Abstract
Background
Amyotrophic lateral sclerosis (ALS) is a fatal progressive motor neuron disease, for which there are still no diagnostic/prognostic test and therapy. Specific molecular biomarkers are urgently needed to facilitate clinical studies and speed up the development of effective treatments.Methodology/principal findings
We used a two-dimensional difference in gel electrophoresis approach to identify in easily accessible clinical samples, peripheral blood mononuclear cells (PBMC), a panel of protein biomarkers that are closely associated with ALS. Validations and a longitudinal study were performed by immunoassays on a selected number of proteins. The same proteins were also measured in PBMC and spinal cord of a G93A SOD1 transgenic rat model. We identified combinations of protein biomarkers that can distinguish, with high discriminatory power, ALS patients from healthy controls (98%), and from patients with neurological disorders that may resemble ALS (91%), between two levels of disease severity (90%), and a number of translational biomarkers, that link responses between human and animal model. We demonstrated that TDP-43, cyclophilin A and ERp57 associate with disease progression in a longitudinal study. Moreover, the protein profile changes detected in peripheral blood mononuclear cells of ALS patients are suggestive of possible intracellular pathogenic mechanisms such as endoplasmic reticulum stress, nitrative stress, disturbances in redox regulation and RNA processing.Conclusions/significance
Our results indicate that PBMC multiprotein biomarkers could contribute to determine amyotrophic lateral sclerosis diagnosis, differential diagnosis, disease severity and progression, and may help to elucidate pathogenic mechanisms.Free full text

Amyotrophic Lateral Sclerosis Multiprotein Biomarkers in Peripheral Blood Mononuclear Cells
Associated Data
Abstract
Background
Amyotrophic lateral sclerosis (ALS) is a fatal progressive motor neuron disease, for which there are still no diagnostic/prognostic test and therapy. Specific molecular biomarkers are urgently needed to facilitate clinical studies and speed up the development of effective treatments.
Methodology/Principal Findings
We used a two-dimensional difference in gel electrophoresis approach to identify in easily accessible clinical samples, peripheral blood mononuclear cells (PBMC), a panel of protein biomarkers that are closely associated with ALS. Validations and a longitudinal study were performed by immunoassays on a selected number of proteins. The same proteins were also measured in PBMC and spinal cord of a G93A SOD1 transgenic rat model. We identified combinations of protein biomarkers that can distinguish, with high discriminatory power, ALS patients from healthy controls (98%), and from patients with neurological disorders that may resemble ALS (91%), between two levels of disease severity (90%), and a number of translational biomarkers, that link responses between human and animal model. We demonstrated that TDP-43, cyclophilin A and ERp57 associate with disease progression in a longitudinal study. Moreover, the protein profile changes detected in peripheral blood mononuclear cells of ALS patients are suggestive of possible intracellular pathogenic mechanisms such as endoplasmic reticulum stress, nitrative stress, disturbances in redox regulation and RNA processing.
Conclusions/Significance
Our results indicate that PBMC multiprotein biomarkers could contribute to determine amyotrophic lateral sclerosis diagnosis, differential diagnosis, disease severity and progression, and may help to elucidate pathogenic mechanisms.
Introduction
Amyotrophic lateral sclerosis (ALS) is an incurable neurodegenerative disorder of unknown cause arising from progressive degeneration of motor neurons and resulting in paralysis and death, usually within 2–4 years from diagnosis. Its incidence is between 1.5 and 2.5 per 100.000 per year: approximately 90% of cases are sporadic and the remaining 10% are familial. The diagnosis is mostly based on clinical assessment with a history of progression of symptoms and is thus made with a delay of about a year from symptom onset, quite likely beyond the therapeutic window of a disease-modifying drug. Moreover, the clinical course varies widely. No ALS biomarkers are currently in clinical use, but they would be valuable to support early diagnosis, monitor disease progression, and assess the efficacy of any new treatment [1].
The pathological process in ALS is now recognized as extending beyond motor neurons [2]–[8], so it can be regarded as a multi-cellular/multi-systemic disease. In particular, peripheral blood mononuclear cells (PBMC) display traits of the disease such as down-regulation of Bcl-2 [9], [10], increased nitrative stress [11], intracellular calcium dysregulation [4] and glutamatergic dysfunction [12], suggesting that they can be a useful source of disease biomarkers.
In a complex disorder it is unlikely that an individual molecule may serve as a clinically useful biomarker. Therefore, proteomic approaches and multiple measurements are likely to be necessary to identify ALS subjects with a worthwhile degree of accuracy. In fact, the most promising candidate biomarkers have been so far combinations of proteins identified in cerebrospinal fluid (CSF) [13]–[15]. However, when the same proteins were searched in plasma either were not present or were not significantly different in comparison with controls [13], [16]. Whereas CSF is considered the ideal source for identifying biomarkers in neurological diseases because of its proximity to the affected tissue, it involves an invasive sampling, that limits large-scale validation studies and thus introduction into clinical practice. PBMC are readily accessible clinical samples and offer a series of advantages over serum/plasma and CSF. Bio-fluids have wide inter-individual variability and a broad range of protein abundance, which make them difficult to analyze by proteomic approaches [17], [18]. The cellular proteome is relatively stable, less complex to analyze and gives direct information on alterations of cellular pathways, hence insights into possible pathogenic mechanisms.
We here reported a proteome-based strategy to identify and validate disease biomarkers in PBMC. By using this procedure we found a panel of proteins that are closely associated with ALS and have high potential in clinical applications and translational medicine. Moreover, our results support the use of PBMC of sporadic ALS (sALS) patients for mechanistic studies.
Results
Proteomic analysis and validation
Figure 1 schematically shows the proteome-based strategy used to identify and validate protein biomarkers of ALS in PBMC. In the first phase, PBMC of healthy controls and sALS patients with two levels of disease severity (Table S1), low, with a ALS functional rating scale revised (ALSFRS-R) score>24 (ALS>24), and high, with a ALSFRS-R score≤24 (ALS≤24), were analyzed by 2D DIGE (Fig. 1A). The analysis, done with 11 pooled samples for each group, detected a total of 129 differential protein spots in the three experimental groups (Table S2). From these spots we used mass spectrometry to identify 71 corresponding proteins (Fig. 1B) that were the candidate protein biomarkers (Tables S3, S4). We classified these proteins in different categories based on their most recognized function (Table 1). Some of the proteins were identified in multiple spots, at lower Mw or different pI than the ones expected (Table S3). These are fragments of the protein or post-translationally modified isoforms.
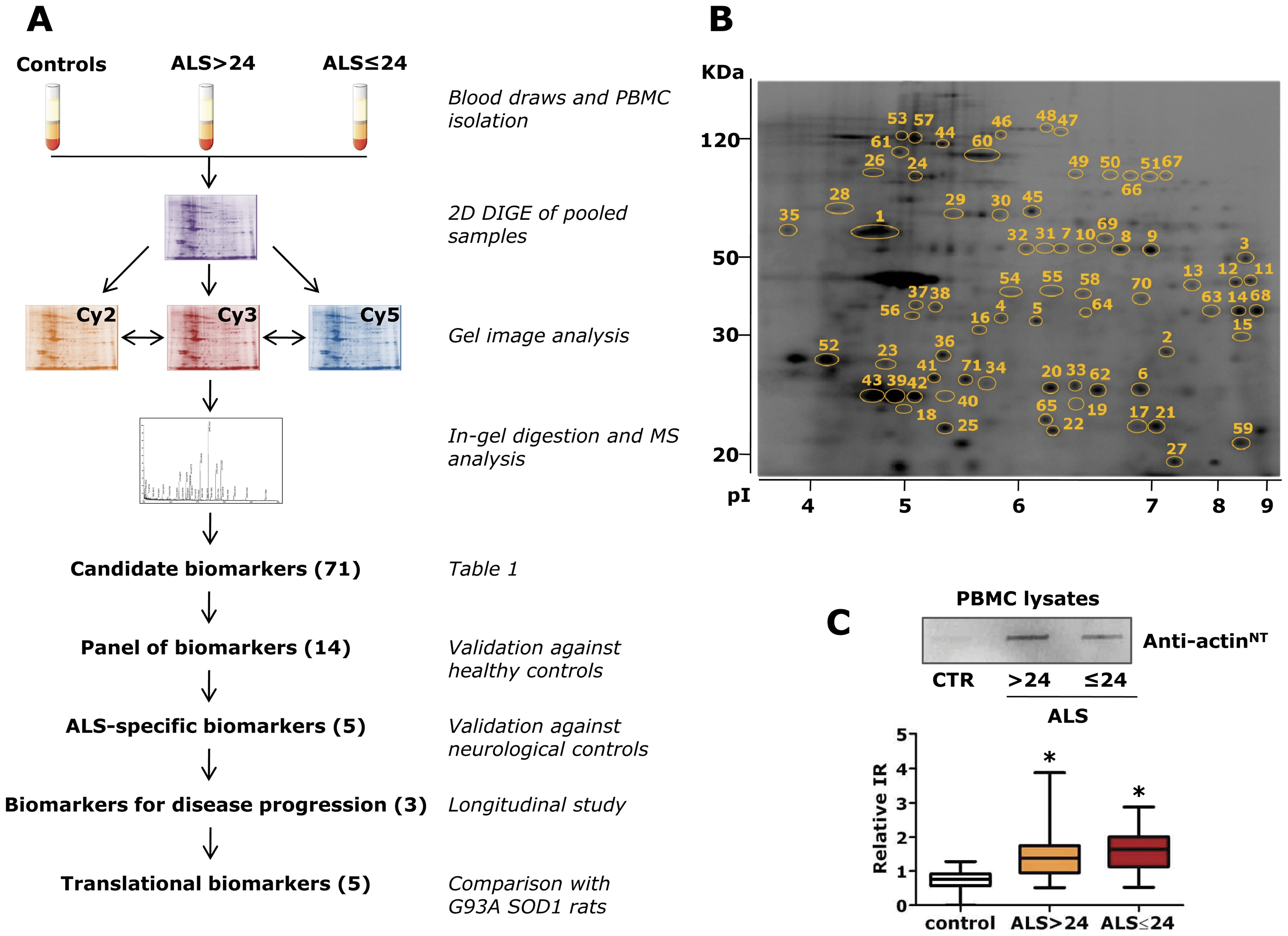
(A) PBMC of healthy controls and sALS patients with two levels of disease severity, low, ALS>24, or a high one, ALS≤24, were analyzed by 2D DIGE. Samples were pooled for analysis (n=
11 for each group). The candidate biomarkers, 71 differentially expressed proteins, were identified by mass spectrometry (MS). Validation was done on 14 proteins by dot blot analysis with single samples from an independent set of sALS patients, healthy and non-ALS neurological disorder controls. We obtained five protein biomarkers that significantly distinguish ALS patients from healthy and non ALS neurological controls and three proteins that associate with disease progression. The 14 PBMC protein biomarkers were also measured in G93A SOD1 rats: 5 of them were similarly regulated in the patients and animal model (translational biomarkers). (B) Representative Sypro-Ruby stained 2D gel of the PBMC proteome. The numbered spots correspond to the differentially expressed proteins listed in Table 1; (C) Validation by dot blot analysis was done with commercially available antibodies except for the in-house developed anti-actinNT antibody (Figures S1 and S2). A representative dot blot with the anti-actinNT antibody and quantification of the immunoreactive signals are shown. The box-plot shows relative immunoreactivity (IR) in ALS>24 (n
=
30) (yellow box), ALS 24 (n
=
30) (red box) patients and healthy controls (n
=
30) (white box). The bottom and top of the boxes are the lower and upper quartiles, respectively, the lines inside the boxes indicate the median and the ends of the whiskers represent the minimum and maximum of all the data. *p<0.05 versus controls as assessed by univariate logistic regression.
Table 1
Spot | Protein name | Uniprot1 |
Energy metabolism | ||
1 | ATP synthase subunit beta | P06576 |
2 | Triosephosphate isomerase | P60174 |
3–6 | Phosphoglycerate kinase 1 | P00558 |
7–10 | Alpha-enolase | Q8WU71 |
11–13 | Fructose-bisphosphate aldolase A | P04075 |
14 | Glyceraldehyde-3-phosphate dehydrogenase | P04406 |
15 | L- Lactate dehydrogenase A chain | P00338 |
16 | L-Lactate dehydrogenase B chain | P07195 |
Redox regulation | ||
17 | Flavin reductase | P30043 |
18 | Peroxiredoxin-2 (PRDX2) 2 | P32119 |
19 | Peroxiredoxin-6 | P30041 |
20 | Glutathione S-transferase omega-1 (GSTO1) | P78417 |
21 | Superoxide dismutase [Mn] | P04179 |
22 | Protein DJ-1 | Q99497 |
23 | Chloride intracellular channel protein 1 (CLIC1) | O00299 |
Protein folding and degradation | ||
24–25 | Heat shock cognate 71 kDa protein (HSC70) | P11142 |
26 | 78 kDa glucose-regulated protein | P11021 |
27 | Peptidyl-prolyl cis-trans isomerase A (CypA) | P62937 |
28 | Protein disulfide-isomerase (PDI) | P07237 |
29–32 | Protein disulfide-isomerase A3 (ERp57) | P30101 |
33–34 | Endoplasmic reticulum protein ERp29 | P30040 |
35 | Calreticulin (CALR) | P27797 |
36 | Proteasome activator complex subunit 1 (PA28a) | Q06323 |
Cytoskeleton-associated | ||
37–43 | Actin | P60709 |
44–48 | Vinculin | P18206 |
49–51 | Moesin | P26038 |
52 | Tropomyosin alpha-4 chain | P67936 |
53 | Alpha-actinin-1 | P12814 |
54–55 | Actin-regulatory protein CAP-G | P40121 |
56 | F-actin capping protein subunit-alpha 1 | P52907 |
57–58 | Talin-1 | Q9Y490 |
59 | Transgelin-2 | P37802 |
60 | Filamin-A | P21333 |
61 | Giantin | Q14789 |
Inflammatory response | ||
62 | Group XIIA secretory phospholipase A2 | Q9BZM1 |
63 | Annexin A2 | P07355 |
64 | Leukocyte elastase inhibitor | P30740 |
65 | Interleukin-1 receptor-associated kinase 4 (IRAK4) | Q9NWZ3 |
DNA/RNA binding | ||
66–67 | Far upstream element-binding protein 1 (FUBP1) | Q96AE4 |
68 | Heterogeneous nuclear ribonucleoproteins A2/B1 (ROA2) | P22627 |
69 | Probable ATP-dependent RNA helicase DDX41 | Q9UJV9 |
Others | ||
70 | AH receptor-interacting protein | O00170 |
71 | Spindle and kinetochore-associated protein 1 | Q96BD8 |
We then validated selected proteins by dot blot analysis on single samples of an independent set of 60 sALS patients, ALS>24 (n=
30) and ALS≤24 (n
=
30), and healthy controls (n
=
30) (Table S5). From the panel of the candidate biomarkers we validated 12 proteins (PRDX2, GSTO1, CLIC1, HSC70, CypA, PDI, ERp57, CALR, PA28a, IRAK4, FUBP1, ROA2) for the following reasons: they are also expressed in the central nervous system (CNS), they are associated with neurodegenerative processes, some of them specifically with ALS [11], [19]–[24], and are easily detectable by commercially available specific antibodies. In the same validation analysis we also measured actinNT, whose level was already found high in PBMC of a small cohort of sALS patients and G93A SOD1 transgenic rats [11] as well as in the spinal cord of the G93A SOD1 mouse model of familial ALS [25]; and TDP-43 identified as the major component of ubiquitinated inclusions in brains of patients with ALS and frontotemporal lobar degeneration [26] and found accumulated in the cytoplasm of PBMC in a group of ALS patients [27]. We confirmed that all 14 proteins can significantly distinguish healthy controls from sALS patients (Fig. 2A, Table 2, Tables S6, S7). As a negative control for the dot blot assay we used SOD1, that was one of the protein spot that did not change in the 2D DIGE analysis. Multivariate logistic analysis showed that CLIC1, actinNT and ROA2 were the proteins most associated with ALS in comparison with healthy controls, with 98% discriminatory power (AUC 0.981) (Fig. 2A). For the combination of these three proteins, the most convenient cut-off generated a sensitivity of 91% and a specificity of 96%. In all 60 sALS patients the levels of the 14 protein biomarkers were independent of sex and age.
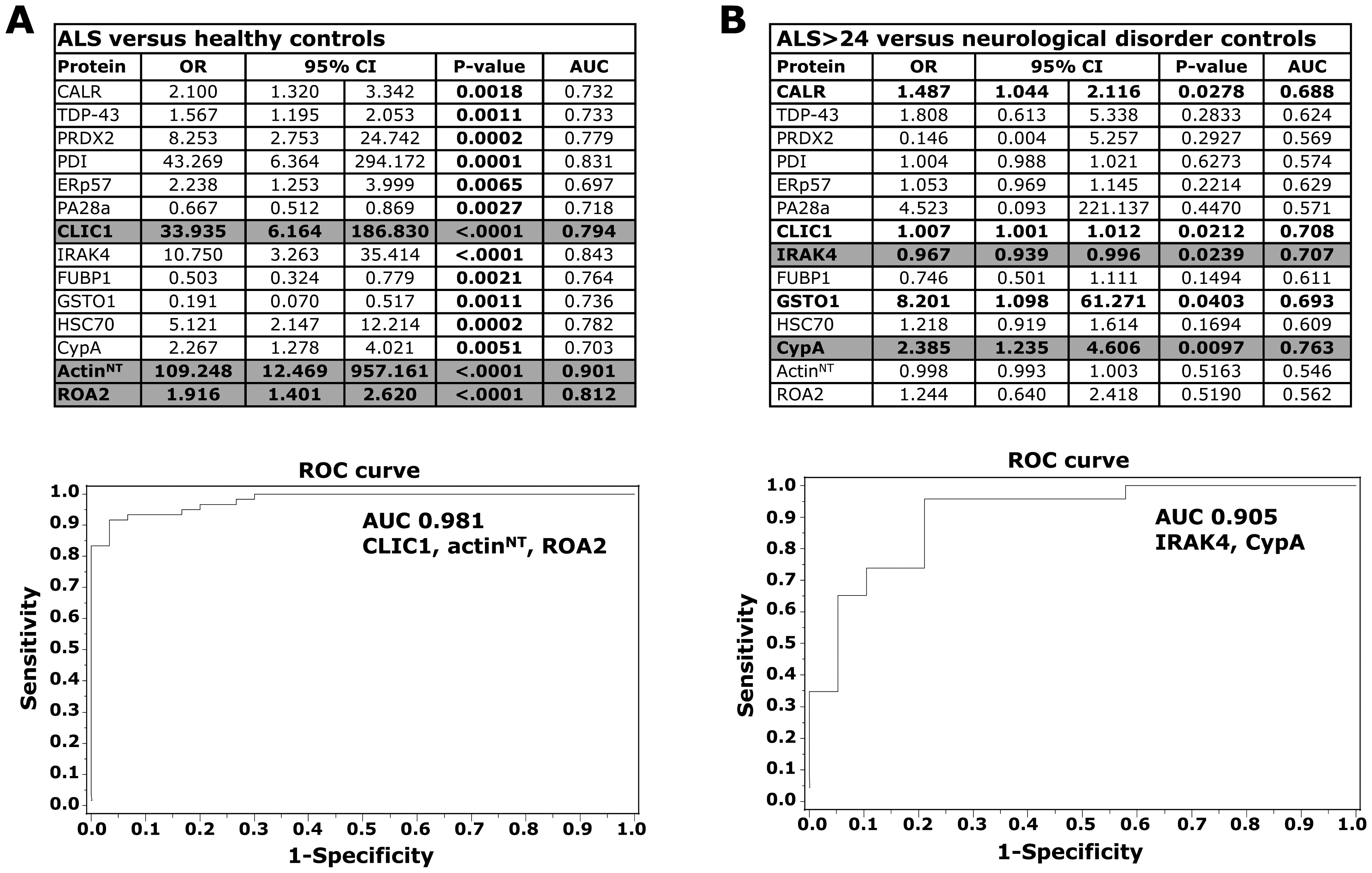
Validation on single PBMC samples from ALS patients, healthy and non-ALS neurological controls was done by dot blot analysis. Receiver operating characteristics (ROC) curves and analysis of the area under the curve (AUC) were used to find the discriminatory power for proteins showing a significant association with ALS (A,C) or with disease severity (B). Results were expressed as odds ratios (OR) and 95% confidence intervals (95% CI). A 95% CI not including the value of 1 indicates a statistically significant result. All probability values were two-sided and p<0.05 was considered significant. (A) Univariate logistic regression: healthy controls (n=
30) versus ALS, ALS>24 (n
=
30) and ALS≤24 (n
=
30). All 14 proteins were significantly associated with ALS (p values in bold type). CLIC1, actinNT and ROA2 were significant in multivariate analysis (highlighted in grey): a ROC curve for the combination of the three proteins and relative AUC is shown; (B) Univariate logistic regression: ALS>24 (n
=
20) versus non-ALS neurological controls (n
=
23). CALR, CLIC1, IRAK4, GSTO1, CypA had significant associations with ALS (in bold type). IRAK4 and CypA were significant in multivariate analysis (highlighted in grey): a ROC curve for the combination of the two proteins and relative AUC is shown.
Table 2
Protein | controls | ALS>24 | ALS≤24 | ALS>24 vs controls* | ALS≤24 vs controls* | ALS≤24 vs ALS>24* |
CALR | 2.8±0.7 | 4.9±2.8 | 4.4±2.3 | 1.8 | 1.6 | −1.1 |
TDP-43 | 4.1±1.6 | 5.2±2.1 | 6.8±2.6 | 1.3 | 1.7 | 1.3 |
PRDX2 | 0.9±0.5 | 1.4±0.7 | 2.0±1.1 | 1.6 | 2.2 | 1.4 |
PDI | 1.0±0.3 | 1.6±0.7 | 1.8±0.7 | 1.6 | 1.8 | 1.1 |
ERp57 | 2.5±0.7 | 3.8±1.4 | 4.7±2.4 | 1.5 | 1.9 | 1.2 |
PA28a | 4.1±4.5 | 1.4±1.1 | 1.6±1.6 | −2.9 | −2.6 | 1.1 |
CLIC1 | 0.4±0.3 | 0.8±0.4 | 0.9±0.5 | 2.0 | 2.3 | 1.1 |
IRAK4 | 1.0±0.5 | 2.2±1.5 | 2.4±1.5 | 2.2 | 2.4 | 1.1 |
FUBP1 | 1.7±1.5 | 0.6±0.6 | 0.9±1.0 | −2.8 | −1.9 | 1.5 |
GSTO1 | 1.2±0.7 | 0.7±0.5 | 0.6±0.4 | −1.7 | −2.0 | −1.2 |
HSC70 | 0.8±0.5 | 1.7±1.2 | 2.3±2.3 | 2.1 | 2.9 | 1.4 |
CypA | 1.4±0.7 | 3.4±2.7 | 2.0±1.2 | 2.4 | 1.4 | −1.7 |
ActinNT | 0.7±0.3 | 1.5±0.8 | 1.6±0.6 | 2.1 | 2.3 | 1.1 |
ROA2 | 2.9±1.8 | 5.7±2.7 | 6.4±3.0 | 2.0 | 2.2 | 1.1 |
SOD1 | 0.3±0.1 | 0.3±0.2 | 0.3±0.1 | 1.0 | 1.0 | 1.0 |






CALR, CLIC1, IRAK4, GSTO1 and CypA are ALS-specific biomarkers
We verified the specificity of the 14 biomarkers with PBMC samples from patients with neurological disorders that in some cases may resemble ALS, e.g. some peripheral neuropathies (Table S8). We analyzed our multiprotein biomarkers by dot blot in ALS>24 patients (n=
20) and controls with neurological disorders (n
=
23) (Table 3). Univariate logistic analysis showed that CALR, CLIC1, IRAK4, GSTO1 and CypA were associated with ALS (Fig. 2B). Multivariate logistic analysis showed that IRAK4 and CypA were the proteins most associated with ALS rather than neurological disorders, with 91% discriminatory power (AUC 0.905) (Fig. 2B). For the combination of these two proteins, the most convenient cut-off generated a sensitivity of 96% and a specificity of 79%. It is interesting to note that an equally high level of TDP-43 was also detected in patients with neurological disorders (Table 3).
Table 3
Protein | controls | ALS>24 | ALS>24 vs NC* |
CALR | 4.9±2.8 | 3.2±1.3 | −1.5 |
TDP-43 | 2.2±0.6 | 2.0±0.5 | −1.1 |
PRDX2 | 0.5±0.2 | 0.6±0.2 | 1.2 |
PDI | 0.9±0.4 | 0.8±0.4 | −1.1 |
ERp57 | 0.1±0.1 | 0.1±0.1 | 1.0 |
PA28a | 0.3±0.2 | 0.3±0.2 | 1.0 |
CLIC1 | 2.9±2.1 | 1.5±0.8 | −1.9 |
IRAK4 | 0.7±0.2 | 1.0±0.4 | 1.4 |
FUBP1 | 2.0±1.3 | 2.8±2.0 | 1.4 |
GSTO1 | 0.8±0.6 | 0.5±0.3 | −1.6 |
HSC70 | 3.4±2.6 | 2.3±2.2 | −1.5 |
CypA | 4.5±2.2 | 2.6±1.0 | −1.7 |
ActinNT | 2.8±1.3 | 3.0±1.2 | 1.1 |
ROA2 | 1.9±0.9 | 1.7±1.0 | −1.1 |




CypA, TDP-43 and ERp57 as biomarkers for disease progression
We found that CypA, TDP-43 and ERp57 levels significantly differed in ALS>24 and ALS≤24 patients (Figure 3, Table 2), indicating that these proteins can discriminate between patients with high and low disease severity. Multivariate logistic analysis showed that ERp57 was the most associated with more severe ALS, with 89% discriminatory power (AUC 0.893) (Fig. 3). For ERp57, a threshold of 2.7 relative immunoreactivity generated a sensitivity of 93% and a specificity of 70%. We next examined in a pilot longitudinal study whether CypA, TDP-43 and ERp57 changed over time. We collected three longitudinal PBMC samples from an independent set of 13 sALS patients over a period of six months and used a statistical model for repeated measures to assess the effect of time on protein levels, detected by dot blot assay. The patients had on average a disease duration of 21±10 months, a 33±5 ALSFRS-R score at the first draw and a 28±7 ALSFRS-R score at the third draw (Table S9). The level of all the three protein biomarkers showed a significant increase within six months from the first PBMC collection and for TDP-43 already within 3 months (Fig. 4). As a negative control, in the same set of samples we measured SOD1, which did not significantly change over time (Fig. 4).
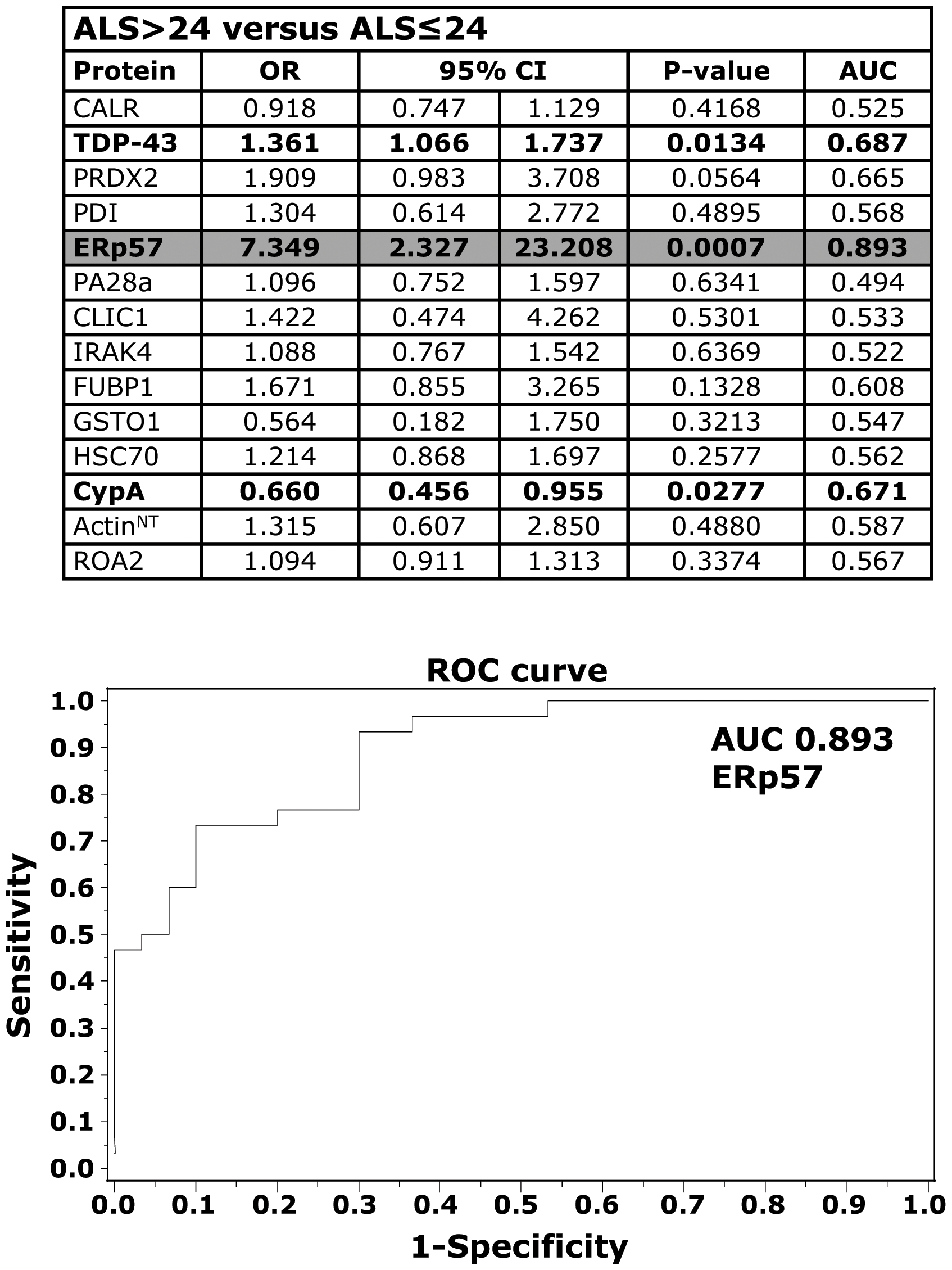
Analyses of the 14-protein biomarkers were done on single PBMC samples from ALS patients, ALS>24 (n=
30) and ALS≤24 (n
=
30), by dot blot analysis. ROC curves and analysis of the area under the curve (AUC) were used to find the discriminatory power for proteins showing a significant association with disease severity. Results were expressed as odds ratios (OR) and 95% confidence intervals (95% CI). A 95% CI not including the value of 1 indicates a statistically significant result. All probability values were two-sided and p<0.05 was considered significant. Univariate logistic regression: TDP-43, ERp57 and CypA gave a significant association with disease severity (in bold type). Multivariate analysis indicated that ERp57 was the protein most closely associated with disease severity (highlighted in grey). A ROC curve for ERp57 is shown.
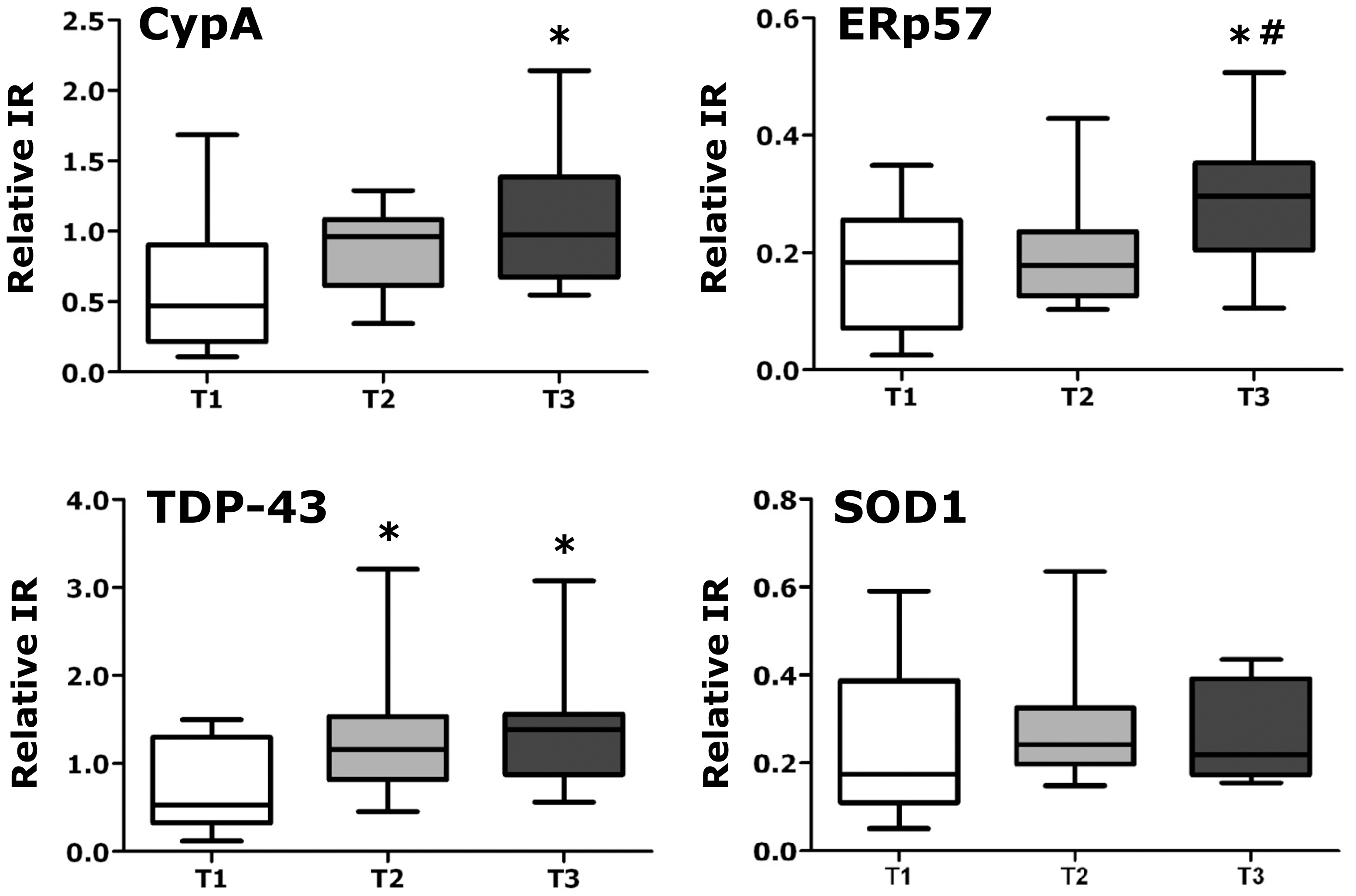
Graphs show relative immunoreactivity (IR) for the indicated proteins measured by dot blot assay in PBMC of sALS patients (n=
13) over a period of six months. PBMC were collected at t
=
0 (T1, white column), at t
=
3 months (T2, grey column) and at t
=
6 months (T3, black column). SOD1 was used as a negative control. Immunoreactivity was normalized to the actual amount of protein loaded, detected after Red Ponceau staining. Data are the means ± SEM of relative immunoreactivity. *, significantly different (p<0.05) from T1 as assessed by repeated measures ANOVA followed by Tukey's multiple comparison test; #, significantly different (p<0.05) from T2 as assessed by repeated measures ANOVA followed by Tukey's multiple comparison test.
CypA, GSTO1, FUBP1, CLIC1 and actinNT are translational biomarkers of ALS
Translational biomarkers are molecules that can be assessed in both human and animal models. To test whether our 14 PBMC proteins were translational biomarkers, their level was measured in PBMC of a transgenic G93A SOD1 rat model of ALS, at pre-symptomatic and symptomatic stages of the disease, in comparison with samples from non transgenic rats by immunoblotting. Five out of fourteen protein biomarkers, CypA, GSTO1, FUBP1, CLIC1 and actinNT, showed similar changes in PBMC of sALS patients and transgenic rats (Fig. 5). It is interesting to note that FUBP1 was one of the least specific ALS biomarkers in PBMC of sporadic patients. It is possible that this protein is more associated with SOD1 mutation-induced alterations and is more informative for the mutant SOD1 fALS forms. The translational biomarkers were also investigated in the rat spinal cord at a presymptomatic and symptomatic stages of the disease (Fig. 5). Lumbar spinal cords were sectioned in ventral and dorsal horns to investigate whether proteins alterations were associated or not with motor neuron dysfunction and degeneration. In ventral horns, all of them changed similarly to PBMC already at a presymptomatic stage except for CLIC1, up-regulated only at a symptomatic stage. In dorsal horns, the proteins did not change with the progression of the disease and if compared with controls (Figure S3), indicating that protein alterations observed in ventral horns are specifically associated with motor neuron pathology. In conclusion, CypA, GSTO1, FUBP1, CLIC1 and actinNT are translational biomarkers that might be markers of multi-cellular/multi-systemic alterations underlining pathogenic events both in the human sporadic and animal mutant SOD1-linked disease forms.
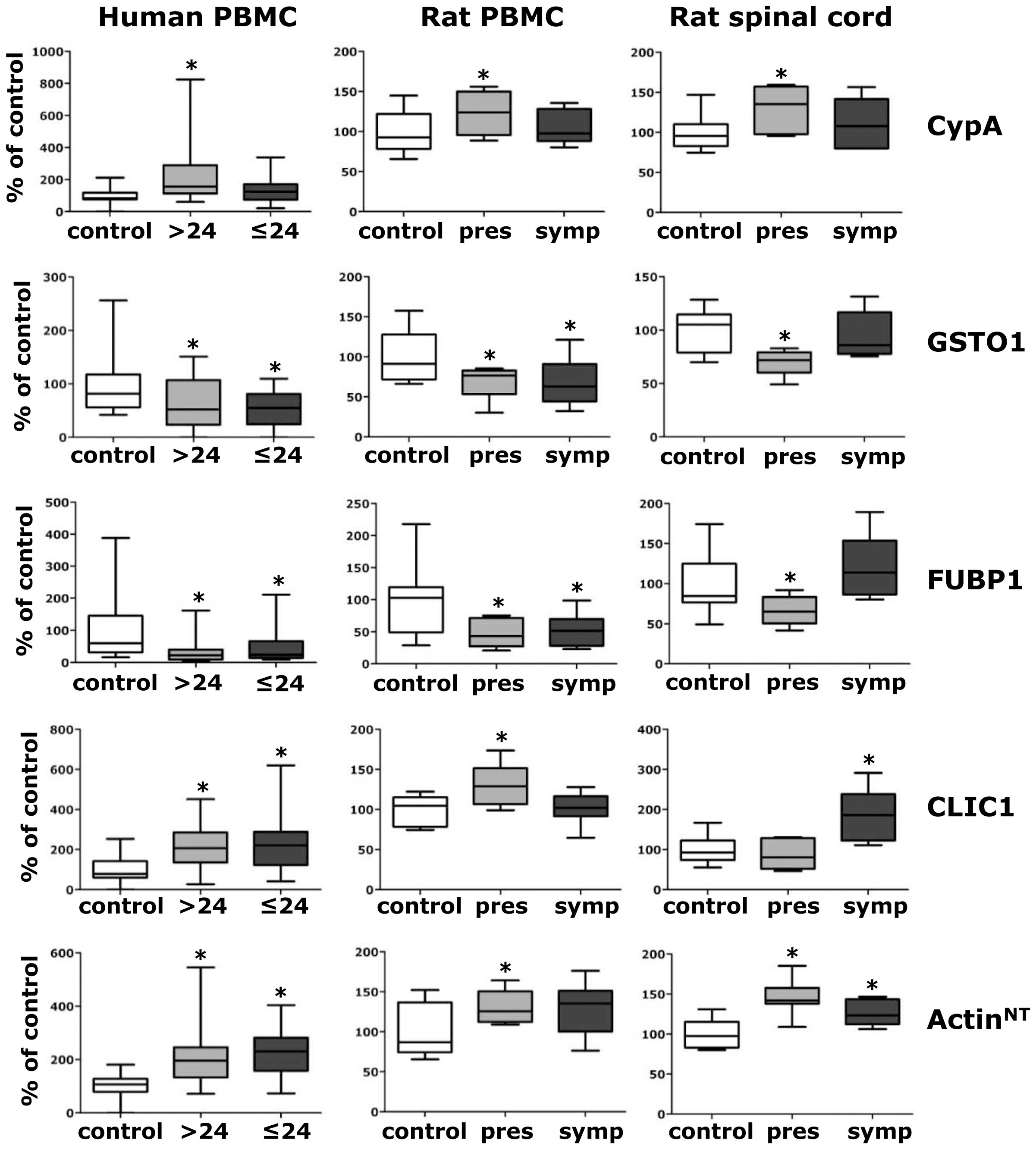
Human PBMC (left column): box-plot shows relative immunoreactivity for the indicated proteins measured by dot blot assay in ALS>24 (n=
30) (grey box) and ALS≤24 (n
=
30) (dark grey box) patients as percentage of healthy controls (control) (n
=
30) (white box). *, significantly different (p<0.05) from control (univariate logistic regression). Rat PBMC (middle column): box-plot shows relative immunoreactivity for the indicated proteins measured by WB (except for actinNT, measured by dot blot) in PBMC lysates (15 µg) of presymptomatic (pres) (n
=
6) and symptomatic (symp) G93A SOD1 (n
=
6) rats as percentage of non transgenic (control) (n
=
8) rats. Protein level values were normalized to actin loading control. *, significantly different (p<0.05) from controls (one-way ANOVA followed by Newman-Keuls multiple comparison test). Rat spinal cord (right column): box-plot shows relative immunoreactivity for the indicated proteins measured by dot blot in ventral horn tissue lysates of presymptomatic (pres) (n
=
6) and (symp) G93A SOD1 (n
=
6) rats as percentage of non transgenic (control) (n
=
8) rats. Immunoreactivity was normalized to the actual amount of protein loaded, detected after Red Ponceau staining. *, significantly different (p<0.05) from control (one-way ANOVA followed by Newman-Keuls multiple comparison test).
Discussion
A number of gene expression studies have demonstrated the utility of PBMC as a source of biomarkers in neurological disorders [28]–[30]. This is the first study that describes a highly feasible strategy to identify and validate multiprotein biomarkers in PBMC, potentially applicable to several neurological/neurodegenerative diseases. Protein profiles are directly connected to changes in molecular pathways related to health and disease, therefore have the potential to accurately monitor the progression of the disease or the response to a treatment.
We have previously shown that PBMC undergo immunophenotypic changes in sALS patients [10]. We have now identified a panel of protein biomarkers in these cells that are associated with sALS with high discriminatory power. These PBMC proteins are easily measurable in large-scale immunoassays aimed at developing diagnostic/prognostic tests for clinical use. The great advantages of such an in vitro test is low invasiveness for the patient compared to CSF tests, the consequent greater availability of samples for large clinical studies, including longitudinal ones, and the simple laboratory procedures involved.
The ideal diagnostic marker should detect disease before clinical diagnosis, which is highly challenging for a rare and sporadic disease. ALS patients very often see the specialized neurologist only months after the first symptoms, when they are unquestionably ill. It is therefore very difficult to test and validate the applicability of biomarkers in preclinical diagnosis. Our PBMC protein biomarkers seems to be promising to support prompt clinical diagnosis, since all the 14 validated proteins can distinguish with high significance ALS patients with low disability from healthy controls (Table S6). PBMC biomarkers could be measured on patients after as early as 5 months up to 108 months from symptom onset, and with high (from 45) and low (up to 10) ALSFRS-R scores (Table S5, S8, S9). Now large-scale validations are needed, in which data will be verified in a prospective cohort with analysis of the biomarkers at the time of symptom presentation. In the G93A SOD1 rat model of ALS, alterations of the level of CypA, GSTO1, FUBP1, CLIC1 and actinNT are detected before disease onset. It is possible that some of these biomarkers may predict the onset of SOD1-linked familial ALS. This potential application will be also considered in future analysis.
Up to 10% of patients initially diagnosed as having ALS are false positive [31], [32]. A similar percentage is false negative and undergoes inappropriate medical or surgical procedures [33], [34]. Thus, there is a need for biomarkers that distinguish ALS with high accuracy from neurological disorders that in some cases may resemble it, e.g. some peripheral neuropathies that are treatable and do not have a fatal prognosis. We found that there are PBMC protein biomarkers that significantly distinguish ALS from the group of neurological disorders considered in our study. These are chaperones (CALR, CypA), proteins involved in redox homeostasis (GSTO1, CLIC1) and immune responses (IRAK4). IRAK4, which has no previous association with ALS, has a central function in innate immunity [35].
Finally, multivariate analysis helped us in defining the most convenient combination of protein biomarkers that could be potentially used in clinics (i) to support diagnosis (CLIC1, actinNT and ROA2), (ii) to contribute to differential diagnosis of ALS from other neurological conditions (CypA and IRAK4), and (iii) to determine disease severity (ERp57).
There are no precise measures of ALS disease progression that allow for short-term monitoring of the disease and assessment of treatment efficacy. In clinical trials survival time is therefore used as the primary measure of outcome. This requires large number of patients followed over a long period of time making ALS clinical trials very expensive. A panel of biomarkers that can reliably assess disease progression would enable a substantial reduction of the costs of the clinical trial and accelerate therapy development in ALS. ERp57, CypA and TDP-43, that were able to discriminate between patients with high and low disease severity, were selected for a pilot longitudinal study and proved to be good candidates for such applications. Large longitudinal studies are now needed to further validate the use of these proteins in clinical practice.
The pathogenesis of sALS is largely unknown. The concept of PBMC as a window into the CNS has been already proposed for several neurological disease states [28]–[30], [36], [37]. CNS and immune cells communicate through multiple mechanisms and have several similarities in receptor expression and transduction processes [38]. We therefore hypothesized that PBMC protein profiles could help to elucidate pathways underlying ALS etiology. Indeed, some of the protein biomarkers identified in PBMC of sALS patients were previously found as hallmarks of disease in CNS. Studies in spinal cord tissues of sALS patients showed that PDI and ERp57 were up-regulated [20], CypA and HSC70 were accumulated in the detergent-insoluble fraction [39], HSC70 was present in hyaline inclusions [24], and TDP-43 was identified as the major component of the ubiquitinated inclusions [26].
The protein profile changes detected in PBMC of ALS patients are suggestive of possible pathogenic mechanisms. For instance, up-regulation of endoplasmic reticulum (ER) chaperones (PDI, ERp57, CALR) is a typical cellular response to ER stress that triggers the unfolded protein response leading eventually to cell death [40]. The increased level of a nitrotyrosine-linked protein, actinNT, is indicative of nitrative stress [41]. Alterations in CypA, GSTO1, PRDX2 suggest disturbances in cellular redox regulation [42]–[45]. It is important to note that all these pathogenic alterations were previously reported in the spinal cord of sALS patients and mutant SOD1 animal models [19], [20], [25], [46]–[49]. ROA2 belongs to the family of heterogeneous nuclear ribonucleoproteins that participates to several RNA-related biological processes such as transcription, pre-mRNA processing, mRNA transport to the cytoplasm and translation [50]. It is also a binding partner of TDP-43 and seems to be crucial for at least one of its putative functions [51]. The up-regulation of ROA2 and TDP-43 in PBMC of both ALS>24 and ALS≤24 patients may underline aberrant RNA processing events that are now emerging as central in ALS and other neurological disorders [52]. These specific intracellular alterations would not be detectable from the protein profiles of CSF or plasma. Neuroinflammation, which is characterized by activated microglia and infiltrating peripheral blood immune cells, is a prominent pathological feature in ALS [53]. It is possible to speculate that such consistent protein profile changes in immune cells may influence their infiltration and/or interaction with microglia and neurons, thus upsetting the delicate balance between neuroprotection and neurotoxicity. In summary, all these data endorse the use of PBMC for further in vitro mechanistic studies. Experimental models for the sporadic form are not available and all mechanistic studies are done with transgenic cells and animals expressing one of the mutant genes linked to familial ALS. Studies with PBMC would have the advantage to consider the influence of the genetic background of the patient.
Translational biomarkers, that link responses between human and animal model, are of particular interest because their role in the pathogenesis can be investigated in detail in the animal model where they can also offer important preliminary information for clinical trials. We found that CypA, GSTO1, FUBP1, CLIC1 and actinNT are translational biomarkers. Moreover, all of them except for CLIC1 are altered in the ventral horn spinal cord of SOD1 G93A rats before disease onset, suggesting a possible involvement in pathways that trigger the disease. Further mechanistic studies in the animal models with these proteins are now warranted.
In conclusion, we identified and verified a panel of highly promising protein biomarkers of ALS in PBMC that may be useful in clinical studies, helping elucidate pathogenic mechanisms and pin-pointing pathways to tackle for future therapeutic interventions. The fact that our protein biomarkers are easily measurable in accessible clinical samples using straightforward immunoassays makes them attractive candidates for true multi-centric large-scale validations and eventually introduction into clinical practice.
Materials and Methods
Subjects
The study was approved by the Ethical Committees of all the Centers involved in the study, IRCCS Fondazione S. Maugeri, in Milano and Pavia, NEMO-Niguarda Ca’ Granda Hospital, Milano, and Transfusion Medical Centre at the IRCCS Policlinico S. Matteo, Pavia, and written informed consent was obtained from all participating subjects. In this study we included 94 sALS patients and 64 controls (41 healthy, 23 with non-ALS neurological disorders). Patients with definite ALS, according to revised El Escorial criteria, were examined and blood samples were collected at the IRCCS Fondazione S. Maugeri, in Milano and Pavia, Italy. To evaluate the level of disability of the patients the ALSFRS-R score was used [54]. The patients were arbitrary divided into two groups according to the ALSFRS-R score, >24 or ≤24. None of the sALS patients had systemic inflammatory conditions as detected by the erythrocyte sedimentation rate and total blood cell count. Sixty-five percent of the patients were treated with riluzole. For the longitudinal study PBMC samples were collected every three months over a period of six months at the NEMO, Niguarda Ca’ Granda Hospital. Blood samples of non-ALS neurological disorder controls were provided by the NEMO and the IRCCS Fondazione S. Maugeri, in Milano and Pavia. None of the patients was receiving drugs that might interfere with total blood cell count. The characteristics of ALS patients and non-ALS neurological disorder controls are further described in Tables S1, S5, S8 and S9. Blood samples of healthy donors were provided by the Transfusion Medical Centre at the IRCCS Policlinico S. Matteo, Pavia.
PBMC
Samples of peripheral venous blood from patients and controls were collected in EDTA pre-coated vials (Vacuette K3E K3EDTA, Greiner bio-one). PBMC were isolated from EDTA-blood by Ficoll-Hypaque (Ficoll-Plaque™ Plus, GE Healthcare) density gradient centrifugation. Mononuclear cells were harvested from the interface and washed three times with RPMI 1640 medium (EuroClone). Platelets were eliminated by an additional wash and centrifugation at 200× g for 10 min. Patients' and controls' PBMC were stored as pellets at −80°C. Just before analysis PBMC proteins were obtained by cell lysis in 20 mM Tris-HCl pH 7.5, 0.1% NP40 and 0.1% SDS supplemented with Protease Inhibitors (Sigma). Proteins were quantified by the BCA protein assay (Pierce).
Two-dimensional difference in gel electrophoresis (2D DIGE)
PBMC proteins were prepared for 2D DIGE analysis. Three pools of 25 µg from 11 healthy controls, 11 ALS>24 and 11 ALS≤24 patients were methanol-precipitated. Proteins were then dissolved in 30 mM Tris-HCl pH 8.5, 7 M urea, 2 M thiourea, CHAPS 4% (w/v) and Cydye-labeled according to the manufacturer's instructions (GE Healthcare) with minor modifications. Briefly, 25 µg of each pool was labeled with 200 pmol of Cy3 or Cy5 dye for 30 min in ice in the dark. To exclude preferential labeling of the dyes, each sample was also reverse labeled. As an internal standard, aliquots of each pool were mixed and labeled with Cy2 dye. Labeled samples were then resuspended in Destreak Solution™ (GE Healthcare) with IPG buffer pH 3–10 NL 0.5% v/v (GE Healthcare) added and loaded into 7 cm-IPG strips pI range 3–10NL (GE Healthcare). Isoelectrofocusing was done on an IPGphor apparatus (GE Healthcare) with the following protocol: 30 V for 270 Vhrs, 200 V for 200 Vhrs, 2000 V for 2000 Vhrs, a linear gradient of 3500 V for 1375 Vhrs, 3500 V for 7000 Vhrs, a linear gradient of 8000 V for 8625 Vhr, 8000 V for 32000 Vhr and forever at 30 V. SDS-PAGE was done using precast 10% polyacrylamide SDS gel (Invitrogen). Four 2D gels were run with the three experimental groups: healthy controls and ALS patients, ALS>24, and ALS≤24. Each gel contained two experimental groups, one Cy3-labelled, the other Cy5-labelled plus the Cy2-labelled internal standard. Gel images were captured by the laser scanner Molecular Imager FX (Bio-Rad). Image analysis was done with Progenesis PG240 v2006 software (Nonlinear Dynamics). For each spot the normalized volume was standardized against the intra-gel standard, dividing the value for each spot normal volume by the corresponding internal standard spot normal volume within each gel. The values for each spot in each group were expressed as the mean of the Cy3- and Cy5-labelled analyses. The values for ALS patients were reported as fold change: higher (positive) or lower (negative) spot volume of the samples from ALS patients in comparison with healthy controls (Table S4).
Protein identification
Protein spots were located and excised from 2D gels with the EXQuest™ spot cutter (Bio-Rad). Spots were processed and gel-digested with modified trypsin from bovine pancreas (Roche) and identified by mass spectrometry (MS), essentially as previously described [25]. Digestion, desalting and concentration with ZipTip® pipette tips with C18 resin (Millipore) and MALDI target deposition were carried out on an automated protein digestor DigestPro MS (Intavis AG). Peptide mass fingerprinting and tandem mass spectrometry (MS/MS) were done on a 4800 MALDI TOF/TOF mass spectrometer (Applied Biosystems). The mass spectra were internally calibrated with trypsin autolysis fragments. The five most abundant precursor ions, out of the exclusion mass list (ions from human keratin and trypsin), were selected for MS/MS analysis. The combined MS and MS/MS data were submitted by GPS Explorer v.3.6 software (Applied Biosystems) to the MASCOT database search engine (Version 2.1, Matrix Science) and searched with the following parameters: Uniprot_Swissprot 57.8 database over all Homo sapiens protein sequences deposited, no fixed modifications, as possible modifications carboamidomethylation of cysteine and oxidation of methionine, 1 missed trypsin cleavage, a mass tolerance of ±0.1 Da for the peptide masses and ±0.3 Da for the MS/MS fragment ion masses. A protein was regarded as identified if the MASCOT protein score, based on the combined MS and MS/MS data, was above the 5% significance threshold for the database [55]. Some of the proteins were identified by liquid chromatography-(LC)-MS/MS using the microfluid chip-based technology for nanoelectrospray coupled to an ion trap mass spectrometer (Agilent 1200 LC/MSD Trap XCT) as previously described [56]. Data were acquired sequentially in MS mode (scan range 200–2000 amu) and in data-dependent mode, automatically recording the MS/MS spectra of the four most abundant ions in every scan cycle. Data files of all MS/MS spectra in a LC run were merged, and submitted as an “mgf” file (BioWorks Rev 3.1 SR1, Thermo Scientific) to the MASCOT database search engine in MS/MS Ion Search mode. A protein was regarded as identified if MASCOT individual ion scores were >36, which indicate identity or extensive homology (p<0.05), for at least three matched peptides. Search parameters were the same as above.
Anti-nitrated actin (actinNT) antibody preparation
Purified human non-muscle actin (>99% pure; Cytoskeleton, Inc.) (1.5 mg/mL) was incubated for 24 hours at room temperature in a nitration solution containing 20 mM sodium acetate pH 5.6, 9 µM FeCl3, 10 mM NaNO2, 0.3% H2O2. Nitration of the protein was verified by Western blotting (WB) with the anti-nitrotyrosine antibody (clone HM.11; Hycult Biotechnology). ActinNT was used as antigen in rabbits for raising polyclonal antibodies at Eurogentec S.A. LIEGE Science Park (Belgium). Anti-actinNT antibody was characterized as described in Figures S1 and S2.
Dot blot analysis
Aliquots (3 µg) of PBMC samples were loaded on nitrocellulose membrane, Trans-Blot Transfer Medium (Bio-Rad), by vacuum deposition on the Bio-Dot SF blotting apparatus (Bio-Rad). Membranes were probed overnight with primary antibodies: rabbit polyclonal anti-CALR (15000), rabbit polyclonal anti-PDI (1
4000) and mouse monoclonal anti-ERp57 (1
500) from StressGen, mouse monoclonal anti-HSC70 (1
1000), goat polyclonal anti-PRDX2 (1
2000), goat polyclonal anti-PA28a (1
2000), mouse monoclonal anti-CLIC1 (1
2500), goat polyclonal anti-IRAK4 (1
1000) and rabbit polyclonal anti-FUBP1 (1
1000) from Santa Cruz Biotechnology, mouse monoclonal anti-GSTO1 (1
500) and mouse monoclonal anti-ROA2 (1
2500) from Abnova, rabbit polyclonal anti-CypA (1
2500) and rabbit polyclonal anti-hSOD1 (1
2000) from Upstate Biotechnology, rabbit polyclonal anti-actinNT (1
7500) developed in-house, and rabbit polyclonal anti-TDP-43 (1
2000), kindly provided by Francisco Baralle, ICGEB, Trieste, Italy, and then with secondary anti-mouse, anti-rabbit or anti-goat peroxidase-conjugated secondary antibodies (Santa Cruz Biotechnology). Blots were developed by Immobilon Western Chemiluminescent HRP Substrate (Millipore) on the ChemiDoc XRS system (Bio-Rad). Densitometry was done with Progenesis PG240 v2006 software (Nonlinear Dynamics). Immunoreactivity was normalized to the actual amount of proteins loaded on the membrane as detected after Red Ponceau staining (Fluka).
Statistical analysis
Continuous variables such as age and all data of the considered proteins were described using ‘standard’ statistics (mean, standard deviation), and relative and absolute frequencies were used for categorical variables. Spearman correlation analysis was done to assess the associations between the 14 proteins. Univariate and multivariate logistic regression models were also built to identify the level of association between proteins and ALS patients. Receiver operating characteristics (ROC) curves and analysis of the area under the curve (AUC) were used to find the discriminatory power for proteins showing a significant association with ALS or disease severity. Results were expressed as odds ratios (OR) and 95% confidence intervals (95% CI). A 95% CI not including the value of 1 indicates a significant result. Dependence on sex and age (at the time of PBMC collection) was analyzed by two-way ANOVA. All probability values were two-sided and p<0.05 was considered statistically significant. Version 9.1 of the SAS statistical software (SAS Institute, Inc, Cary, NC, USA) and version 5.03 of the GraphPad Prism software (GraphPad Software, Inc, La Jolla, CA, USA) were used.
Analysis of protein biomarkers in the rat model
Procedures involving animals and their care were conducted in conformity with the institutional guidelines that are in compliance with national (D.L. No. 116, Suppl. 40, Feb. 18, 1992 Circolare No. 8, G.U., 14 luglio 1994) and International laws and policies (EEC Council Directive 86/609. OJ L 358,1, Dec. 12, 1987; NIH Guide for Care and use of Laboratory Animals, U.S. National Research Council, 1996). They were reviewed and approved by the Mario Negri Institute Animal Care and Use Committee that includes ad hoc members for ethical issues (ID 33/01-D Appl3). Non transgenic and transgenic rats expressing a high copy number of mutant human SOD1 with Gly-93-Ala substitution were bred and maintained at the Mario Negri Institute for Pharmacological Research, Milan, Italy. Animals were housed at 21±1°C with relative humidity 55±10% and 12 h of light. Food (standard pellets) and water were supplied ad libitum. Transgenic rats were identified with PCR on DNA from tail biopsies. G93A SOD1 rats were sacrificed at presymptomatic (15 weeks of age) and early-symptomatic (20 weeks of age) stages of disease. Non transgenic rats were used as controls (20 weeks of age). Blood was sampled directly by intracardiac puncture from rats and collected in EDTA pre-coated vials (Vacuette K3E K3EDTA, Greiner bio-one). PBMC were isolated from blood, washed and stored as pellets at −80°C in the same way as the human samples. PBMC proteins were obtained by cell lysis in 50 mM Tris-HCl, pH 7.5, 2% (w/v) CHAPS, 37.5 U benzonase (Merck), supplemented with Protease Inhibitors (Sigma). Proteins were quantified by the BCA protein assay (Pierce). Proteins were separated by SDS-PAGE on precast 12% Criterion XT Bis-Tris gels (Bio-Rad) and immunoblotted, as described [39]. The blots were probed with primary and secondary antibodies, as described for dot blot assay of the human samples. Blots were also probed with an antibody that recognizes actin (mouse monoclonal, 11000 dilution, Chemicon) for loading control and developed by Immobilon Western Chemiluminescent HRP Substrate (Millipore) on the ChemiDoc XRS system (Bio-Rad). Densitometry was done with Progenesis PG240 v2006 software (Nonlinear Dynamics). Spinal cords were removed and the lumbar tract was cut into ventral and dorsal horn sections on a cryostat. Ventral and dorsal horn sections were suspended in 1% SDS (5 µL/mg), sonicated and boiled for 10 min. Homogenates were centrifuged at 12000 RPM and supernatants analyzed by dot blot, as described for the human samples except for the FUBP1 and PA28a analyses where the mouse monoclonal anti-FUBP1 antibody (1
500) from Santa Cruz Biotechnology and the rabbit polyclonal anti-PA28a antibody (1
1000) from Cell Signaling were used.
Supporting Information
Figure S1
Anti-nitrated actin (actinNT) antibody preparation and characterization by dot blot assay. Purified human actin was nitrated in vitro and used as antigen in rabbits for raising polyclonal antibodies. The rabbit polyclonal antiserum was tested by dot blot with actin, actinNT, bovine serum albumin (BSA) and BSANT, prepared by the same procedure as actinNT. Figure S1 shows that anti-actinNT does not efficiently recognize another nitrated protein and has more than three times affinity for actinNT of unmodified actin. 3 µg of actin, actinNT, BSA and BSANT were loaded in each slot on the nitrocellulose membrane. The membrane was probed overnight with the polyclonal antiserum diluted 17500. Immunoreactivity was normalized to the actual amount of protein loaded on the membrane as detected after Red Ponceau staining.
(TIF)
Figure S2
Characterization of the anti-actinNT antibody. The anti-actinNT antibody was further characterized to determine the specificity of the immunoreactivity against PBMC lysates from controls and ALS patients by Western blotting. Figure S2 shows that the antiserum recognized a band at 42 kDa, which is the expected Mw for actinNT, but also other bands at higher Mw. These are probably polymerized actin forms. These high-Mw species are essentially only detected in the patients and make this antibody especially useful to distinguish ALS patients from controls, also in a dot blot assay, as shown in Figure 1. Equal amounts of PBMC lysates (30 µg) from healthy controls and ALS patients were analyzed. A representative experiment is shown. The PVDF membrane was probed first with the anti-actin antibody (mouse monoclonal, 11000 dilution, Chemicon), and the signal was revealed with a goat anti-mouse Qdot® 800-conjugated secondary antibody (Invitrogen, 1
1000 dilution) on a laser scanner Molecular Imager FX (Bio-Rad), then with the anti-actinNT antibody, and the signal was revealed with an anti-rabbit peroxidase-conjugated secondary antibody/chemiluminescent HRP Substrate (Millipore) on a ChemiDoc XRS system (Bio-Rad).
(TIF)
Figure S3
Analysis of protein biomarkers in dorsal horn spinal cord of the G93A SOD1 transgenic rat. Bars show relative immunoreactivity for the indicated proteins measured by dot blot in ventral horn tissue lysates of presymptomatic (pres, grey bars) (n=
6) and (symp, dark grey bars) G93A SOD1 (n
=
6) rats and non transgenic (control, white bars) (n
=
8) rats. Immunoreactivity was normalized to the actual amount of protein loaded, detected after Red Ponceau staining. None of the protein levels were significantly different from control (one-way ANOVA followed by Newman-Keuls multiple comparison test).
(TIF)
Table S1
Main characteristics of healthy individuals and sALS patients used in the proteomic 2D DIGE analysis.
(DOC)
Table S2
Spot volume changes in 2D DIGE analysis.
(DOC)
Table S3
Candidate protein biomarkers identified by mass spectrometry.
(DOC)
Table S4
Candidate protein biomarkers: 2D DIGE quantitative analysis.
(DOC)
Table S5
Main characteristics of healthy individuals and sALS patients used for the validation analysis ( Figure 2A and Figure 3 ).
(DOC)
Table S6
Univariate logistic regression: controls versus ALS>24.
(DOC)
Table S7
Univariate logistic regression: controls versus ALS≤24.
(DOC)
Table S8
Characteristics of sALS patients and non-ALS neurological controls used for the validation analysis ( Figure 2B ).
(DOC)
Table S9
Characteristics of sALS patients used for the longitudinal study ( Figure 4 ).
(DOC)
Acknowledgments
We are grateful to individuals who participated in the study and to Cesare Perotti at the Transfusion Medical Centre, IRCCS Policlinico San Matteo, Pavia, Italy, and numerous unnamed staff also at the IRCSS Fondazione S. Maugeri, in Pavia and Milano, Italy, and at the NEMO, Niguarda Ca’ Granda Hospital, Milano, Italy, for collecting blood samples. We also thank Donatella Carpi, Roberta Campagna and Renzo Bagnati for assistance in mass spectrometric analysis, Francisco Baralle for the anti-TDP-43 antibody, and Judith Baggott for editorial assistance. We thank the Tolomio and Boldrin families and their friends at Liceo Galilei, Dolo, and Ciba Vision for the donation in memory of Giuseppe Boldrin.
Footnotes
Competing Interests: This work is part of a patent application yet to be published (US Provisional n.61/361,730: Multiprotein biomarkers of amyotrophic lateral sclerosis in peripheral blood mononuclear cells, diagnostic method and kits). This does not alter the authors' adherence to all the PLoS ONE policies on sharing data and materials.
Funding: This work was supported by Telethon Foundation (S01010 and TCR08002 to V.B.), Cariplo Foundation (to V.B. and C.B.), Compagnia San Paolo Foundation (to V.B.), Fondazione Aldo e Cele Daccò per la ricerca scientifica (to V.B.), Vialli and Mauro Foundation for Research and Sport (to S.P. and V.B.), and the European Community's Seventh Framework Programme (FP7/2007–2013) under the Health Cooperation Programme, grant agreement n° 259867 (to V.B. and C.B.). The funders had no role in study design, data collection and analysis, decision to publish, or preparation of the manuscript.
References
Articles from PLOS ONE are provided here courtesy of PLOS
Full text links
Read article at publisher's site: https://doi.org/10.1371/journal.pone.0025545
Read article for free, from open access legal sources, via Unpaywall:
https://journals.plos.org/plosone/article/file?id=10.1371/journal.pone.0025545&type=printable
Citations & impact
Impact metrics
Citations of article over time
Alternative metrics
Article citations
In vivo diagnosis of TDP-43 proteinopathies: in search of biomarkers of clinical use.
Transl Neurodegener, 13(1):29, 03 Jun 2024
Cited by: 0 articles | PMID: 38831349 | PMCID: PMC11149336
Review Free full text in Europe PMC
Identifying dysregulated regions in amyotrophic lateral sclerosis through chromatin accessibility outliers.
HGG Adv, 5(3):100318, 13 Jun 2024
Cited by: 0 articles | PMID: 38872308 | PMCID: PMC11260578
A transient protein folding response targets aggregation in the early phase of TDP-43-mediated neurodegeneration.
Nat Commun, 15(1):1508, 19 Feb 2024
Cited by: 0 articles | PMID: 38374041 | PMCID: PMC10876645
Can Some Anticancer Drugs Be Repurposed to Treat Amyotrophic Lateral Sclerosis? A Brief Narrative Review.
Int J Mol Sci, 25(3):1751, 01 Feb 2024
Cited by: 1 article | PMID: 38339026 | PMCID: PMC10855887
Review Free full text in Europe PMC
Using Redox Proteomics to Gain New Insights into Neurodegenerative Disease and Protein Modification.
Antioxidants (Basel), 13(1):127, 20 Jan 2024
Cited by: 4 articles | PMID: 38275652 | PMCID: PMC10812581
Review Free full text in Europe PMC
Go to all (87) article citations
Data
Data behind the article
This data has been text mined from the article, or deposited into data resources.
BioStudies: supplemental material and supporting data
Genes & Proteins (Showing 43 of 43)
- (1 citation) UniProt - Q9UJV9
- (1 citation) UniProt - P62937
- (1 citation) UniProt - P12814
- (1 citation) UniProt - Q14789
- (1 citation) UniProt - P30041
- (1 citation) UniProt - Q8WU71
- (1 citation) UniProt - P30043
- (1 citation) UniProt - Q06323
- (1 citation) UniProt - P30101
- (1 citation) UniProt - P30740
- (1 citation) UniProt - O00170
- (1 citation) UniProt - P40121
- (1 citation) UniProt - P60174
- (1 citation) UniProt - P07195
- (1 citation) UniProt - Q96AE4
- (1 citation) UniProt - P26038
- (1 citation) UniProt - P07355
- (1 citation) UniProt - P07237
- (1 citation) UniProt - Q9Y490
- (1 citation) UniProt - Q9BZM1
- (1 citation) UniProt - P32119
- (1 citation) UniProt - P60709
- (1 citation) UniProt - P04406
- (1 citation) UniProt - O00299
- (1 citation) UniProt - P37802
- (1 citation) UniProt - Q9NWZ3
- (1 citation) UniProt - P78417
- (1 citation) UniProt - P22627
- (1 citation) UniProt - P18206
- (1 citation) UniProt - P11142
- (1 citation) UniProt - P11021
- (1 citation) UniProt - P52907
- (1 citation) UniProt - P00558
- (1 citation) UniProt - P00338
- (1 citation) UniProt - Q99497
- (1 citation) UniProt - P30040
- (1 citation) UniProt - P04075
- (1 citation) UniProt - P67936
- (1 citation) UniProt - Q96BD8
- (1 citation) UniProt - P27797
- (1 citation) UniProt - P06576
- (1 citation) UniProt - P04179
- (1 citation) UniProt - P21333
Show less
Similar Articles
To arrive at the top five similar articles we use a word-weighted algorithm to compare words from the Title and Abstract of each citation.
Diagnostic and prognostic values of PBMC proteins in amyotrophic lateral sclerosis.
Neurobiol Dis, 139:104815, 20 Feb 2020
Cited by: 12 articles | PMID: 32087285
Tissue-enhanced plasma proteomic analysis for disease stratification in amyotrophic lateral sclerosis.
Mol Neurodegener, 13(1):60, 07 Nov 2018
Cited by: 25 articles | PMID: 30404656 | PMCID: PMC6223075
Biomarkers in Human Peripheral Blood Mononuclear Cells: The State of the Art in Amyotrophic Lateral Sclerosis.
Int J Mol Sci, 23(5):2580, 25 Feb 2022
Cited by: 5 articles | PMID: 35269723 | PMCID: PMC8910056
Review Free full text in Europe PMC
Redox proteomics analysis of oxidatively modified proteins in G93A-SOD1 transgenic mice--a model of familial amyotrophic lateral sclerosis.
Free Radic Biol Med, 39(4):453-462, 14 Apr 2005
Cited by: 87 articles | PMID: 16043017
Funding
Funders who supported this work.
European Commission FP7 (1)
Grant ID: FP7_259867
Telethon (1)
NOVEL BIOMARKERS OF AMYOTROPHIC LATERAL SCLEROSIS: ROLE IN PATHOGENESIS AND POSSIBLE THERAPEUTIC TARGETS
Dott.ssa Valentina Bonetto, Istituto di Ricerche Farmacologiche Mario Negri - IRCCS
Grant ID: TCR08002