Abstract
Free full text

Characterization of In Vitro Endothelial Linings Grown Within Microfluidic Channels
Abstract
In vivo, endothelial cells grow on the inner surface of blood vessels and are shaped to conform to the vessel's geometry. In the smallest vessels this shape entails substantial bending within each cell. Microfabricated channels can replicate these small-scale geometries, but endothelial cells grown within them have not been fully characterized. In particular, the presence of focal adhesions and adherens junctions in endothelial cells grown in microchannels with corners has not been confirmed. We have fabricated square microfluidic channels (50μm wide, 50
μm deep) and semicircular microfluidic channels (60
μm wide, 45
μm deep) in polydimethylsiloxane and cultured human umbilical vein endothelial cells (HUVEC) within them. Immunofluorescent staining and three-dimensional reconstruction of image stacks taken with confocal microscopy confirmed that HUVEC are capable of forming adherens junctions on all channel walls in both channel geometries, including the sidewalls of square profile channels. The presence of shear stress is critical for the cells to form focal adhesions within both channel geometries. Shear stress is also responsible for the conforming of HUVEC to the channel walls and produces a square cross-sectional geometry of in vitro endothelial linings within square profile channels. Thus, geometry and applied shear stress are important design criteria for the development of in vitro endothelial linings of microvessels.
Introduction
Experimenting with in vitro models of the endothelial lining of microvessels allows researchers to develop an understanding of disease phenomena such as atherosclerotic plaque formation, inflammation, and cancer metastasis. In the past, parallel plate flow chambers have been used to study cell interactions with the endothelium under physiological shear conditions.1 More recently developed device designs provide physiological shear stresses and also take the small diameter and the rounded geometry of in vivo microvessels into account. Besides replicating small vessel diameters and rounded wall geometries, microfabrication techniques also allow us to recreate branching points within in vitro vessel networks as they occur in vivo.2–4 These models that more faithfully replicate in vivo blood vessels are starting to replace parallel plate flow chambers for investigating disease processes in the microvasculature.5,6
Small diameter tubes that provide rectangular, trapezoidal (50μm wide and larger), and round (60–200
μm in diameter) channel wall geometries have been fabricated using various methods and substrates such as polydimethylsiloxane (PDMS), poly(glycerol sebacate), hydrogels, and collagen.6–10 Because of its transparency, low cost, and ease of handling, PDMS is one of the most commonly used materials. Square channels can be fabricated in PDMS relatively easily with standard microfabrication techniques such as SU-8 imaging. The fabrication of channels with round cross-sectional profiles is more complicated and requires the use of techniques such as resist reflow11 or isotropic etching and subsequent bonding of two half channels.
Even though several studies have shown that endothelial cells can be grown within microfluidic channels of developed devices,6,7,9,10,12 knowledge about the impact of geometry on endothelial cell structure and behavior within very small (<100μm) microfabricated channels is limited.13 Most importantly, the formation of intercellular bridges (adherens junctions) within microfluidic channels has not been confirmed. Adherens junctions are protein complexes that connect endothelial cells to each other and regulate molecular traffic through intercellular space. They are essential in establishing endothelial barrier function. Compromisation of endothelial barrier function often precedes or facilitates various disease processes, including inflammation and cancer metastasis.14 Thus, a key feature of in vitro models of microvessels or engineered vascular tissue is the formation of a confluent endothelial monolayer with developed adherens junctions. In addition, endothelial cell adhesion to the channel material is crucial for proper cell function.15 Focal adhesions physically link the intracellular cytoskeleton and the cell surface to the underlying substrate.16 Endothelial cells that lack focal adhesions undergo apoptosis.17–19 By linking to components of the cytoskeleton and signaling cascades, focal adhesions transmit mechanical signals from the extracellular environment, allowing cells to change shape and move in response to these signals.20–22
It has been found that both the geometry of the underlying substrate and the shear stress to which endothelial cells can be exposed affect the endothelial cytoskeleton. Endothelial cells that grow on curved vessel walls contain about half as many actin stress fibers as endothelial cells that grow on flat surfaces.23 Cells in curved vessels respond to shear stress by decreasing the number of stress fibers, whereas shear applied to cells grown on flat surfaces does not influence the number of stress fibers.23 When endothelial cells are exposed to mechanical forces or when cytoskeletal tension is high, the number of focal adhesions increases.24,25 Cells that are subjected to shear forces via a stream of medium respond with the formation of new focal adhesions in the direction of flow.26 They also stretch and re-arrange their cytoskeleton.27,28 Geometry and shear stress can also affect other cellular processes. Cells can alter their gene expression in response to shear stress.5 Protein expression of surface receptors, such as CD31, differs in endothelial cells grown in round expanded polytetrafluoroethylene cylinders and in flat parallel plate flow chambers.5 Thus, geometry and shear are factors that have to be taken into account when designing in vitro microvessels and experimenting with endothelial cells within microfluidic channels.
We hypothesized that fabricated microchannel geometry and exposure to shear stress both affect the ability of endothelial cells to form confluent, substrate-adherent monolayers when cultured within microfluidic channels. We tested this by constructing small (50–60μm) channels with semicircular and square cross-sectional profiles in PDMS, seeding human umbilical vein endothelial cells (HUVEC) in these channels and culturing them under static conditions and with applied shear. We evaluated confluency and substrate adhesion by immunofluorescent staining of vascular endothelial (VE)-cadherin and vinculin, markers of adherens junctions, and focal contacts, respectively.29,30 We found that shear had a larger impact than geometry on endothelial confluence and substrate adhesion within microfluidic channels.
Materials and Methods
Microfabrication
Channels with 50×50μm square cross-sectional profiles were prepared by casting PDMS (Sylgard 184; Dow Corning, Midland, MI) on SU-8 masters. To fabricate SU-8 masters, SU-8 2050 (MicroChem Corp., Newton, MA) was spun at 2800
rpm, baked for 1
min at 60°C and then 6
min at 90°C, exposed at 250
mJ/cm2 using a broadband ultraviolet light source (365–405
nm), baked postexposure for 1
min at 60°C and then 6
min at 90°C, and finally developed for 3
min (SU-8 developer; MicroChem Corp.). Channels with semicircular cross-sectional profiles (60
μm wide and 45
μm deep) were etched into silicon using an SF6-plasma generated with a Unaxis SLR 770 deep reactive ion etch tool (Plasma-Therm LLC, St. Petersburg, FL). The channels were etched for 8
min and the pattern was transferred to a plastic replica (Zeonor 1020R; Zionex, Inc., Tokyo, Japan) through pressurizing a silicon wafer/Zeonor stack at 120°C. The plastic replica then served as a mold for PDMS casting (Fig. 1). The square and semicircular PDMS channels were activated via oxygen plasma applied through a PlasmaFlo plasma cleaner at 29.6 W (Herrick Plasma, Ithaca, NY) (for 30
s at room temperature) and permanently bonded to 24×48
mm glass cover slips.
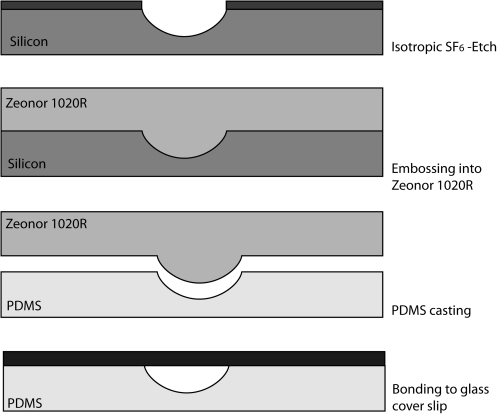
Fabrication of PDMS channels with rounded semicircular cross-sectional profiles: Channels with 60μm wide openings were etched with SF6 into silicon to a depth of 45
μm. The etched feature was transferred to hard plastic (Zeonor 1020R) via imprinting at 120°C. The plastic replica then served as a mold for PDMS casting. The PDMS channels was then activated with oxygen plasma and bonded to a 20×40
mm glass cover slip. The channels were coated with fibronectin before seeding HUVEC. PDMS, polydimethylsiloxane; HUVEC, human umbilical vein endothelial cells.
Seeding HUVEC into PDMS channels
HUVEC (Lonza Group Ltd., Basel, Switzerland) were maintained in culture in a humidified chamber at 37°C with 5% CO2 using specific growth medium (EGM with supplements, Lonza Group Ltd.). To ensure that the cells retained their primary endothelial characteristics, experiments were conducted before passage 11. Cells were detached using 0.0625% trypsin-EDTA (Sigma-Aldrich, St. Louis, MO). The cells were concentrated by centrifugation and cell counts were performed using a hemocytometer with Trypan-blue exclusion. Cell viability was >99% for all experiments. Before seeding, the PDMS was sterilized for 15min in 70% ethanol, air-dried, and then coated with fibronectin (10
μg/mL; Sigma-Aldrich) for 1
h at 37°C.
For culture under flow conditions, all PDMS channels were sealed with glass coverslips. HUVEC (5×105 per cm2) were seeded into inlets at the entrance to the closed channels with a micropipette, and then a flow rate of 0.5μL/min was subsequently applied for 30
s to draw the cells from the inlet into the channel. Flow was then stopped and the cells that were distributed along the channel length were allowed to attach for 1
h under static conditions. The PDMS channels were then immersed in fresh medium, which was pulled through the channel at flow rates of 0.5, 3, or 7.5
μL/min with a syringe pump. For culture under static conditions, HUVEC (2×105 per cm2) were added to open PDMS channels, allowed to attach to the surface for 1
h, and then the PDMS was covered with medium. Medium was replaced every 2–3 days for both static and flow systems and cell growth and viability were monitored with phase-contrast microscopy.
Estimating the shear stress in microfluidic channels
The mean wall shear stress within closed channels was estimated using the following equation.31
with μ being the dynamic viscosity of medium in Pa·s (measured to be 1 Pa·s for EGM-2 medium), is the ratio of the channel semi-axes c and b in meters (m), w is the mean fluid velocity in m/s, A is the cross-sectional area of the channel in m2, and P is the perimeter of the channel in m.
At flow rates of 0.5, 3, and 7.5μL/min, the mean wall shear stress in the square channels was estimated to be 5, 30, and 79
dyn/cm2, respectively. At the same flow rates, the mean wall shear stress was estimated to be 5.2, 31, and 78
dyn/cm in semicircular channels.2 This wall shear stress calculation provides only an estimate of the actual wall shear stress.
Immunofluorescent staining of adherens junctions, focal adhesions, and the actin cytoskeleton
After 6 days in culture, cells were fixed in situ with 2% paraformaldehyde, rinsed with phosphate-buffered saline containing 1% bovine serum albumin, permeabilized with 0.1% Triton X-100, and then immunostained with antibodies against VE-cadherin (goat anti-human VE-cadherin, 4μg/mL; Santa Cruz Biotechnology, Santa Cruz CA) and vinculin (murine anti-human vinculin, 52
μg/mL, Sigma-Aldrich) for 40
min at room temperature. Cultures incubated with goat or murine IgG served as negative immunofluorescent controls. Some cultures were stained for filamentous actin (instead of vinculin) with Alexa-488-phalloidin (Invitrogen, Inc., Eugene, OR) in combination with VE-cadherin. After washing, fluorescent secondary antibodies (Alexa-594-conjugated or Alexa-488-conjugated donkey anti-goat or anti-murine antibodies at 40
μg/mL, Invitrogen, Inc.) were added for 40
min in the dark at room temperature. Nuclei were counterstained with either TOPRO-3 or DAPI (Invitrogen, Inc.). Images were captured along the channel length and channel depth (at 0.2–0.5
μm depth intervals) using a Leica SP2 confocal microscope (Leica Microsystems, Bannockburn, IL) or a Zeiss 710 confocal microscope (Carl Zeiss MicroImaging, LLC, Thornwood, NY). All channels were imaged through their glass cover slip wall. Three-dimensional images were reconstructed from the collected image stack using Volocity software (PerkinElmer, Waltham, MA). HUVEC adhered to the bottom of the channel inlets served as an internal positive control for immunofluorescence staining.
Results
HUVEC growth in closed PDMS channels under flow conditions
At the highest flow rate of 7.5μL/min (mean wall shear stress of 79
dyn/cm2 in square channels and 78
dyn/cm2 in semicircular channels), HUVECs seeded into closed square channels formed confluent monolayers that conformed to the channel walls and thereby formed square endothelial linings (Fig. 2A, B, E, F). Three-dimensional reconstructions of image stacks indicate that adherens junctions formed on all walls of square channels, including their sidewalls (Fig. 2E). HUVEC grown under the same conditions in closed semicircular channels also produced confluent cell growth (Fig. 2C, D, G). In addition, we observed that HUVEC in both channel geometries developed focal adhesions, which firmly attach the basal layer of the cells to the extracellular matrix protein fibronectin, which was used to coat the channel walls (Fig. 2A–E). Similar results were observed at a lower flow rate of 3
μL/min, which results in a shear stress of approximately 30 and 31
dyn/cm2 in square or semicircular channels, respectively (results not shown). However, at the lowest flow rate of 0.5
μL/min, resulting in a shear stress of approximately 5
dyn/cm2 in both channels, HUVEC seeded into either channel geometry did not develop adherens junctions and immunostaining for vinculin within focal adhesions was weak or absent (Fig. 3). This was not a staining artifact because cells within the inlet demonstrated strong staining for VE-cadherin and vinculin (data not shown).
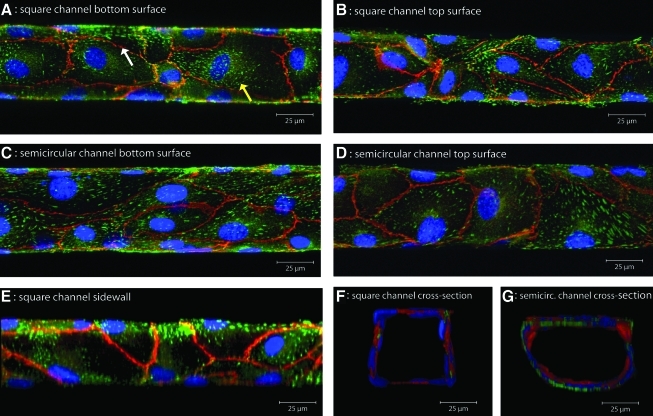
(A) Square channel bottom surface, (B) Square channel top surface, (C) Semicircular channel bottom surface, (D) Semicircular channel top surface, (E) Square channel sidewall, (F) Square channel cross-section, and (G) Semicircular channel cross-section. Maximum intensity images from z-stacks obtained with confocal microscopy of HUVEC grown at 79 and 78dyn/cm2 in closed square and semicircular PDMS channels, respectively. The cells were double immunostained for VE-cadherin (red, a marker of adherens junctions, which delineates cell boundaries) and vinculin (green, a marker of focal adhesions which are seen as dash-like structures in multifocal areas of the cells). Nuclei were counterstained with TOPRO-3 or DAPI (blue). Cells form confluent monolayers (unbroken VE-cadherin staining, left white arrow) with focal adhesions (right yellow arrow) on all channel walls. A cross-sectional view of three-dimensional reconstructions of confocal z-stacks shows that HUVEC in closed square channels conform to the channel, forming rounded profiles with slightly squared off ends (F). Reconstructed sidewall images confirm the development of adherens junctions and focal adhesions on the sidewalls of square profile channels (E). VE, vascular endothelial. Color images available online at www.liebertonline.com/tea
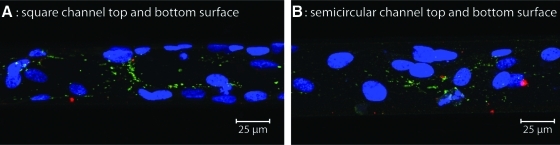
Maximum intensity images from z-stacks obtained with confocal microscopy of HUVEC grown at a mean wall shear stress of 5 and 5.2dyn/cm2 in closed square (A) and semicircular (B) channels, respectively. The cells were double immunostained for VE-cadherin (no red cell boundaries) and vinculin (few dash-like green structures). Nuclei were counterstained with TOPRO-3 (blue). Cells did not form confluent monolayers, as shown by the lack of VE-cadherin staining, and there is weak or absent immunostaining for vinculin, suggesting poor or weak formation of focal adhesions. Color images available online at www.liebertonline.com/tea
We have also observed that to obtain confluent coverage within microfluidic channels, it was necessary to use cells of very low passage numbers. While HUVEC of passages 5–11 formed adherens junctions on flat surfaces under static conditions, within microfluidic channels cells of passage numbers 8–11 lost their endothelial character and did not form adherens junctions.
HUVEC growth in open PDMS channels under static conditions
To investigate the role of geometry without the application of shear on endothelial cells, we seeded HUVEC onto open channels and characterized the endothelial linings that formed under static conditions. We used open channels because under static conditions nutrient supply to the cells in closed channels would be limited to diffusion. We examined the formation of adherens junctions and focal adhesions after 4 days of culture. When cultured under these conditions, HUVEC grew to confluence within 2–3 days in both geometries. We found that adherens junctions developed between neighboring HUVECs, which formed a semi-cylindrical shape in cross section in both geometries (Fig. 4). However, immunostaining for vinculin in focal adhesions was absent in the cells at the bottom of the square channel, whereas they were readily visible on cells that had attached to the flat upper surface of the PDMS (Fig. 5A). In contrast, cells at the bottom of the etched semi-circular channel formed distinct focal adhesions (Fig. 5B). These data suggest that focal adhesion formation is less robust in square compared to semicircular channels.
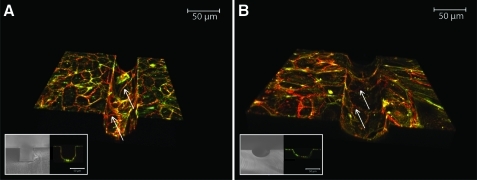
Three-dimensional reconstructions of stacks of images taken via confocal microscopy of HUVEC grown under static conditions in open square (A) and semicircular channels (B). Insets show brightfield microscopy images of the channel cross section and immunofluorescent microscopic cross-sectional images of HUVEC within the PDMS channels. Cells were immunostained for vascular endothelial (VE)-cadherin (red) and with Alexa-488-conjugated phalloidin for filamentous actin (green). Unbroken lines of VE-cadherin outline intercellular borders and indicate that the cells formed confluent monolayers in both channel geometries (arrows). Color images available online at www.liebertonline.com/tea
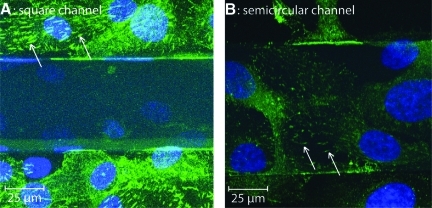
Maximum fluorescent signal projections of confocal image stacks of HUVEC grown under static conditions in open square (A) and semicircular (B) channels. The cells were immunostained for vinculin (dash-like appearance) and nuclei were counterstained with TOPRO-3 (blue). Cells at the bottom of both channels are visible via their nuclei. Focal adhesions are not seen in cells at the bottom of the open square channels, but are readily visible on cells growing on the flat top surface of PDMS (arrows). In contrast, cells in the depths of the semicircular channels form dash-like focal adhesions (arrows) to the fibronectin coating of the channel. Color images available online at www.liebertonline.com/tea
Discussion
For the first time, using confocal microscopy, we have confirmed that HUVEC can achieve confluence in 50×50μm square profile PDMS channels even on the sidewalls of the channels so that the cells form a complete in vitro lining that resembles the in vivo lining of micrometer-sized blood vessels in terms of formation of adherens junctions. High shear stresses of 30 and 79
dyn/cm2 were required to fully develop these endothelial cell structures. The absent or weak immunostaining for VE-cadherin and vinculin in HUVEC cultured at a lower shear stress of 5
dyn/cm2 suggests that the HUVEC are not fully confluent within the channels and may not respond normally to environmental stimuli. The differences in HUVEC structure observed at the lowest shear stress may be due to the low seeding density of cells within these 50
μm channels (due to their high surface area to volume ratio of the channels, the number of cells seeded per channel wall area is small). Our results suggest that shear stress above a certain threshold is necessary to populate areas that are not initially covered with cells through cell proliferation and migration. This assumption is supported by a study by Li et al. that showed that shear stress of 12
dyn/cm2 enhanced endothelial cell migration in the direction of flow.26 The lack of confluence in endothelial cells grown under low shear conditions in either channel was not evident under phase or differential contrast light microscopy (data not shown). This indicates that confluence, a prerequisite for any in vitro vascular lining, needs to be evaluated by immunostaining for markers of intercellular junctions, such as VE-cadherin, or measuring transendothelial resistance in microfluidic models of the vasculature.32
Our findings show that HUVEC grown under high shear stress within PDMS channels conformed to the channel geometry and formed confluent monolayers and firm attachments through focal adhesions to the fibronectin coating of the channel wall. This suggests that PDMS channels with traditionally fabricated square geometry can be used as in vitro models of the endothelial lining of micrometer-sized blood vessels. However, even though adherens junctions and focal adhesions were present in HUVEC cultured in square channels under high shear, the resulting cross-sectional square geometry could potentially influence subsequent endothelial behavior in response to various mechanical or chemical stimuli. However, this theory remains to be tested. Conformation of HUVEC to channel walls was the result of shear force and not fluidic pressure, as the pressure was negative since we used a syringe pump at the outlet of the channel to draw medium through the channel.
Endothelial cells grown in open square channels under static conditions form rounded monolayers (as seen in cross section). This observation suggests that endothelial cells might spontaneously form rounded structures when not exposed to shear stress. Others have observed this phenomenon when HUVEC were cultured in microenvironments such as sponge-like, three-dimensional matrixes in which shear was not present.33 In such environments, HUVEC spontaneously take on shapes, which resemble rounded tubes that support the formation of vessel-like structures. This spontaneous arrangement capability has been utilized to construct capillary-like networks in skin equivalent tissues in which endothelial cells showed characteristics associated with those of the in vivo microvessels, including expression of von Willebrand factor, development of Weibel-Palade bodies, and formation of adherens junctions.34
The formation of a confluent endothelial lining with established barrier function is a key requirement for in vitro models of the microvessels. Barrier function is frequently compromised during diseases, including inflammation and cancer metastasis.14 In vivo, adherens junctions are produced by homodimeric interactions between VE-cadherin on adjacent cells and only form when the cells are confluent. Continuous staining of VE-cadherin, which delineates cell boundaries, is a physical marker of endothelial barrier function.29 Our results showed that HUVEC cultured under static or high shear conditions in both channel geometries had strong immunostaining for VE-cadherin supporting the formation of a confluent monolayer with likely normal barrier function, regardless of channel geometry. However, under static conditions, vinculin immunostaining (as a marker of focal adhesions) was absent in HUVEC grown in square but not semicircular channels, suggesting that geometry can influence focal adhesion formation in the absence of shear. Focal adhesions are complexes consisting of various structural and enzymatic proteins, including integrins, which bind to motifs in extracellular matrix proteins such as fibronectin, which anchors the cell to the subendothelial matrix. Focal adhesions also link the extracellular matrix to the actin cytoskeleton via structural proteins such as vinculin, paxillin, and talin and to downstream signaling pathways through nonreceptor tyrosine kinases such as focal adhesion kinase and Src.35 Thus, focal adhesions not only provide structural support for cells, but are also signaling complexes that relay information from the local environment and permit cells to migrate and respond to mechanical stimuli.36 Cells lacking key proteins of focal adhesions become more mobile and are less stiff.37,38 The lack of vinculin immunostaining in HUVEC cultured in square channels under static conditions could reflect abnormal focal adhesion formation or potentially altered vinculin conformation affecting antigenic epitope exposure. It is possible that these HUVEC are weakly attached to the square channels as compared to semicircular channels and may not be able to change shape or alter their structure in response to biological stimuli. Since vinculin immunostaining was robust in HUVEC cultured in square channels under high shear stress, our findings illustrate that shear is more important than geometry in attaining a suitable in vitro microfluidic model of the microvasculature.
The shear stresses selected in this study are within the range of stresses reported within the in vivo microvasculature. Physiologic shear stress has been estimated to be in the range of 20–200dyn/cm2 in cerebral arteries between 4 and 5
mm in diameter and about 55
dyn/cm2 in retinal microvessels between 108–147
μm in diameter in humans.39,40 Similar shear stresses of 52–86
dyn/cm2 (calculated from the reported wall shear rate, assuming a blood viscosity of 3×10−3 Pa/s) have been estimated for smaller arterioles in rabbits (17–32
μm in diameter).41 Since physiological shear stress varies markedly, we suggest that endothelial cells are exposed and conditioned to shear before using in vitro microvessels for experiments.
Conclusions
We have evaluated the effect of channel geometry (square versus semicircular) and shear stress on the formation of endothelial adherens junctions and focal adhesion complexes, two junctional components of cells that are critical for endothelial cell function in vivo. We found that shear force has a greater impact on these endothelial structures than geometry in that high applied shear forces are required to produce confluent linings with robust focal adhesions, regardless of underlying channel geometry. Thus, for purposes of tissue engineering or studies of microvascular function, shear is critical for producing physiologically relevant in vitro microvessels. Channel shape influenced the final cross-sectional profile of the in vitro vascular linings formed under shear conditions, although the consequence of this altered conformation for endothelial behavior is unknown. In conclusion, both geometry and shear stress are important design parameters that should be considered when engineering in vitro endothelial linings to be used as in vitro microvascular models.
Acknowledgments
This work was performed in part at the Cornell NanoScale Facility, a member of the National Nanotechnology Infrastructure Network, which is supported by the National Science Foundation (Grant ECS-0335765). This work was supported in part by the STC Program of the National Science Foundation under Agreement No. ECS-9876771, the Consolidated Grants Program at the College of Veterinary Medicine, Cornell University, and the Cornell Center on the Microenvironment and Metastasis through Award Number U54CA143876 from the National Cancer Institute. The content is solely the responsibility of the authors and does not necessarily represent the official views of the National Cancer Institute or the National Institutes of Health. The authors acknowledge the technical assistance of Carol Bayles at the Microscopy and Imaging Facility in the Life Sciences Core Laboratories Center of Cornell University who assisted with the confocal images. The authors would also like to thank Janelle Daddona for her help with developing the protocol for immunofluorescent staining of HUVEC.
Disclosure Statement
No competing financial interests exist.
References
Articles from Tissue Engineering. Part A are provided here courtesy of Mary Ann Liebert, Inc.
Full text links
Read article at publisher's site: https://doi.org/10.1089/ten.tea.2010.0371
Read article for free, from open access legal sources, via Unpaywall:
https://europepmc.org/articles/pmc3226056?pdf=render
Citations & impact
Impact metrics
Citations of article over time
Article citations
Arched microfluidic channel for the promotion of axonal growth performance.
iScience, 27(10):110885, 04 Sep 2024
Cited by: 0 articles | PMID: 39319262 | PMCID: PMC11419798
Assessment of NK cytotoxicity and interactions with porcine endothelial cells by live-cell imaging in 2D static and 3D microfluidic systems.
Sci Rep, 14(1):24199, 15 Oct 2024
Cited by: 0 articles | PMID: 39406778 | PMCID: PMC11480498
Generation of precision microstructures based on reconfigurable photoresponsive hydrogels for high-resolution polymer replication and microoptics.
Nat Commun, 15(1):5673, 06 Jul 2024
Cited by: 0 articles | PMID: 38971797 | PMCID: PMC11227548
TPP-Based Microfluidic Chip Design and Fabrication Method for Optimized Nerve Cells Directed Growth.
Cyborg Bionic Syst, 5:0095, 09 May 2024
Cited by: 1 article | PMID: 38725973 | PMCID: PMC11079595
Bio-inspired microfluidics: A review.
Biomicrofluidics, 17(5):051503, 27 Sep 2023
Cited by: 0 articles | PMID: 37781135 | PMCID: PMC10539033
Review Free full text in Europe PMC
Go to all (37) article citations
Similar Articles
To arrive at the top five similar articles we use a word-weighted algorithm to compare words from the Title and Abstract of each citation.
Procedure for the development of multi-depth circular cross-sectional endothelialized microchannels-on-a-chip.
J Vis Exp, (80):e50771, 21 Oct 2013
Cited by: 3 articles | PMID: 24193102 | PMCID: PMC3947964
Fabrication of circular microfluidic channels by combining mechanical micromilling and soft lithography.
Lab Chip, 11(8):1550-1555, 14 Mar 2011
Cited by: 55 articles | PMID: 21399830
Microfluidic perfusion culture chip providing different strengths of shear stress for analysis of vascular endothelial function.
J Biosci Bioeng, 118(3):327-332, 03 Jul 2014
Cited by: 23 articles | PMID: 24630614
Three-dimensional co-cultures of human endothelial cells and embryonic stem cell-derived pericytes inside a microfluidic device.
Lab Chip, 13(18):3562-3568, 01 Sep 2013
Cited by: 79 articles | PMID: 23702711