulted in 4899 records. We then used a Python script to download the full-text PDF of these articles which lead to at least one PDF file for 3520 articles. These files were converted to ASCII text with the tool pdf to text from the xpdf package (http://www.foolabs.com/xpdf). We ran Peptide::Peptide (46) at default settings over all text files in order to find the peptide sequences in them. Peptide sequences were searched with BLAT (47) against the CDR3 sequences and the results inspected manually.
References and Notes
Full text links
Read article at publisher's site: https://doi.org/10.1126/scitranslmed.3002536
Read article for free, from open access legal sources, via Unpaywall:
https://europepmc.org/articles/pmc4179204?pdf=render
Citations & impact
Impact metrics
Citations of article over time
Alternative metrics

Discover the attention surrounding your research
https://www.altmetric.com/details/101860082
Smart citations by scite.ai
Explore citation contexts and check if this article has been
supported or disputed.
https://scite.ai/reports/10.1126/scitranslmed.3002536
Article citations
Effector memory-type regulatory T cells display phenotypic and functional instability.
Sci Adv, 10(36):eadn3470, 04 Sep 2024
Cited by: 0 articles | PMID: 39231218 | PMCID: PMC11421655
Evolution of T cells in the cancer-resistant naked mole-rat.
Nat Commun, 15(1):3145, 11 Apr 2024
Cited by: 1 article | PMID: 38605005 | PMCID: PMC11009300
Flow Sorting, Whole Genome Amplification and Next-Generation Sequencing as Combined Tools to Study Heterogeneous Acute Lymphoblastic Leukemia.
Diagnostics (Basel), 13(21):3306, 25 Oct 2023
Cited by: 0 articles | PMID: 37958202 | PMCID: PMC10650172
Antigen-specificity measurements are the key to understanding T cell responses.
Front Immunol, 14:1127470, 14 Apr 2023
Cited by: 3 articles | PMID: 37122719 | PMCID: PMC10140422
Review Free full text in Europe PMC
The emerging roles of γδ T cells in cancer immunotherapy.
Nat Rev Clin Oncol, 20(3):178-191, 09 Jan 2023
Cited by: 88 articles | PMID: 36624304
Review
Go to all (78) article citations
Similar Articles
To arrive at the top five similar articles we use a word-weighted algorithm to compare words from the Title and Abstract of each citation.
Human alpha beta and gamma delta thymocyte development: TCR gene rearrangements, intracellular TCR beta expression, and gamma delta developmental potential--differences between men and mice.
J Immunol, 176(3):1543-1552, 01 Feb 2006
Cited by: 40 articles | PMID: 16424183 | PMCID: PMC1592528
On the role of the pre-T cell receptor in alphabeta versus gammadelta T lineage commitment.
Immunity, 9(5):649-655, 01 Nov 1998
Cited by: 75 articles | PMID: 9846486
Evidence for the divergence of innate and adaptive T-cell precursors before commitment to the αβ and γδ lineages.
Blood, 118(25):6591-6600, 21 Oct 2011
Cited by: 18 articles | PMID: 22021367
Development and selection of gammadelta T cells.
Immunol Rev, 215:15-31, 01 Feb 2007
Cited by: 118 articles | PMID: 17291276
Review
Funding
Funders who supported this work.
NIAID NIH HHS (2)
Grant ID: R56 AI081860
Grant ID: AI081860