Abstract
Free full text

Local Drug Delivery Strategies for Cancer Treatment: Gels, Nanoparticles, Polymeric Films, Rods, and Wafers
1. Introduction
Polymer-based drug delivery systems have been investigated over the last few decades as a means of achieving high therapeutic concentrations of chemotherapy to the site of malignant disease in cancer patients[1-10]. The development of these technologies is guided by the desire to improve overall survival and quality of life by increasing the bioavailability of drug to the site of disease, containing delivery to the cancerous tissues, increasing drug solubility, and minimizing systemic side effects. Existing systems can be divided into two groups based on their mode of administration and mechanism of action. The first relies on systemic delivery and consists of nano-materials such as polymer nanoparticles, liposomes, and dendrimers. These delivery vehicles find their target by localization to solid tumors by passive diffusion via leaky tumor vasculature, active targeting by conjugation to a chemical moiety with an affinity for an over-expressed/unique tumor cell marker (i.e. folic acid receptor, monoclonal antibody, etc), or by triggering the release of payload from an environment-responsive nano-carrier using a local stimulus (i.e. pH, temperature, etc). These nano-materials are predominantly intended for intravenous administration, and, while they promise the ability to target tumor tissues with accumulation of therapeutic concentrations of drug, localization is challenging due to removal and sequestration of these nanomaterials by the reticuloendothelial system. Additionally, there is a recognized need for development and validation of nano-toxicity characterization methods for obtaining reliable predictive safety information [11].
The second group of polymer delivery vehicles (and focus of this review) includes controlled release drug delivery depot systems for implantation intra-tumorally or adjacent to the cancerous tissue (Figure 1). These technologies have been embodied in a variety of form-factors such as drug-eluting films, gels, wafers, rods, and particles and feature predictable and prolonged drug release kinetics. The majority of these devices are biodegradable so as to circumvent a second surgery for device removal and to avoid a chronic foreign-body immune response. The polymers used in these systems can be broadly divided in to natural and synthetic materials. Natural polymers that have been investigated for drug delivery applications include polysaccharides such as alginate[12-14], hyaluronic acid[15], dextran[16] and chitosan[17-19] and polypeptides including collagen[20], albumin[21, 22], elastin[23], and gelatin[24, 25]. These materials are tolerated well in vivo, are available in abundance in nature, and can form hydrogels via self-assembly or by cross-linking. Furthermore, the property of spontaneous hydrogel formation of some natural polymers has been exploited to develop smart delivery vehicles that can be injected locally as a liquid, and upon exposure to changes in environment such as temperature, pH, or ionic composition, solidify into a hydrogel drug depot. Drawbacks of these materials include: 1) a necessity for high purity for biocompatibility, 2) poor solubility, particularly in organic solvents, restricting processing options and complicating the inclusion of water-insoluble chemotherapy agents, and 3) limited opportunity for chemically tuning polymer compositions to affect key properties such as drug release kinetics and degradation rate.
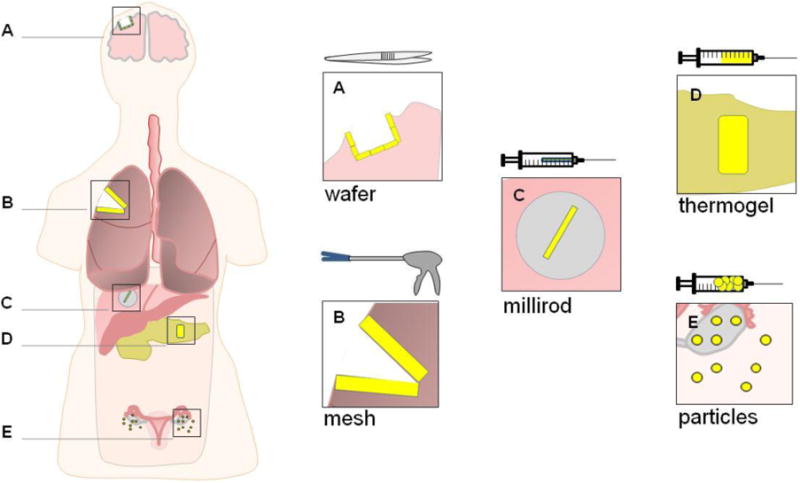
Examples of localized chemotherapy delivery form factors at various treatment sites and their respective modes of administration.
Conversely, the degree of customization achievable with synthetic polymers allows the application-specific design of local implants with respect to degradation, drug release, and mechanical properties. A wide range of delivery materials have been fabricated using polyesters based on lactide, glycolide, caprolactone, and dioxanone, polyanhydrides based on sebacic and adipic acid, as well as polyamides, polycarbonates, polyorthoesters, and phosphate-based polymers, which have been reviewed in detail elsewhere[7, 9, 10, 26-28]. These polymers are often hydrophobic in nature, and are ideally suited for long-term delivery and internal stabilization of sensitive water-insoluble drugs. A significant drawback to synthetic materials is that many form acidic degradation products that can accumulate and cause inflammation at the implant site. However, this effect can be mitigated via adjustments in chemical composition and degradation profile. The aims of this article are to review the most well-studied and efficacious local polymer delivery systems from the last two decades, to examine the rationale for utilizing drug-eluting polymer implants in cancer patients, and to identify the patient cohorts that could most benefit from localized therapy.
2. The Clinical Need for Localized Cancer Therapy
The lifetime probability of developing an invasive cancer is 44% for men and 38% for women resulting in an estimated 1,529,560 cancer diagnoses and 569,490 deaths in the US in 2010 [29]. Treatment is dictated by the cancer type, stage at diagnosis, and the patient's tolerance to the prescribed therapy. Tumors are normally classified by the TNM staging system (tumor, node, distant metastasis) which describes the extent to which the cancer has spread. Staging can be broadly divided into early, intermediate, or late stage cancers. Early stage tumors are localized to an anatomical site without evidence of spreading, intermediate cancers may include larger tumor masses and/or evidence of lymph node involvement, and late stage cancers have metastasized from their primary tissue site to other regions of the body. As evident in Table 1, for most, the majority of patients' cancers are diagnosed at local or regional stages, with 5-year survival rates varying dramatically depending on tumor type and stage. For example, locoregional prostate cancers are almost always curable, while locoregional lung cancers are significantly more difficult to treat effectively. Even those cancers that boast high 5-year survival statistics could benefit from improved control of localized disease by limiting the extent of surgical resection, circumventing radiation therapy, and avoiding various treatment toxicities, thereby maximizing preservation of functioning tissue. While the incidence of cancer mortality has steadily decreased over the last several decades due to improvements in early detection and advancements in technology, there are still major shortcomings in treatment-associated morbidity, recurrence rates, and other outcome measures with current standard of care approaches for most cancers. There are potential intervention points at each stage of cancer where localized therapy, either for curative or palliative intent, could supplement or replace existing treatments.
Table 1
Cancer statistics (2010) for cancer types under investigation for localized chemotherapy interventions [2]. The requirements of localized drug delivery vary depending upon cancer type and stage at diagnosis. Surgically operable early stage tumors are often associated with a high risk of recurrence (i.e. lung, colon), while late stage inoperable tumors may benefit from localized palliative chemotherapy. A fraction of patients cannot be properly staged at diagnosis, thus percentages do not always add to 100%.
Total Cases | Stage at Diagnosis | 5-Year Survival | ||||||
---|---|---|---|---|---|---|---|---|
Diagnoses | Deaths | Localized | Regional | Distant | Localized | Regional | Distant | |
Lung and Bronchus | 222,520 | 157,300 | 15% | 22% | 55% | 53% | 24% | 4% |
Female Breast | 209,060 | 40,230 | 60% | 33% | 5% | 98% | 84% | 23% |
Prostate | 217,730 | 32,050 | 80% | 12% | 4% | 100% | 100% | 31% |
Colon | 102,900 | 51,370 | 39% | 37% | 19% | 91% | 70% | 11% |
Rectum | 39,670 | |||||||
Urinary Bladder | 70,530 | 14,680 | 56% | 8% | 4% | 74% | 36% | 6% |
Uterine Corpus | 43,470 | 7,950 | 69% | 19% | 8% | 96% | 67% | 17% |
Uterine Cervix | 12,200 | 4,210 | 50% | 35% | 11% | 92% | 58% | 17% |
Surgical resection of the primary tumor and/or adjacent lymph nodes is the preferred treatment for most early stage (localized) or intermediate stage solid tumors with locoregional lymphatic involvement. The partial resection or debulking of late stage or diffuse multifocal tumors can, in some cases, have a palliative effect and improve the quality of life for some patients [30, 31]. Depending on the tumor location, the benefit of removing cancerous tissue must be balanced against the resulting morbidity to the patient. Unfortunately, undetected occult microscopic disease can remain despite a complete surgical resection, and thus concurrent treatment with radiation and/or chemotherapy is often utilized with more aggressive cancer types, in an attempt to prevent recurrent tumor growth. For example, reported locoregional recurrence rates following ‘curative’ surgery have remained unacceptably high for some cancer types including lung (27%) [32] and colon (11%) [33]. For this reason, neoadjuvant chemotherapy and/or external beam radiation therapy are sometimes used to shrink particularly large tumors and/or ‘control’ regional disease prior to surgical excision, effectively ‘down-staging’ the disease before surgery in some patients. Radiation treatment, although generally associated with lower 5-year survival than surgery, can be curative for early stage cancers and is utilized as an alternative to resection in late stage patients including prostate, breast, and lung cancers or those unable to tolerate surgery. Unfortunately, the acute and long-term toxicities and limited efficacy associated with these adjuvant therapies have prevented their routine use as prophylactic interventions in all patients.
Chemotherapy is reserved primarily for intermediate and late stage cancers and can be administered before or after surgical resection, or with or without radiation, in lieu of surgery when the tumor is unresectable. Many chemotherapeutic drugs suffer from prohibitively low aqueous solubility which prevents intravenous administration unless they are chemically modified as a soluble pro-drug (e.g. Irinotecan) or formulated in a surfactant-containing solution such as Cremophor/ethanol as in the case of paclitaxel. However both strategies can lead to low bioavailability, sensitization reactions, and other secondary side effects [34, 35]. Given that intravenous systemic chemotherapy is not specifically targeted to the tumor, it is very difficult to achieve therapeutic levels of drug within or adjacent to the tumor. Furthermore, significant concentrations of drug frequently accumulate in healthy tissue, leading to severe side effects and dose-limiting toxicity. For example, almost half the dose of an intravenous injection of paclitaxel is eliminated during the first 24 hours, with less than 0.5% of the total dose locally available to treat tumor within the lung [36]. The use of radiation and chemotherapy also significantly adds to the cost of cancer care due to the high material costs and the need for appropriate specialists including radiation and medical oncologists and a robust support staff for delivery of therapy, appropriate monitoring, and treatment of side effects. These treatments are time consuming for both patients and personnel, requiring frequent visits over months of therapy.
Brachytherapy, the implantation of point-source radiation seeds intra-tumorally, adjacent to tumor, or at the surgical resection margins, has proven to be one efficacious localized therapy option for treatment of inoperable tumors and for prevention of local tumor recurrence. It is routinely administered for slow-growing prostate tumors as an alternative to surgery and has shown utility in several other cancers including lung, cervical, and breast malignancies [37-39]. For example, brachytherapy seeds applied at the site of surgical resection have been shown to reduce the incidence of local recurrence in lung cancer patients after wedge resection from 19% to 2% [40]. Furthermore, it was demonstrated that adding brachytherapy to a lobectomy performed for 2-3 cm lung cancer tumors significantly decreased recurrence rates and increased survival in patients from 44.7 months to 70 months (p=0.003), demonstrating that prevention of local recurrence via localized therapy can significantly improve survival in such patient populations [41]. Given that incremental increases in survival by a few months are considered exceptional for novel chemotherapy drugs, the increase in survival by over two years with local brachytherapy is remarkable [42]. Though localized brachytherapy has demonstrated significant benefits to disease-free progression and survival in patients with localized tumors following resection, global adoption has been resisted due to its cumbersome method of administration, by-stander tissue effects, issues of radiation handling and toxicity, and limited availability [40, 43-45].
Treatment failure in early and intermediate stage cancers, including high locoregional recurrence rates, is indicative of the shortcomings seen with the current standard of care for some malignancies. The potential benefits of localized chemotherapy at the tumor site are numerous and are intended to both enhance the efficacy of treatment and reduce patient morbidity. Drug-loaded implants are administered directly at the site of disease, offering the following advantages over traditional systemic delivery: 1) stabilization of embedded drug molecules and preservation of anticancer activity, 2) controlled and prolonged drug release to ensure adequate diffusion and uptake into cancer cells over many cycles of tumor cell division, 3) loading and release of water-insoluble chemotherapeutics, 4) direct delivery to the site of disease, resulting in less waste of drug, 5) one-time administration of the drug, and 6) diminished side effects due to the avoidance of systemic circulation of chemotherapeutic drugs. Furthermore, prolonged exposure of tumor cells to chemotherapy over multiple cell cycles has been shown to be more cytotoxic than bolus delivery for most drugs that target pathways involved in cell replication. Only 10 to 15% of tumor cells are expected to be in the mitotic phase of cell division at any time, thus limiting the sensitivity of these cells to anti-neoplastic chemotherapy agents over short term exposures [46-48]. Use of a long-term drug eluting implant promises to be beneficial when a) the incidence of local recurrence does not warrant universal treatment of all patients with a highly morbid systemic therapy, b) local disease is inoperable or untreatable through traditional means in late stage disease, or c) local tumor control offers the opportunity to perform a less invasive surgical procedure or provides palliative relief. As such various types of synthetic and natural-based polymers have been evaluated as controlled release depots for cancer therapy including poly(ethylene-co-vinyl acetate) [49], poly(caprolactone) [50], poly(methyl methacrylate) [51], segmented polyurethane [52], poly(lactide-co-glycolide) [53], chitosan [54, 55], fibrin [56], gelatin [57], and collagen [20] (Figures 2, ,3).3). This review will survey polymer implant technologies investigated for local delivery of anticancer agents ranging from promising novel devices with notable preclinical successes to established, commercialized therapies that have undergone human clinical trials.
3. Gliadel Wafer
The Gliadel Wafer (MGI Pharma/Easai Pharmaceuticals) is perhaps the most-well studied and successful drug delivery implant for the treatment of recurrent brain cancer. Developed in the early 1980's by Langer and Brem, this technology has been reviewed from its chemistry and mechanistic aspects of drug release to its performance across multiple clinical trials [58-60]. The highlights of this technology will be outlined briefly to allow comparison to other technologies in this review.
The Gliadel Wafer is a dime-sized, biodegradable polyanhydride wafer containing the chemotherapeutic carmustine and is used for the treatment of high grade malignant glioma, an aggressive brain cancer. Up to eight wafers are deposited along the resection cavity following surgical excision of the primary brain tumor (Figure 4). It should be noted that in many cases these types of glioma tumors cannot be completely removed, thus the surgeon will “debulk” as much tumor as possible while concurrently using adjuvant treatments like chemotherapy or radiation. In addition to the conventional benefits offered by local drug delivery, delivery of drug from the Gliadel Wafers directly to brain tissue bypasses the problem of delivering systemic chemotherapy across the blood-brain barrier. The polymer matrix is comprised of a copolymer of 1,3-bis-(p-carboxyphenoxy) propane and sebacic acid (PCPP-SA; 80:20 molar ratio) that is dissolved in an organic solvent with carmustine, spraydried into microparticles ranging from 1-20 μm, and compression molded into wafers (14 mm diameter, 1 mm thick). The rigid wafers degrade in a two-step process wherein water penetration hydrolyzes the anyhydride bonds during the first 10 hours followed by erosion of the copolymer into the surrounding aqueous environment. In a preclinical study, the wafers were shown to release carmustine in rat brains over a period of approximately five days followed by complete degradation of the polymer matrix at 6-8 weeks after implantation [61]. Carboxyphenoxypropane is eliminated by the kidney while sebacic acid is metabolized by the liver. It was discovered during a clinical trial that wafer remnants were present in human patients for up to 232 days following implantation. According to the clinical studies described in the prescribing information for Gliadel Wafers, treatment with the wafers resulted in significant increases in survival time for both primary and recurrent disease. Patients with newly-diagnosed, high grade malignant glioma (n = 240) demonstrated an increased survival from 11.6 months (placebo) to 13.8 months with Gliadel wafers together with surgical resection and, in most cases, radiation. Additionally, median survival increased for patients treated after surgery for recurrent disease (n = 222) from 5.5 months (placebo) to 7.4 months [62], though a systematic review concluded no significant added benefit between the treatment groups [63].
4. Paclimer Microspheres
Paclimer microspheres are a microparticle technology intended for intraperitoneal administration and ultimately prevention of recurrent ovarian cancer. Developed in part by Guilford Pharmaceuticals, the use of Paclimer has demonstrated modest success in vivo leading to a Phase 1 clinical trial. Paclimer microparticles consist of a sustained-release formulation of paclitaxel-loaded (10% wt/wt) polyphosphoester particles. Harper et. al. reported the first formulation and in vivo assessment of Paclimer microspheres against non-small cell lung cancer by intratumoral injection[64]. The microspheres (average diameter: 53 μm, range: 20 to 200 μm) were shown to release paclitaxel at a rate of approximately 1-2%/day continuously over 90 days (Figure 5). In vivo efficacy was evaluated against two non-small cell lung cancer cell lines (A549 and H1229), which were injected subcutaneously in mice and grown to yield small tumor nodules (200 – 300 mm3), followed by intratumoral (i.t.) or intraperitoneal (i.p.) treatment injection. The highest and most effective dose (24 mg/kg) was assessed against conventional i.t. and i.p. formulations. When given i.t., the paclimer delivery system decreased the tumor volume doubling time nearly six-fold against A549 tumor (60 ± 9.4 days) compared to i.p. (11.5 ± 2.3 days) and i.t. controls (10.2 ± 4.7 days); furthermore, it decreased the doubling rate approximately three-fold against H1229 tumor (35 ± 8 days) compared to i.p. (12 ± 1.9 days) and i.t. (11.2 ± 1.9 days).
In a later study, the Paclimer microparticles were investigated as a potential localized treatment for malignant glioma in the brain, in a manner analogous to the Gliadel wafer [65]. The microparticles were mixed with PEG-1000 and compressed into discs (1 mm depth, 3 mm diameter) to facilitate administration to the brain; PEG-1000 is solid at room temperature but liquid at 37°C, thus the disc is designed to melt after implantation and quickly release the microparticles locally. The biocompatibility of the delivery system was assessed via implantation in the cerebral hemispheres of adult rats and compared against the Gliadel polymer (PCPP-SA). The severity of the invoked immune response elicited by the Paclimer material was equal or less than PCPP-SA over 1, 2, 4, and 12 weeks after implantation. Notably, the addition of paclitaxel to the particles did not result in additional toxicity. In contrast, paclitaxel-loaded PCPP-SA was shown to produce significant adverse effects in rats and led to death in 25% of animals. This disparity in toxicity was attributed to the release kinetics of paclitaxel from the two implants. PCPP-SA releases a burst of drug of up to 50% within 24 hrs of implantation, whereas the polymer used in Paclimer microspheres does not exhibit large burst release kinetics, but rather approximates zero order release kinetics. Therapeutic levels of paclitaxel were measured within 5-7 mm of the implant 30 days after treatment. Paclimer increased the median survival time of rats with established 9L glioma tumors from 16 to 35 days. In comparison, systemically delivered paclitaxel produced no survival advantage.
Pradilla et. al. demonstrated acceptable safety and toxicity of Paclimer (2 and 20 mg/kg) for single dose local intracerebral administration in a canine model [66]. No systemic toxicity was observed for either dose over the 120 day experiment. There were no neurological complications resulting from the lower dose (0/5 animals), and just one complication from the higher dose (1/5) attributed to accidental intraventricular administration. One animal in each group developed suture dehiscence, likely caused by the subcutaneous presence of paclitaxel, and three animals developed wound infections; all these animals were successfully treated with antibiotic therapy. Histological examination showed a chronic inflammatory reaction and superficial gliosis localized to the parenchyma surrounding the implant location.
Given the radiosensitizing properties of paclitaxel, the efficacy of Paclimer treatment via intratumoral injection of microparticles in conjunction with radiation treatment was investigated by Lapidus et. al. in a mouse xenograft model of prostate cancer [67]. Combination therapy with Paclimer microspheres and one acute dose of radiation (10 Gy) led to an improved response (td = 13.4 ± 1.0 days, 7/10 survived) compared to those treated with a placebo (td = 5.7 ± 0.4 days, 2/10 survived), Paclimer alone (td = 6.7 ± 0.7 days, 3/10 survived), or radiation with placebo (td = 6.8+0.4 days, 3/10 survived).
More recently, a phase I trial by the Gynecological Study Group investigated intraperitoneal administration of Paclimer microspheres in 12 patients with recurrent ovarian cancer [68]. Dose-limiting toxicity was not observed in patients receiving up to 1200 mg/m2 paclitaxel from i.p.-administered Paclimer, compared to the dose-limiting toxicity of 60 – 125 mg/m2 observed in several other clinical trials using i.p. paclitaxel. One patient did undergo surgical re-exploration, where a significant inflammatory response was noted, including extensive adhesions, fat necrosis, fibrous connective tissue, and foreign body giant cell reaction. In addition, residual polymer filaments were found at the time of re-exploration seven months after concluding treatment, indicating that the Paclimer polymer degrades slowly. This study was halted due to the manufacturer's decision to suspend further clinical development of the Paclimer microspheres, but the reasons for this decision have not been made public.
5. Expansile Nanoparticles
Expansile nanoparticles have been designed to release their drug payload upon exposure to an environmental trigger, thus focusing the delivery of drug at the treatment site and minimizing systemic exposure. Their utility has been demonstrated successfully in two tumor models: lung cancer[69], and mesothelioma[70][71].
Griset et. al. reported the use of a novel nanoparticle polymer composition (100 nm diameter) for the prevention of lung tumor growth [69]. Upon exposure to a pH of 5 or lower, such as would be encountered in the endosome following internalization of the particle into the cell, acid-labile hydrophobic protecting groups on the polymer are cleaved, causing the nanoparticle to swell dramatically and release its payload within 24 hours (Figure 6). The particles were evaluated in vivo using a murine tumor establishment model where Lewis lung carcinoma cells were co-injected subcutaneously with either paclitaxel-loaded expansile nanoparticles (pax-eNP; 2 or 20 μg pax), paclitaxel-loaded non-expansile nanoparticles of a similar polymer composition (pax-neNP; 2μg pax), empty expansile nanoparticles (eNP), or paclitaxel alone (pax; 20 μg). Tumor developed rapidly for each control group, with tumor establishment present in the vast majority of unloaded eNP (12 of 15 mice), pax (6/6), and pax-neNP (7/7) treated mice within fourteen days following injection. Conversely, low-dose expansile particles (2 μg) prevented tumor establishment in all but one animal (1/14) at a dose 100-fold less than the reported therapeutic dose of paclitaxel, and high-dose pax-eNP (20 μg) completely prevented tumor establishment throughout the two week study (0/8). In a subsequent study, the paclitaxel-loaded expansile particles were evaluated in a murine recurrence model in which an LLC primary tumor was established subcutaneously (300 mm3) followed by complete surgical resection[73]. After surgery, the mice were treated with intravenous paclitaxel (pax-IV; 300 μg), or a subcutaneous injection of pax-eNP (300 μg), empty eNP, or saline control. A modest but statistically significant delay in median local recurrence time was observed for pax-eNP compared to controls (10 vs. 6 days) with an incremental survival increase in overall survival (16 vs. 11-12 days). It was noted that these results highlight both the benefit of localized delivery and the limitations of the short-term (<24 hr) delivery of anti-neoplastic drugs to adequately eliminate dividing cancer cells.
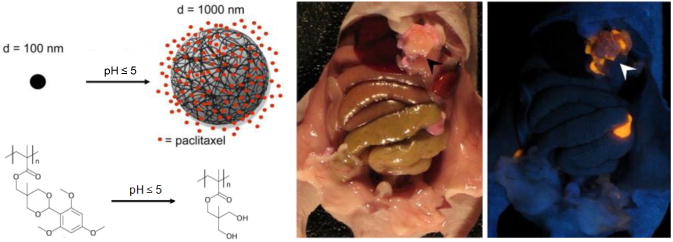
(left) Expansile nanoparticles swell upon exposure to a mildly acidic pH, releasing their payload intracellularly. (right) The nanoparticles localize to tumors following intraperitoneal injection (Ambient image on left and corresponding UV image on right were taken following injection) (adapted from Colson et. al.[71])
Expanding upon this work, paclitaxel-loaded expansile nanoparticles were evaluated in a murine model of peritoneal carcinomatosis, a disease characterized by the occurrence of diffuse tumor in the abdominal region [71]. Cancers of this type are caused by malignancies such as mesothelioma, appendiceal carcinoma, and ovarian metastases resulting in 5-year survival rates of less than 40% and recurrence rates as high as 80% following surgical debulking, even with the use of intraperitoneal taxane chemotherapy [74-76]. Specifically, mesothelioma (MSTO-211) cells were co-injected intraperitoneally with empty eNP, pax-neNP, pax-eNP, saline, or paclitaxel (pax-ip). All paclitaxel dosages were 20 μg/per treatment. The animals were sacrificed 35 days following injection and analyzed for tumor burden, and assigned disease severity scores (DSS; scores range from mild (1-5) to severe (15-20)). The empty eNPs, PBS, and pax-ip control groups were ineffective at preventing tumor growth, with DSS scores of 12, 14, and 15.5 and total tumor burden of 1.52 ± 0.2g, 2.32 ± 0.41g, and 2.14 ± 0.04g respectively. While both the expansile and non-expansile particles significantly reduced the severity of disease (DDS of 3 and 5, respectively), tumor burden was almost un-detectable in animals treated with pax-eNP (0.05 ± 0.02 g). The pax-neNP-treated group demonstrated a nearly 10 fold greater tumor burden (0.4 ± 0.2 g) than with Pax-eNP. The improvement in efficacy of pax-eNP compared to pax-ip has been hypothesized to result from the localization of expansile nanoparticles to the tumors. Localization was confirmed using expansile nanoparticles conjugated to rhodamine dye. Tumor was established in mice seven days prior to intraperitoneal injection of the fluorescent nanoparticles. Co-localization was confirmed by fluorescence and UV microscopy (Figure 6). Additional studies demonstrated a survival advantage for a single treatment of pax-eNP over pax-neNP or pax-ip with median survival times of 54, 39, and 26 days, respectively.
Lastly, it has also been shown that expansile nanoparticles are able to travel through the lymphatic system in a large animal over long distances (40 cm) to reach a sentinel lymph node following subcutaneous injection[72]. This may have implications for preventing lymph node metastasis, and efficacy studies need to be performed following this proof-of-principle demonstration. Additionally, since the particles retain their payload until triggered by cell encapsulation, eNP delivery may facilitate drug penetration in solid tissues by allowing time for diffusion before releasing the drug downstream of the delivery site.
6. Chitosan Hydrogels
Chitosan is a linear cationic polysaccharide derived from the shells of crustaceans (e.g. crabs and shrimp) that has found various uses in biomedical applications due to its reported biodegradation and biocompatibility [77]. Chitosan is produced by the deactylation of chitin, and has been used clinically in applications such as suture and wound healing materials [78]. The material has been investigated as a drug delivery system in the form of microparticles, nanoparticles, hydrogels, and films for cancer treatment. Two recent chitosan-based gels have been developed to deliver chemotherapy or radiation.
Early studies have shown that the gelation temperature of aqueous chitosan solutions is dependent, in part, on the degree of acetylation present in the polymer. Glycerol-phosphate salt can be added to the chitosan solution to help modulate electrostatic, hydrophobic, and hydrogen bonding interactions, culminating in the displacement of water upon heating followed by the gelation of chitosan molecules [79]. Biosyntech Inc. has optimized this technology (BST-Gel) and several such formulations have been assessed in mouse tumor models delivered via intratumoral injections (Figure 7). In a study by Ruel-Gariepy et. al., a thermogelling solution composed of chitosan, β-glycerophosphate salt and paclitaxel (0.64 or 6.4% w/v) was evaluated in vitro and in vivo for eventual use along tumor resection margins to prevent local tumor recurrence [80]. In vitro release of paclitaxel from the gels was continuous for 30 days, with a somewhat unexpected two-fold slower release (2.0 vs 4.2% over days 2 to 10) and smaller initial burst (7.0 vs 16.6%) for the higher loading compared to the lower dose . Next, the drug-loaded gels were assessed in vivo using mice with subcutaneous EMT-6 mammary carcinoma tumors (30 mm3) where it was demonstrated that paclitaxel-chitosan intratumoral injections were as effective at inhibiting tumor growth as the intravenous paclitaxel positive control (10 mg/kg/day × 4 days). Furthermore, 38-40% growth inhibition was observed with both treatment groups when compared to saline-treated mice over 17 days. A second model was investigated where the mice were treated four days after inoculation to simulate remaining malignant cells after surgical resection. Approximately 70% growth inhibition was observed for both high- and low-dose paclitaxel treatment groups compared to the negative control after 17 days. Those mice treated with intravenous paclitaxel displayed weight loss over the first week of treatment in contrast to those treated locally with the loaded gels. However, tumors treated with the chitosan formulation did exhibit inflammatory cell infiltration and hyperemia compared to the intravenous control.
A similar study evaluated the same gel technology used as a delivery vehicle for camptothecin [81]. Drugs from the camptothecin family are known to cause significant adverse reactions in patients following systemic administration, partly due to their rapid conversion from the active lactone form to the inactive and toxic carboxylate form in human serum (e.g. mean t1/2 = 9.5 min for Irinotecan [82]). Local delivery of camptothecins promises concentrated levels of drug at the site of disease with reduced systemic circulation. Camptothecin (4.5% w/w) was added to a thermo-gelling formulation of chitosan and β-glycerophosphate. Release of camptothecin was nearly linear (2-3%/day) with a cumulative release of greater than 80% over 30 days without a significant initial burst. When assessed in mice bearing RIF-1 tumors (100 mm3), significant tumor growth delay (determined at 500 mm3) was observed for animals treated with an intratumoral injection of the camptothecin gel (25.0 ± 2.7 days) compared to the control i.p. injection (7.7 ± 1.3 days), blank gel (6.8 ± 1.1 days) or untreated animals (6.5 ± 0.9 days). No observable signs of toxicity were seen for the loaded gels, as assessed by weight loss or topical irritation.
In addition to local delivery of chemotherapy, chitosan-based delivery of radioactive substances has been actively investigated as an alternative method of controlling localized or recurrent tumor growth. One such strategy utilized slow and fast-degrading chitosan hydrogels incorporated with the radioactive substance 131I-norcholesterol (131I-NC) [83]. Following subcutaneous or intraperitoneal implantation in rats, the slow-degrading gels remained relatively stable over 28 days whereas the fast-degrading gels degraded almost completely over 14 days. Histology revealed a fibrous capsule approximately 80-100 μm thick around both fast and slow-degrading implants at 14 and 28 days without other evidence of acute or chronic inflammation. The slow-degrading gel implanted in the left pectoral tissue showed that 131I-NC retained approximately 20% of its initial activity over 30 days, and, 4% activity was detected in the axillar lymph nodes at days 4 and 13. In a separate study, it was shown that the foreign body response to the unloaded gels was milder than that invoked by Vicril absorbable sutures [84]. Gel degradation products were not found in distant organs following implantation. Tissue damage and the severe fibrotic response elicited by the 131I-NC-loaded gels were evident only within several microns of tissue adjacent to the implant.
Azab et. al. investigated the inhibitory effect of 131I-NC-loaded chitosan gels against models of primary and locoregional recurrent tumor growth [44]. Gluteraldehydye-crosslinked chitosan gels were loaded with 131I-Norcholesterol to produce a dose similar to that used in a standard intravenous injection for breast cancer treatment. For the primary therapy model, mice were inoculated subcutaneously with 4T1 mouse mammary tumor cells on the back, and, following two weeks of tumor establishment, the gels were implanted at the tumor site. Initial tumor progression over the first 14 days was 5-fold slower for the treatment group (0.02 g/day) and survival was increased by one week compared to the untreated and control groups (0.11 g/day). However, all three groups reached similar tumor sizes at 28 days. Metastatic spread to the lungs was evident in the control groups after 14 days, whereas no such spread was detected in the treatment group. Next, an adjuvant therapy model was designed to simulate recurrent tumor growth through tumor inoculation at the time of gel implantation. For this model, untreated and blank gel groups showed survival of 77 to 84 days following tumor inoculation. In contrast, 69% of the animals in the chitosan gel treated group were tumor-free over the 160 day experiment. Again, metastatic spread was only evident in the control groups. The loaded gels were determined to have a biological elimination half-life of 14 days.
7. Oncogel
Paclitaxel formulations of a poly(lactide-co-glycolide) and poly(ethylene glycol) tri-block copolymer(PLGA-PEG-PLGA), also known as ReGel and manufactured under the trade name OncoGel, have been assessed in vivo to evaluate efficacy in local tumor management [85]. The ReGel system is a thermosensitive, water-soluble implant comprised of an aqueous solution of biocompatible polymers. Similar to the BST-gel chitosan technologies described earlier, ReGel solutions are designed to undergo a reversible thermal gelation upon injection in vivo. OncoGel demonstrates controlled release of paclitaxel over 50 days with complete degradation observed over 6-8 weeks in vitro (Figure 8). Solubility enhancement of paclitaxel was greater than 400-fold (> 10 mg/mL). Following intratumoral injection of OncoGel into mice bearing human breast carcinoma xenografts, less than 0.1% of radioactively-labeled taxol was measured in the other organs or blood combined over the study. Animals treated with Oncogel responded at a 10-fold lower dosing with similar efficacy compared to those treated with the maximum-tolerated systemic dose, and they exhibited no drug-related adverse effects.
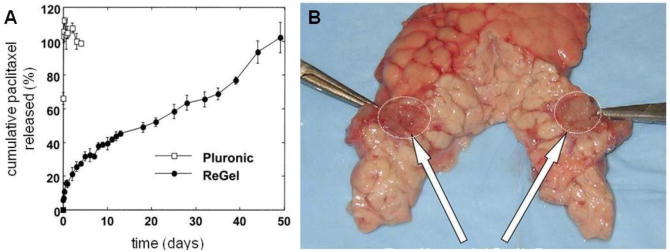
Oncogel PLGA-PEG-PLGA paclitaxel delivery system. A) cumulative in vitro release of paclitaxel from Oncogel, compared to release from Pluronic, an ethylene glycol/propylene glycol triblock copolymer, and B) injected pancreatic tail w/ arrows identifying Oncogel depot. (adapted from (A) Zentner et. al[85].; (B) Matthes et. al.[86])
Localized chemotherapy may also provide palliative therapy through local tumor control in aggressive unresectable malignancies such as pancreatic carcinoma [86]. OncoGel injections were guided by endoscopic ultrasound for localized administration to the pancreas in a porcine model. The objective of this study was to determine if a minimally-invasive injection of the gel could produce sustained therapeutic drug concentrations to the pancreas. Injected OncoGel delivery depots (14.7 ± 0.5 mm in diameter) were able to deliver therapeutic concentrations of paclitaxel 30 – 50 mm from the depot in the pancreas. Observed localized tissue reactions included mild chronic inflammation, fat necrosis and atrophy; animals showed localized fibrotic tissues 14 days after the initial injection. Additionally, the animals tolerated the injection procedure without developing pancreatitis or intra-abdominal infection.
Symptomatic spinal metastases, which affect 5% of the 1.2 million diagnosed cancer patients a year, could also benefit from local palliative therapy. Seen most often in lung and breast cancer patients, treatment of symptomatic lesions traditionally involves radiation and/or surgery to maintain ambulatory status. Reports of effective chemotherapy treatment are rare due to the nature of the disease. OncoGel injections were evaluated in a breast adenocarcinoma metastatic spine tumor rat model as a means to reduce symptoms. Complete hind limb paresis was significantly delayed following intratumoral injection of OncoGel within the L-6 vertebral body, with paralysis occurring at approximately 16 days for blank gels, 19 days for low dose gels (3% paclitaxel), and 24 days for high dose gels (6% paclitaxel). Median survival increased an average of 4 days for both OncoGel doses (18 days) compared to the control (14 days).
Lastly, a Phase I clinical trial was performed on patients with inoperable solid tumors in order to characterize toxicity, pharmacokinetics, and antitumor activity of OncoGel injected directly into tumors accessible by percutaneous injection [87]. A total of 16 patients received OncoGel injections with paclitaxel dose levels ranging from 0.06 to 2.0 mg/cm3 tumor volumes with injection volumes averaging 21% of total tumor volume. Only one dose-limiting toxicity event occurred for a patient who reported grade 3 injection site pain, and this event was quickly resolved with a local injection of an analgesic. Twelve adverse reactions were observed during the study and attributed to local administration of OncoGel including injection site pain (5), injection site or tumor site erythema (4), injection site bruising (1), post-procedural discharge (1), and muscle spasm (1). Changes in tumor volumes ranged from 56.3% reduction to 232.5% increase in size. Systemic paclitaxel concentrations were minimal with only 3.3% of samples analyzed containing quantifiable level of paclitaxel. The highest dose evaluated in this study (2.0 mg/cm3 tumor volume) did not produce a dose-limiting response.
8. Polymer Millirods
The use of chemotherapyloaded polymer millirod implants to supplement the treatment of tumors with radio-frequency (RF) ablation in an effort to reduce local recurrence rates has also been actively studied. Tumor recurrence is greatest at the periphery of the ablation zone and around blood vessels where residual tumor cells remain. It is hypothesized that drug eluted from an implant placed at the center of an ablation region will undergo enhanced tissue penetration, in part due to destroyed tumor vasculature in the ablated region [88]. The Gao group developed drug-loaded poly(lactide-co-glycolide) polymer millirods to prevent recurrence in thermoablated tissues through image-guided delivery via a 14 gauge biopsy needle [89]. The release kinetics of doxorubicin from these millirods were quantified after implantation in thermoablated rat livers [90]. Following in vivo implantation of doxorubicin-loaded millirods (16 w/w %) into rat livers, half of the doxorubicin was released into non-ablated and ablated (~4 mm radius) livers over 3.5 ± 1.5 hr and 6.5 ± 2.5 hr, respectively. The non-ablated livers achieved therapeutic concentrations of drug 1.1 ± 0.1 mm from the implant-tissue interface over 24 hr and decreased thereafter. In contrast, doxorubicin penetrated 4.1 ± 0.2 mm by 7 hr in livers of the ablated animals with the concentration of drug remaining relatively constant in this region for up to 96 hr. A subsequent study assessed the anti-tumor activity of doxorubicin-loaded millirods in an in vivo liver carcinoma model [91]. Spherical liver carcinoma xenograft tumors (~4 mm radius) were implanted in healthy rabbits and grown for 12 days, before introducing one millirod into each tumor through a pre-punctured opening. In vivo drug release revealed 87% of loaded drug was released by day 4 with a penetration distance of 2.8 ± 0.5 mm and a tissue elimination half-life of 1.6 ± 0.2 days (Figure 9). Tumor size was evaluated by visible cross-sectional area in excised livers, and it was demonstrated that treated tumors (0.14 ± 0.04 cm2) were significantly smaller than untreated controls (1.8 ± 0.8 cm2) after 8 days. It was noted that viable tumor cells were found outside the treated area of the tumor, suggesting that these millirods would benefit from a combined modality treatment. This hypothesis was evaluated in another study where it was found that the fibrous capsule that typically forms around the ablated tissue during the normal healing response seemed to act as a transport barrier to drug penetration from an implant placed within the ablated region to the surrounding healthy tissue adjacent to the region [92]. Therapeutic levels of doxorubicin released from PLGA millirods inside liver tumors (11 mm in diameter) in rabbits were found within 81% of the ablated tissue (8 mm in diameter) after 4 days, and 40% of the tissue after 8 days. After 8 days, 1 of 4 control livers had viable tumors and 0 of 3 treated livers had viable tumors. Doxorubicin penetrated 3.7 ± 1.3 mm into the tissue from the implant by day 4 and was detected 2.1 ± 0.3 mm from the implant boundary by day 8. Unfortunately, tumors recurred around the ablation boundary, likely a consequence of only limited amounts of drug penetrating past the fibrous capsule and traveling outside of the ablation zone. Blanco et. al. reported a potential solution to the delivery of therapeutic levels of drug outside ablated tissue by delivering the potent anti-inflammatory dexamethasone (DEX) complexed to hydroxypropyl β-cyclodextrin (HPβ-CD, solubilizer) from millirods to inhibit the formation of a fibrous capsule around ablated tissues and to prevent angiogenesis. In this manner, drug penetration might be enhanced due to a lack of capsule formation around the ablation zone [93]. The DEX-HPβ-CD-loaded millirods released 95% of their total drug content within four days in vitro. Loaded millirods were implanted into RF ablated rat livers and the inflammation response was monitored over eight days. Heightened inflammation and formation of a fibrous capsule were observed for unloaded millirods with or without an i.p. injection of DEX (0.26 ± 0.07 mm and 0.29 ± 0.08 mm thick capsules, respectively), while DEX-loaded millirods inhibited significant fibrous capsule formation (0.04 ± 0.01 mm thick) after eight days. Given these promising results, it was indicated that future studies would evaluate millirods loaded with both anti-cancer and anti-inflammatory agents.
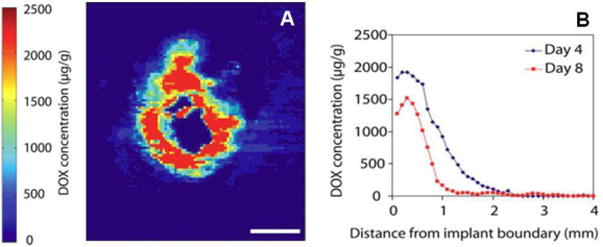
Release of Doxorubicin from drug-loaded PLGA millirods implanted into rabbit liver tumor tissue. A) fluorescence image from representative tissue section (millirod located at center of image); B) quantification of drug diffusion from implant. Scale bar = 2 mm. (adapted from Weinberg et. al.[91])
In a similar approach, Haaga et. al. fabricated extruded cylindrical rods (0.6 – 1.0 mm in diameter) from a copolymer of 1,3 bis(p-carboxyphenoxy propane) and sebacic acid loaded with 5-fluorouracil (5-FU, 15-20% wt/wt) [94]. 5-FU is metabolically converted to its active form (5-FUrd) by viable cells, thus the highest concentration of active drug is expected to be found at the periphery of the ablated region, near the closest region of viable cells to the implant. Following in vivo implantation into ablated rat livers (10 mm ablation diameter), drug concentrations equivalent to a typical systemic dose (12 mg/kg) were observed over a region of radius greater than 8 mm over the first 24 hr, with levels decreasing significantly thereafter. In comparison, 5-FU concentrations were always below therapeutic systemic levels in non-ablated animals. The activated 5-FUrd drug was found at even higher concentrations at the periphery (16 mm away from the ablation center) after 24 and 48 hr compared to the immediate vicinity of the ablation center. Rabbits with VX2 liver tumors treated with RF ablation in conjunction with the drug-eluting implants showed slightly reduced tumor volume (1.8 ± 0.28 cm3) after fourteen days compared to those treated with RF ablation alone (3.5 ± 0.52 cm3).
9. Flexible Film Composites
One particular challenge to local delivery along soft tissue surgical resection margins is administration—achieving adequate surface coverage, fixation, and drug diffusion within the tissue at highest risk for local recurrence. The irregular shape of soft tissues after surgery precludes the use of rigid polymers in most cases, such that other strategies must be employed to provide a flexible, drug-eluting material. While methods such as coating the tissue surface with a polymerizable hydrogel or applying a layer of microparticles via aerosol or adhesion may yield acceptable coverage, other aspects, including lack of controlled release or mechanical stability, often undermine these strategies. As a result, the number of successful studies investigating local delivery to resection margins for recurrence control is limited.
Recently, the groups of Grinstaff and Colson have described flexible composites comprised of multi-layered, drug-loaded films adhered to collagen-based buttressing materials for surgical fixation to tumor resection margins at the time of initial surgery [95-97]. A series of studies demonstrated these composites to be highly effective at completely preventing local recurrent tumor growth in several animal models of local tumor recurrence. The first such investigation evaluated hydroxycamptothecin-loaded poly(glycerol monostearate-co-ε-caprolactone) films in a subcutaneous murine model mimicking residual malignant disease [96]. Camptothecin-based drugs are topoisomerase I inhibitors that are difficult to administer intravenously due to poor aqueous solubility, short in vivo half-lives (i.e. t1/2 = ~14 hr for Irinotecan), and instability from a hydrolysable lactone ring that when opened, significantly reduces the anti-cancer activity of the drug [98]. Hydroxycamptothecin (HCPT) is a camptothecin analogue with high anti-cancer activity but whose use has been limited clinically because of the high systemic toxicity imparted to the patient. Incorporation of HCPT into poly(glycerol monostearate-co-ε-caprolactone) films was expected to facilitate prolonged drug release kinetics while stabilizing the sensitive lactone ring of drug residing in the polymer matrix. Poly(glycerol monostearate-co-ε-caprolactone) is a waxy hydrophobic polymer developed specifically for controlled release applications [99]. An HCPT (2% w/w) in vitro release study showed controlled cumulative release for at least 50 days without a significant initial burst, releasing about 2% of the total dose loading per day for the first two weeks, and gradually decreasing to approximately 0.5% release per day towards the end of release. Significant inhibition of in vitro Lewis Lung carcinoma (LLC) cell proliferation was achieved over the 50-day release period of the films. Next, these films were evaluated in an in vivo model of microscopic lung cancer using the same cell line. Local tumor establishment was defined as tumor growth within 0.5 cm of the implanted films. The films were implanted subcutaneously, the mice allowed to heal, followed by injection of 750,000 LLC cells directly over the surface of the films. Local tumor growth was prevented within the local environment of the implanted chemotherapy-eluting strips in vivo in 86% of implanted mice, whereas those that received unloaded films, experienced local tumor growth directly at the site of tumor injection in 78% of implanted mice. An equivalent dose of HCPT administered intravenously was not effective at preventing local tumor growth. Treated mice showed no evidence of systemic toxicity.
A second study assessed the ability of paclitaxel-loaded (10 % w/w) composites of the same polymer, implanted at the time of the initial tumor resection, to prevent local recurrent tumor growth [95]. An LLC primary tumor was established subcutaneously (300 mm3) in mice followed by surgical resection. Treated mice received the paclitaxel-loaded implant placed within the tissue bed of the resected tumor site. The control animal groups were treated with intraperitoneal or subcutaneous paclitaxel injections, or unloaded composites, and all experienced local recurrence rates greater than 80%. In striking contrast, none of the mice treated with paclitaxel-loaded films developed a recurrent tumor directly at the site of the film and only two mice developed regional tumors when treated with drug-loaded implants (Figure 10). Furthermore, administration of paclitaxel from poly(glycerol monostearate-co-ε-caprolactone) films resulted in a 3000 fold greater tissue concentration of paclitaxel at 10 days compared to systemic paclitaxel administration via intraperitoneal injection (5750 vs. 1.68 ng/g, median, P < 0.05), while the plasma concentration of Paclitaxel following film implantation remianed low (< 10 ng/mL) at both 30 minutes and 10 days, minimizing the risk of systemic toxicity. Healing was clinically indistinguishable between mice receiving paclitaxel-loaded films and mice undergoing sham surgery. There was no evidence of persistent local inflammation, infection, fibrous capsule, or subcutaneous fluid accumulation upon inspection in any of the treatment groups.
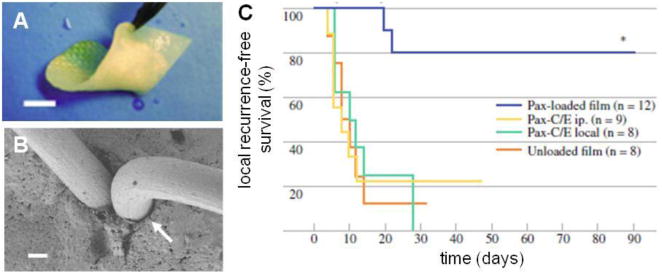
Paclitaxel-loaded poly(glycerol monostearate-co-ε-caprolactone) films prevent local tumor recurrence. A) flexible polymer film coated onto collagen surgical scaffold (scale bar = 5 mm), (B) representative SEM of surgically-stapled film demonstrates good approximation of polymer around staple (scale bar = 100 μm), and (C) prevention of local recurrence with paclitaxel-loaded film compared to intraperitoneal (i.p.), or local paclitaxel injections. (adapted from Liu et. al.[95])
Building upon these results, this technology was assessed in a murine sarcoma model [97]. Soft tissue sarcomas involving the abdomen, pelvis, and retroperitoneum have >50% locoregional recurrence rates following curative resection [100, 101]. The primary cause of death for these patients is locoregional extension rather than distal relapse, which leads to an overall 5-year survival of only 36 to 63% following surgery. Adjuvant chemotherapy and radiation therapy have shown limited benefit, and combined multi-modality treatments have failed to demonstrate superiority. Therefore, prevention of tumor recurrence was assessed using paclitaxel-loaded poly(glycerol monostearate-co-ε-caprolactone) films in an aggressive chondrosarcoma (CS-1) murine post-surgical recurrence model, analogously to the lung cancer recurrence model described above (Figure 11). The observed locoregional recurrence rates following resection were compared for unloaded composites, intravenous paclitaxel injection, or resection alone versus drug-loaded film. Recurrence rates were 69% (9/13), 89% (8/9), and 88% (7/8) for the three controls, respectively, versus only 17% (2/12) for those receiving drug-loaded films following surgical resection. The median time to recurrence was 16, 22, and 13 days for the three control groups, respectively, while ten of twelve mice demonstrated no evidence of recurrence over the 100 day experiment for the group treated with paclitaxel-loaded composites. Additionally, overall survival was increased to 81 days for the paclitaxel-loaded implant group, compared to 64, 48, and 56 days for the respective control groups. This approach holds promise to fulfill a critical clinical need for a drug delivery system that can be molded and secured to a soft tissue surface, provide prolonged therapeutic drug locally, allow unimpaired wound healing, and prevent local tumor recurrence in vivo following surgical resection.
10. Drug Penetration Considerations
Local administration of polymer-based devices ensures high levels of anticancer agents at the site of disease, but the efficacy of these treatments depends upon the accessibility of the delivered therapeutic agent to the tumor and nearby diseased tissue. Adequate diffusion to reach sites harboring occult tumor cells is paramount to preventing recurrence. For instance, lung cancer patients suffer from a 2-fold increase in locoregional recurrence following smaller “wedge” resections (17 – 24%) performed in the setting of limited cardiopulmonary function, as compared to a standard lobectomy resection, whereby ~50% of the entire lung affected by tumor is removed. It is conventionally hypothesized that the risk of local recurrence is related to the presence of residual microscopic disease at or near the surgical resection margins, as evidenced by the observed doubling of recurrence rates following limited resections when the resection margin is less than 1 cm. A recent study retrospectively identified the presence of malignant cells at the surgical margin in 39% of patients following a “curative” limited resection [102], linking the optimal distance of malignant negative margins to the maximum tumor diameter. It is clear that augmenting the effective radius of treatment from the resection margin utilizing local drug delivery technology would increase the potential number of patients cured after “limited” surgical resection and potentially eliminate local recurrence altogether.
The clinical efficacy of brachytherapy in early stage cancer patients combined with the varied levels of success demonstrated by the localized chemotherapy technologies presented here support the hypothesis that local therapy can significantly improve clinical outcomes if the zone of treatment receives a therapeutic dose for a prolonged time period. For the latter, efficacy is correlated to the radial diffusion profile of the embedded drug, as demonstrated in the above lung cancer example. Several mathematical models have been developed to characterize drug transport from a polymer depot in a soft tissue environment[61, 103-106]. Saltzman et. al. developed a model to describe carmustine transport in brain tissue from a polymer composition based on the Gliadel Wafer. Subsequent validation experiments were performed using radio-labeled drug and quantitative autoradiography[103]. Transport kinetics were modeled as an interplay between diffusion balanced against metabolic elimination and permeation into nearby vasculature. Parameters included partitioning, permeation, diffusion, binding, elimination, and drug release kinetics. Tissue samples showed a drug penetration of 5 mm at the end of the first day following implantation, with therapeutic penetration reduced to 1 mm for days 3 - 14. Their model predicted rapid drug elimination beginning on day three and postulated that transient vasogenic edema, resulting from the acute injury of implantation, may be responsible for enhanced drug penetration via convection of interstitial fluid. It is important to note the rapid in vitro release kinetics observed for this delivery system, with 84% cumulative release by 24 hours, thus it is not surprising that penetration distance is reduced following the first several days once the drug diffusion gradient is significantly diminished. Additionally, their model did not take into account interstitial fluid convection, which appeared to play a key role in tissue penetration over the first couple of days following implantation. Arifin et. al. expounded on previous models by adding a component for convection and comparing several ubiquitous chemotherapeutics including carmustine, paclitaxel, 5-fluorouruacil, and methotrexate through simulated flow dynamic calculations[106]. Their model accounted for pressure differentials resulting from interstitial fluid gain from nearby capillary beds. A correlation was observed linking simulated tissue penetration to drug transport due to convection based on the propensity of a particular drug to avoid rapid elimination. Therapeutic concentrations of paclitaxel were predicted to penetrate 1.8 mm into dense tumor tissue while Carmustine, more likely to permeate into nearby vasculature, was simulated to penetrate only to a depth of 0.3 mm.
Additionally, it was recently demonstrated that solid tumors have a greater than 10-fold reduced macromolecule diffusion at depths 500 μm interior to their surface compared to that of at or near the surface[107]. Thiagarajah et. al. suggested that interstitial diffusion is the ratelimiting step in delivering chemotherapy to tumor, and observed an inverse correlation between diffusion rates and the increasing collagen content and density associated with solid tumors. It has been shown in a range of cancers that solid tumors also have high interstitial fluid pressures. This phenomena has been attributed to several mechanisms, including leaky vasculature, interstitial fibrosis and contraction of the interstitial space populated by stromal fibroblasts[108]. Elevated interstitial fluid pressure in solid tumors can reverse the transcapillary flow found in healthy tissues that promotes transport of molecules from inside the capillaries to the interstitial spaces. Thus, increased interstitial pressure is thought to have deleterious effects on systemic chemotherapy delivery to tumor tissue. The effect would likely be similar for localized delivery strategies, where the reversed pressure gradient might expedite drug elimination through increased capillary permeation. For these reasons, solid tumor is not expected to be a conducive environment for achieving a wide spatial distribution of locally administered chemotherapy at therapeutic concentrations, whereas post-surgical therapy at tumor resection margins may represent a more suitable application of local therapy. It is clear that tissue penetration is dependent upon both tissue composition and drug properties. The effect of interstitial fluid flow as a major transport vehicle for those drugs not rapidly eliminated would likely be enhanced in tissues containing lymphatic tissue or that are highly vascularized. The current understanding of tumor physiology and chemotherapy transport demonstrates that the drug can penetrate significant distances at therapeutic concentrations in tissues with normal interstitial fluid pressures and convective flow forces, whereas treating dense tumor presents a challenge as evidenced by the limited efficacy of the Gliadel Wafer. Studies by Weinberg et. al. utilizing the polymer millirods described earlier showing radio-frequency ablation increases drug penetration into tissue suggests that tumor ablation and controlled tissue injury may facilitate increased drug transport throughout the diseased tissue. It may be that localized chemotherapy delivery to solid tumor should be administered in concert with focused radiation, though there are few examples that investigate this hypothesis in the literature.
11. Existing Challenges and Future Outlook
The current standard of care for most primary or recurrent cancers utilizes single or multi-modal combinations of surgery, chemotherapy, and/or radiation depending on the tumor location and patient co-morbidities. The use of chemotherapy for treatment of localized tumor is mostly used as an adjuvant to surgery to protect against or delay the progression of disseminated metastatic disease, or for treatment when other local therapies, such as surgery, are not available. Compared to systemic chemotherapy, localized delivery offers the greatest potential impact as a therapeutic option against early stage cancers with isolated disease for two reasons: First, locoregional recurrence remains a serious mode of failure for many cancers, particularly lung and colon. Local delivery of chemotherapy could sterilize the resection margins, similarly to brachytherapy but without the radiation exposure concerns, thus reducing the incidence of locoregional tumor recurrence. The lack of local targeting by systemic chemotherapy limits its use as a means to prevent local recurrence in all patients. Second, a drug-eluting implant placed at the tumor resection margins would effectively extend the margins to include the depth of therapeutic agents penetrating into the surrounding tissue. This effect could allow for a more limited resection of diseased parenchyma, thus maximizing the amount of functional tissues left behind. For example, limited wedge resections of lung parenchyma could replace the current standard of care whereby the entire lobe of the lung is resected, if locally delivered agents could prevent locoregional recurrence.
It is unclear what role local therapy may have in preventing loco-regional or distal metastasis. Cancer therapy must eliminate all residual malignant tumor cells to be curative, as it is hypothesized that a single remaining cancer cell can grow into a tumor or metastasize into systemic circulation. Distal metastasis is associated with a drastic decrease in survival for cancer patients. Metastasis normally occurs when malignant cells from the primary tumor migrate via vascular or lymphatic channels. Once this occurs, local therapy is likely to be ineffective at preventing secondary tumor growth. What is less certain is if local treatment of the primary tumor environment and regional lymph nodes could prevent metastasis from occurring. One study by Liu et. al. investigated the efficacy of paclitaxel-loaded microspheres embedded in a gelatin matrix at preventing lymphatic metastasis in an orthotopic rat model of lung cancer metastasis[109]. The drug-loaded particles resulted in high therapeutic concentrations in the surrounding local lymph nodes, and were associated with an 80% decrease in lymphatic metastasis compared to controls. This promising data suggests a place for localized therapies in intermediate stage cancer patients.
Additionally, more research needs to be performed characterizing the healing and inflammatory responses invoked by the local accumulation of drugs and polymers, particularly in the post-operative setting. The danger of chemotherapy drugs is two-fold with respect to healing. Anti-cancer agents are often highly cytotoxic and dose-dependent toxicity to healthy parenchyma and supporting connective tissue must be avoided to prevent acute injury. Additionally, anti-proliferative drugs may inhibit normal tissue healing following surgery by retarding the infiltration of immunity cells such as neutrophils and macrophages. As described in the previous section, acute injury at the site of implantation can lead to edema, which may increase drug penetration into the local tissue. However, the foreign-body response to a polymer often leads to the formation of a dense collagenous fibrous capsule around the implant, which would serve as a diffusion barrier to drug release. Thus, understanding the interplay between local chemotherapy delivery and the ultimate effect of healing on interstitial fluid flow and tissue density is important for ensuring that adequate concentrations of drug reach their target over an effective time frame.
As early diagnostic technology improves, the number of patients diagnosed at earlier stages will increase, as will the importance of prophylactic therapies. Advancements in gene sequencing, microarray processing, and mass spectroscopy have accelerated developments in the field of personalized medicine, where the identification of genomic phenotypes is being used to separate patients into unique sub-populations [84]. The ability to screen for early stage disease could dramatically affect the treatments and outcomes of various malignancies, changing the emphasis from late stage disseminated disease to the prevention of local recurrence after initial curative surgery. As the development of more intelligent delivery systems progresses, one can envision a device with programmable delivery to affect all aspects of the disease, from the promotion of normal tissue healing and control of inflammation to the inhibition of tumor growth and angiogenesis. The combination of focused treatment with diminished systemic toxicity represents the next generation of cancer treatments, of which local delivery implants will likely play an integral role in increasing the number of long-term cancer-free patients.
Acknowledgments
This work was supported in part by BU, BWH, CIMIT, NSF DMR-1006601, NIH R01CA149561, and the Boston University's Nanomedicine Program and Cross-Disciplinary Training in Nanotechnology for Cancer, NIH R25 CA153955.
Footnotes
Publisher's Disclaimer: This is a PDF file of an unedited manuscript that has been accepted for publication. As a service to our customers we are providing this early version of the manuscript. The manuscript will undergo copyediting, typesetting, and review of the resulting proof before it is published in its final citable form. Please note that during the production process errors may be discovered which could affect the content, and all legal disclaimers that apply to the journal pertain.
References
Full text links
Read article at publisher's site: https://doi.org/10.1016/j.jconrel.2011.11.031
Read article for free, from open access legal sources, via Unpaywall:
https://europepmc.org/articles/pmc3878823?pdf=render
Citations & impact
Impact metrics
Article citations
Interaction of 6-Thioguanine with Aluminum Metal-Organic Framework Assisted by Mechano-Chemistry, In Vitro Delayed Drug Release, and Time-Dependent Toxicity to Leukemia Cells.
Nanomaterials (Basel), 14(19):1571, 29 Sep 2024
Cited by: 0 articles | PMID: 39404299 | PMCID: PMC11477990
DDS-type near-infrared light absorber enables deeper lesion treatment in laser photothermal therapy while avoiding damage to surrounding organs.
Front Bioeng Biotechnol, 12:1444107, 15 Aug 2024
Cited by: 0 articles | PMID: 39211012 | PMCID: PMC11357940
Genetic Functionalization of Protein-Based Biomaterials via Protein Fusions.
Biomacromolecules, 25(8):4639-4662, 29 Jul 2024
Cited by: 0 articles | PMID: 39074364 | PMCID: PMC11323028
Review Free full text in Europe PMC
Local Delivery of Irinotecan to Recurrent GBM Patients at Reoperation Offers a Safe Route of Administration.
Cancers (Basel), 16(17):3008, 29 Aug 2024
Cited by: 0 articles | PMID: 39272866 | PMCID: PMC11393903
Multi-Sensitive Au NCs/5-FU@Carr-LA Composite Hydrogels for Targeted Multimodal Anti-Tumor Therapy.
Molecules, 29(17):4051, 27 Aug 2024
Cited by: 0 articles | PMID: 39274898 | PMCID: PMC11397649
Go to all (297) article citations
Similar Articles
To arrive at the top five similar articles we use a word-weighted algorithm to compare words from the Title and Abstract of each citation.
Biodegradable polymers for ocular drug delivery.
Ophthalmologica, 215(3):143-155, 01 May 2001
Cited by: 45 articles | PMID: 11340382
Review
Prevention of local tumor recurrence following surgery using low-dose chemotherapeutic polymer films.
Ann Surg Oncol, 17(4):1203-1213, 03 Dec 2009
Cited by: 31 articles | PMID: 19957041
Enhanced apoptotic and anticancer potential of paclitaxel loaded biodegradable nanoparticles based on chitosan.
Int J Biol Macromol, 98:810-819, 09 Feb 2017
Cited by: 25 articles | PMID: 28189791
Tumor Targeting of Polymeric Nanoparticles Conjugated with Peptides, Saccharides, and Small Molecules for Anticancer Drugs.
Curr Pharm Des, 23(35):5349-5357, 01 Jan 2017
Cited by: 7 articles | PMID: 28911307
Review
Funding
Funders who supported this work.
NCI NIH HHS (3)
Grant ID: R01 CA149561
Grant ID: R25 CA153955
Grant ID: R25 CA153955.