Abstract
Free full text

Innate and adaptive immune cells in the tumor microenvironment
Abstract
Most tumor cells express antigens that can mediate recognition by host CD8+ T cells. Cancers that are detected clinically must have evaded antitumor immune responses to grow progressively. Recent work has suggested two broad categories of tumor escape based on cellular and molecular characteristics of the tumor microenvironment. One major subset shows a T cell–inflamed phenotype consisting of infiltrating T cells, a broad chemokine profile and a type I interferon signature indicative of innate immune activation. These tumors appear to resist immune attack through the dominant inhibitory effects of immune system–suppressive pathways. The other major phenotype lacks this T cell–inflamed phenotype and appears to resist immune attack through immune system exclusion or ignorance. These two major phenotypes of tumor microenvironment may require distinct immunotherapeutic interventions for maximal therapeutic effect.
The prospect of effective immunotherapies for the treatment of patients with cancer is now becoming a clinical reality. The foundation of contemporary tumor immunology and cancer immunotherapy arguably lies in the molecular identification of tumor antigens1–3. Although early application of those discoveries was focused on tumor antigen–based therapeutic cancer vaccines, recent accelerated progress has been driven by a greater understanding of immunoregulatory processes that principally are active in the tumor microenvironment. Increasing our understanding of the fundamental details of the tumor-host interaction, both in human tissue-based studies and through mechanistic experiments using mouse models, is accelerating the pace of therapeutic development. The approval by the US Food and Drug Administration in 2011 of the anti–CTLA-4 monoclonal antibody ipilimumab for the treatment of patients with advanced melanoma4 represents the first-in-class strategy of uncoupling inhibitory pathways downstream from initial antigen recognition. Continued detailed analysis of the immunologic features of the tumor microenvironment is enabling rapid development of multiple new immunotherapeutic strategies as well as the identification of potential biomarkers for clinical benefit.
Tumor cells are antigenic
The molecular identity of antigens that can be expressed by malignant cells and recognized by host T cells is now well established5. Most early efforts at antigen identification and selection for therapeutic targeting focused on shared tumor antigens, which have the practical advantage of being applicable to a broad range of cancer patients6. It is becoming increasing clear, however, that many of these shared antigens are expressed at some level by self tissues, either in peripheral cells or in the thymus, which can lead to immunologic tolerance for the highest-avidity interactions between peptide, major histocompatibility complex and T cell antigen receptor (peptide-MHC-TCR). As such, immune responses generated against such antigens can be restricted to lower-avidity interactions, which may limit therapeutic efficacy7. However, neoantigens generated by point mutations in normal genes, which usually are unique to individual tumors, can result in much more potent antitumor T cells. The most critical component of this complex multimolecular binding interaction may be the avidity of the interaction between the antigenic peptide and the MHC molecule8. Defining mutant antigens in both mouse and human cancers is being empowered by remarkable advances in exome sequencing9,10. In addition, excellent databases for predicting binding of individual peptide epitopes to specific MHC molecules (for example, HLA-A2) have been established11. With these tools, defining the landscape of ‘mutatopes’ for individual cancers is becoming a reality.
Some cancers display hundreds or even thousands mutations in coding exons, representing a large repertoire of antigens to serve as potential targets for recognition by the immune system. But despite expression of abundant antigens, most cancers progress and evade immune system–mediated destruction. Although it was initially presumed that failed spontaneous immune system–mediated tumor rejection would likely be due to immunologic ignorance and defects in the initial priming of antitumor T cells, this appears not to be the case in a major subset of patients in whom spontaneous antitumor immune responses can be demonstrated. Patients who do and do not show evidence of induction of spontaneous tumor antigen–specific T cell responses may ultimately require distinct therapeutic interventions; therefore, defining these immune phenotypes may aid in predictive biomarker development for classes of immunotherapeutics.
Immunophenotypes of human cancer
Analysis of the tumor microenvironment in patients with a variety of solid tumors has revealed that a major subset of tumors shows evidence of a T cell–infiltrated phenotype (Fig. 1a). In early stage colorectal cancer, the presence of activated CD8+ T cells both within the tumor and in the peritumoral stroma has been shown to have significant positive prognostic import12,13. Early analyses suggest that the prognostic value of this immunophenotype may be more powerful than traditional staging; the majority of patients with stage I and stage II cancer who lack a T cell infiltrate develop disease recurrence within 5 years, whereas the presence of a T cell infiltrate in patients with stage III cancer can predict an unusually long disease-free interval14. The characterization of this simple immune system–based biomarker has been termed the ‘immunoscore’, and its application is currently being validated in a multicenter international study15.
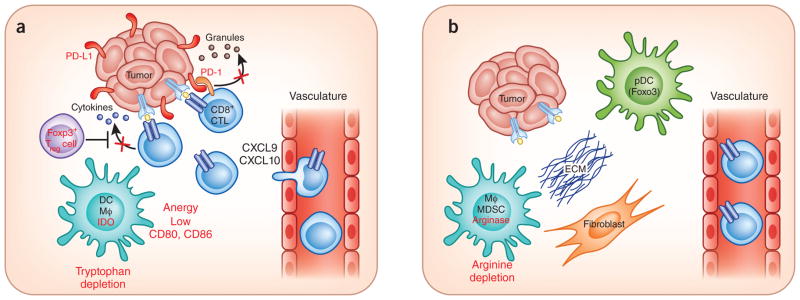
Working model for the segregation of tumors based on immune system regulatory pathways in the tumor microenvironment. (a) In T cell–infiltrated tumors, chemokines support influx of CD8+ effector T cells, but these subsequently become functionally inhibited by the effects of PD-L1, IDO, Treg cells and anergy. The development of this phenotype appears, in part, to be promoted by type I interferon signaling and the CD8 α+ DC lineage. (b) In non–T cell–infiltrated tumors, there is poor chemokine expression and lack of T cell infiltration but also minimal presence of defined immune inhibitory pathways. It is speculated that these tumors also have denser stroma and alternative myeloid or macrophage (MΦ) populations. Although this distinction has been best characterized in patients with melanoma, similar immune phenotypes may be operational in other solid tumors in which a subset of tumors shows T cell infiltration.
A subset of patients with other solid tumor histologies also appears to have a spontaneous T cell infiltrate that may have similar positive prognostic value. This includes breast cancer, renal cell carcinoma, melanoma, ovarian cancer and gastrointestinal stromal tumors (GIST)16–20. A high ratio of CD8+ T cells to Foxp3+ regulatory T cells (Treg cells) in the ovarian cancer tumor microenvironment has been associated with a particularly favorable clinical outcome21. A presumption is that a component of this T cell infiltrate includes tumor antigen–specific T cells that have been activated spontaneously in response to the growing tumor, perhaps through immune system surveillance mechanisms22, that are making an attempt to control the tumor via immune effector functions and thus creating a more favorable clinical outcome. It is important to consider, however, that the accumulation of T cells in the tumor microenvironment could be a secondary phenomenon, reflecting a distinct underlying tumor biology and activity of different oncogenic pathways23,24 that themselves could portend diminished capacity for progression of disease.
In the case of metastatic melanoma, it has been demonstrated in several small studies that CD8+ T cells specific for defined melanoma antigens can be identified in the tumor microenvironment based on peptide-MHC tetramer analysis. However, functional analysis has revealed that those T cells can display blunted cytokine production and proliferation when analyzed directly ex vivo25–27. Stimulation and cytokine-mediated expansion of those T cells in vitro can restore their functional properties, suggesting that a major component of this dysfunction is reversible. In fact, it is tumor-infiltrating T cells that form the starting point of the adoptive T cell transfer protocols developed by Steven Rosenberg and colleagues in melanoma that have consistently yielded clinical response rates of 50% or greater, in selected patients28. Together, these and other observations have pointed toward the likelihood that dominant inhibitory pathways are operational, at least in the melanoma context, that blunt the function of those T cells in the tumor microenvironment and ultimately allow tumor outgrowth. In fact, firm evidence indicates that these tumors show high expression of PD-L1 and indoleamine-2,3-dioxygenase (IDO), and display high infiltration with CD4+Foxp3+ cells29. Recent work has suggested that the upregulation of PD-L1 and IDO is driven by interferon-γ (IFN-γ) produced by CD8+ T cells in vivo and that accumulation of regulatory T cells is also CD8+ T cell–dependent through the production of the chemokine CCL22 that can recruit Treg cells via CCR4 (ref. 30). Indirect evidence also suggests that classical T cell anergy leading to T cell–intrinsic dysfunction also contributes to immune escape31,32. Recent work on the molecular characterization of anergic T cells has revealed expression of LAG-3 and other factors33, which themselves could be immunoregulatory and amenable to pharmacologic manipulation. Identification of a major subset of human cancers that shows preexisting CD8+ T cell infiltration and high expression of defined immune system–inhibitory pathways has important clinical implications for immunotherapeutic approaches aimed at blockade of those pathways, as discussed below.
Innate immune system activation and dendritic cell subsets
A major conundrum has been how it is possible that even a subset of patients can generate a spontaneous CD8+ T cell response against tumor-associated antigens, apparently in the absence of pathogen involvement. This narrows to a question of mechanisms of sterile immunity and suggests the likely participation of stress-associated or damage-associated molecular patterns that may trigger innate immune activation and bridge toward adaptive immunity. Interrogation of melanoma gene expression profiling data revealed that tumors infiltrated with CD8+ T cells also showed expression of a type I interferon transcriptional signature29. This observation raised the possibility that type I interferon signaling might participate in innate recognition of tumors. Indeed, gene-targeted mice deficient in type I interferon receptor (IFNR) or the downstream transcription factor STAT1 showed near complete loss of spontaneous T cell priming in transplantable tumor models34–36. Type I IFNR–deficient mice also displayed increased tumor induction in a carcinogen-induced cancer model using methylcholanthrene37. The functional role for type I interferon signaling mapped to the host antigen-presenting cell compartment, suggesting a defect at the level of dendritic cells (DCs). However, most measurable DC functions seemed to be intact in these mice, including numbers of myeloid DCs, plasmacytoid DCs (pDCs) and CD8α+ DCs in secondary lymphoid organs, upregulation of costimulatory ligands in response to Toll-like receptor (TLR) agonists and migration from the skin to draining lymph nodes as assessed by fluorescein isothiocyanate painting. However, a major defect was observed when the tumor microenvironment was evaluated, in which accumulation of the CD8α+ DC subset was nearly completely absent. Spontaneous priming of CD8+ T cells, and rejection of immunogenic tumors, was eliminated in Baft3−/− mice defective in the CD8α+ DC lineage34,38. Conditional deletion of the type I IFNR in the DC compartment, as well as mixed bone marrow chimera experiments, revealed a critical functional role for type I interferon signaling specifically in the CD8α+ DC lineage.
The mechanism by which tumors induce type I interferon production by host DCs is a topic of active investigation. Infectious disease models have indicated at least three pathways of innate immune sensing that can drive transcription of the Ifnb gene: TLR signaling through the adaptors MyD88 and TRIF, RIG-I sensing of cytosolic RNA leading to signaling through the adaptor IPS-1, and the STING pathway sensing cytosolic DNA39,40. Preliminary data indicate spontaneous CD8+ T cell priming against tumor-associated antigens is preserved in mice deficient in MyD88, TRIF and IPS-1, but is lacking in Tmem173−/− (STING-deficient) hosts (T.F.G. unpublished observations). The possibility that DNA derived from dying tumor cells may be the critical ligand for induction of IFN-β production by DCs through the STING pathway is currently being investigated.
Parallel work has indicated that CD8α+ DCs are particularly effective at acquiring antigen from dying cells, including tumor cells, and targeting antigen for cross-presentation via the class I MHC processing pathway. This effect is mediated, in part, through surface expression of Clec9a (also known as DNGR-1)41–43. Recent work has identified polymerized actin as a major ligand for DNGR-1, which is exposed after cell necrosis44. Thus, an attractive model to consider is that a subset of tumor cells may die in such a way as to expose F-actin for recognition by DNGR-1–expressing CD8α+ DCs, which under the influence of type I interferons lead to productive cross-presentation to CD8+ T cells.
In addition to CD8α-lineage DCs, immunoregulatory properties of pDCs in the tumor microenvironment also have been suggested, but in this case in an inhibitory direction. DCs with a pDC phenotype appear to be tolerogenic when collected from tumors and analyzed ex vivo45. This is despite the fact that properly activated pDCs can effectively prime T cells in vitro46,47. These intratumoral DCs can express IDO and PD-L1, which may contribute to their suppressive phenotype, and show defective production of type I interferon48–50. Recent work has identified a critical role for the transcription factor Foxo3 in the acquisition of inhibitory properties in tumor-infiltrating DCs; knockdown of Foxo3 can partially restore DC stimulatory function51. Understanding the mechanism by which the tumor microenvironment context promotes Foxo3 expression will be important to pursue.
The characterization of the functional importance of the CD8α+ DC and pDC phenotypes in mouse tumor models has provided a conceptual framework for analyzing DCs from the tumor microenvironment in human cancer specimens. The human CD8α+-like DC lineage lacks CD8 expression but does express DNGR-1 (refs. 52,53). An attractive hypothesis is that the presence of DNGR-1+ DCs may be associated with a productive endogenous antitumor T cell response but that the presence of Foxo3+ DCs may be associated with T cell tolerance. These questions can be addressed with currently available tools.
Tertiary lymphoid structures in the tumor microenvironment
A second hypothesis that could explain the presence of T cells in the tumor microenvironment in a subset of cancers is the generation of tertiary lymphoid structures (TLSs). Many tumors appear to have an organizational architecture that includes B cell–T cell segregation and the presence of a high endothelial venule–like vascular structure54,55. T cell–infiltrated tumors express chemokines that likely mediate recruitment of CD8+ effector T cells but also express CCL21 that recruits naive T cells and activated DCs54,56. These features resemble the characteristics of lymph nodes and suggest that TLSs are present in a subset of tumors. Expression of the TNF superfamily 14 protein (LIGHT) has also been detected in a subset of human melanomas57, which are sufficient in mouse models to induce lymph node–like structures in vivo58. Whether TLSs observed in tumor tissues depend on LIGHT or lymphotoxin remains to be determined.
Although lymphocytes in some cases contribute to support of tumor growth, particularly in inflammation-induced cancer models59,60, it is not clear whether induction of TLSs per se would provide a growth advantage for cancers in vivo. The generation of these structures may represent a component of the host immune response to growing tumors. As their presence is also associated with the accumulation of CD8+ effector T cells, it is conceivable that spontaneous priming of an antitumor T cell response leads to chronic inflammation in the tumor microenvironment that subsequently generates signals for generation of TLSs. This picture may be analogous to the TLSs seen in chronic inflammatory conditions, such as rheumatoid arthritis. In contrast, expression of CCL21 in tumors is capable of promoting immunologic tolerance, presumably owing to recruitment of naive T cells specific for tumor antigens that then become inactivated through antigen presentation without proper costimulation61. The functional relationship between these components of the host response will be important to decipher in future studies.
Innate lymphocyte subsets
Besides conventional aβ TCR–expressing T cells, natural killer (NK) cells, gδ T cells and natural killer T (NKT) cells are present in the tumor microenvironment in various cancer settings. A substantial body of evidence suggests that NKG2D recognition of Rae-1 family ligands in the mouse or the MICA and MICB ligands in humans contribute to recognition of tumors by the immune system62–64. Upregulation of NKG2D ligands links the DNA-damage response and also to cell-cycle progression through E2F transcription factors65,66. Although the ability of NK cells to contribute to host control of hematologic malignancies has been well-documented, recent work has indicated that NK cells also can contribute to tumor control in solid tumors. The ability of interleukin 15 (IL-15) and IL-15 receptor α(IL-15Rα) expression in the tumor microenvironment to impart control of solid tumors by the host immune system is predominantly mediated by NK cells in mouse models67. In patients with GIST, evidence has implicated NK cells and NKp30 isoforms in the clinical response to treatment with imatinib68. Engineering T cells to express NKG2D-based chimeric antigen receptors also has been found to be therapeutic via adoptive transfer in mouse models in vivo69,70. In human patients with cancer, the presence of circulating shed MICA or MICB ligands has been associated with downmodulation of NKG2D on effector T cells and diminished cytolytic activity71. Some patients treated with the immune system–potentiating anti–CTLA-4 monoclonal antibody ipilimumab have been found to develop high titers of anti-MICA antibodies, which may be associated with clinical benefit72. Together, these data argue for a contribution of NKG2D-mediated tumor recognition via both NK cells and activated T cells both in mouse and human models.
T cells expressing gδ TCRs also have been implicated in immunomodulation in the tumor microenvironment. Antitumor effects of gδ T cells have been reported73,74, and adoptive transfer strategies using Vγ9Vd2 TCR–transduced T cells are being evaluated because of the direct tumor recognition by these receptors75. In human patients with cancer, the use of the bisphosphonate zoledronic acid has been associated with augmented gδ T cell function76,77. However, gδ TCR–expressing T cell clones isolated from human patients with breast cancer were found to be suppressive for activation of T cells in vitro, arguing that this subset can have regulatory function in certain circumstances78. The conditions under which gδ T cells can contribute to tumor control versus immune suppression need to be better defined.
Preclinical data have suggested that NKT cells also can exert either anti-tumor or immunoregulatory activities, depending on the immunologic context and perhaps the TCR being used. Invariant NKT cells expressing the Va14Ja18 receptor recognize the antigen α-galactosylceramide presented by CD1d and typically produce IFN-γ and support tumor control in vivo79–81. In contrast, a subset of NKT cells appears to be able to suppress productive antitumor immunity, in part via the production of IL-13, which, in turn, induces TGF-β production by myeloid cells82. This latter subset appears to lack the invariant CD1d-restricted TCR and likely recognizes distinct antigens. Vaccination with α-galactosylceramide–pulsed DCs to expand and activate NKT cells in human cancer patients is also being evaluated and has shown clinical activity in multiple myeloma83,84.
Together, these observations suggest that the functional role of these innate lymphoid cells in antitumor immunity is complex. A positive or negative immune system regulatory contribution should be assessed carefully in distinct human cancer types. A better definition of the tumor-expressed ligands recognized by these cell subsets will facilitate more detailed functional analyses and identify possibilities for therapeutic intervention.
Regulation by additional stromal components
In additional to the lymphoid and dendritic cell lineages that can be found to infiltrate subsets of tumors, classical cellular components of the solid tumor microenvironment also influence the host immune response. It has long been recognized that tumor fragments that contain stromal elements can be much more difficult to reject immunologically compared to a tumor cell suspension when implanted into mice in vivo85. This solid tumor stroma consists of fibroblasts, macrophage-lineage cells and vascular endothelial cells, with variable amounts of extracellular matrix, all of which contribute as a support structure for tumor growth. But in addition to positively regulating tumor growth, these components can impair host immune responses and likely contribute to the degree of immune cell infiltration.
One major marker expressed on tumor-associated fibroblasts is fibroblast-activating protein (FAP). Fearon and colleagues have generated xxxxxxx mice, which can eliminate these fibroblasts from the tumor microenvironment. FAP-based deletion led to tumor regression by a mechanism that was dependent on host immunity, in particular on IFN-γ and TNF86. A vaccination approach against FAP as a self-antigen also has been pursued, which led to improved immune system–mediated tumor control and decreased collagen deposition in the tumor87. These results argue that fibroblasts in the tumor microenvironment can impede antitumor immune responses. However, FAP itself, in a direct functional role, also may contribute. Small-molecule inhibitors of FAP enzymatic function have been developed and appear to increase the concentrations of multiple cytokines in mouse models in vivo88. Some of these inhibitors have been moved forward into early phase clinical trials in patients, with evidence of target inhibition89. Collagen deposition in the tumor microenvironment also has been directly implicated as a barrier to T cell entry. Degradation of collagen in an ex vivo model resulted in markedly augmented penetration of T cells in the solid tumor microenvironment, enabling direct contact of T cells with tumor cells90.
Subsets of human tumors with poor T cell infiltration (Fig. 1b) appear to have higher expression of several angiogenic factors56,91. In an ovarian cancer model, Coukos and colleagues have isolated vascular endothelial cells from T cell–infiltrated versus non–T-cell–infiltrated tumors and performed gene expression profiling to identify molecular phenotypes associated with T cell exclusion. This analysis identified the endothelin B receptor as a candidate regulator, and inhibition of this receptor could improve T cell trafficking91. T cell migration into the tumor microenvironment is thought to depend on binding to ICAM-1, VCAM-1 and selectins on vascular endothelial cells92–94, and also on local production of specific chemokines. The presence of CD8+ T cells in the melanoma microenvironment is associated with local production of CCL2, CCL3, CCL4, CCL5, CXCL9 and CXCL10 (ref. 56). Correlative evidence suggests a particular importance of CXCL9 and CXCL10, which engage CXCR3 on the surface of CD8+ effector T cells95,96. Although these chemokines can in some cases be produced by tumor cells56, they also can be secreted by stromal cells in the tumor microenvironment and thereby contribute to recruitment of effector T cells.
Macrophages and other myeloid cells are universally found in the solid tumor microenvironment and can contribute to immune evasion. Tumor-promoting M2 macrophages are induced under the influence of IL-4, IL-13, IL-10 and M-CSF and lack the cytotoxicity of M1 macrophages that are typically induced under the influence of GM-CSF, IFN-γ and TLR agonists. M2 macrophages appear to contribute to immune suppression through the production of IL-10 and TGF-β97. They also can contribute to recruitment of Treg cells through production of CCL22 (ref. 21). Myeloid-derived suppressor cells are immature myeloid-lineage cells that also can be immunosuppressive in the tumor microenvironment. Major mechanisms of suppression include the expression and functional activity of arginase98 and the nitrosylation of surface proteins on infiltrating T cells, including the TCR99. Gene expression profiling studies of human melanoma have revealed arginase transcripts expressed in a subset of non–T cell–infiltrated tumors56, so myeloid-derived suppressor cells may be a component of the phenotype of T cell exclusion. Gaining a better molecular characterization of myeloid-macrophage subsets in T cell–infiltrated versus non–T cell–infiltrated human cancer specimens is warranted.
In mouse models, CD11b+ cells in the tumor microenvironment can take up and present tumor-derived antigens in vivo, using high-affinity TCR tetramers that recognize specific peptide-MHC complexes100. Adoptive transfer of sufficient numbers of T cells with transgenes encoding specific TCRs can lead to recognition and killing of those antigen-expressing myeloid cells, provided they traffic and penetrate into the tumor microenvironment. Thus, highly effective T cell therapies could overcome this mechanism of immune escape and render it non–rate-limiting, provided that tumor-derived antigens are cross-presented. In fact, T cell–mediated killing of stromal cells alone can be sufficient for control of tumors in vivo100.
Studies of agonistic monoclonal antibodies against CD40, which were initially developed as a strategy for DC activation in vivo, revealed an unexpected activity on the tumor microenvironment in pancreatic cancer. An early phase clinical trial of anti-CD40 plus gemcitabine chemotherapy generated an unusually high clinical response rate. Preclinical modeling using a genetically engineered pancreatic cancer model revealed that anti-CD40 treatment markedly augmented influx of macrophages into the tumor site, which appeared to severely disrupt the tumor stroma101. Such stromal collapse can lead to increased concentrations of chemotherapeutic agents accumulating in the tumor site but also could theoretically favor increased infiltration of activated T cells.
Theoretical sources of interpatient heterogeneity
The fact that a subset of patients generates a spontaneous tumor antigen–specific CD8+ T cell response while another major subset does not, raises the fundamental question of the underlying molecular mechanisms that explain these two phenotypes. This question can be reduced to the notion of sources of interpatient heterogeneity in individual patients with cancer, which can be divided into three categories.
First, it is possible that somatic differences in the tumor cells themselves influence the host response, via involvement of different oncogene pathways that are mutated or activated in a heterogeneous fashion and that regulate the expression of immune system regulatory genes. In the melanoma example, most tumors have driver oncogene mutations that activate the Ras pathway, involving wither B-Raf, N-Ras or growth factor receptors102–104. However, other pathways are variably activated, including STAT3, phosphatidylinositol-3-OH kinase (PI(3)K), Notch and β-catenin105–110. Preclinical data have revealed that tumor cell lines with STAT3 activation show poor expression of chemokines and cyto-kines, but small interfering RNA–mediated knockdown of STAT3 could markedly augment production of these factors111. In addition, STAT3 positively controls expression of immunosuppressive factors. Thus, STAT3 activation in tumor cells may represent one signaling pathway that contributes to immune exclusion. Analogous functional studies of other candidate pathways are warranted.
A second category of interpatient heterogeneity is at the level of germ-line polymorphisms in immune system regulatory genes. It is not difficult to imagine thresholds for innate immune system stimulation and/or T cell activation being influenced by the sensitivity of signaling pathways governed by gene polymorphisms, as has been observed for human autoimmune diseases. The first example to be characterized in association with antitumor immunity in patients is a common CCR5 polymorphism that was correlated with favorable clinical benefit to high-dose IL-2 (ref. 112). A recently identified IRF5 polymorphism was associated with clinical response to tumor-infiltrating lymphocyte adoptive therapy in melanoma113.
A third category of interpatient heterogeneity can broadly be designated as environmental in nature. Although differential exposure to certain pathogens could change the activation status and/or frequency of subsets of T cells in the peripheral repertoire, it is not clear whether such features are associated with the presence or absence of a spontaneous antitumor T cell response. However, recent discoveries implicating composition of the intestinal microbiome in regulation of systemic immune responses have generated an attractive hypothesis that is clinically testable. In mouse studies, the presence or absence of a single species of commensal bacteria can have a profound influence not only on inflammatory bowel disease but also on the development of inflammatory arthritis114,115. The availability of high-throughput platforms for analyzing the composition of intestinal flora by 16S rRNA sequencing from stool samples makes it feasible to determine whether specific bacterial species are associated with the presence of a spontaneous antitumor T cell response in patients. Collectively, these concepts support a systematic analysis of tumor genomics, host genetics and intestinal micro-biome as they relate to the presence or absence of a spontaneous T cell infiltrate in human patients with cancer. Identification of the underlying molecular mechanisms of this process may provide new avenues for therapeutic intervention.
Clinical therapeutic implications
The refined definition of immune system regulatory features of the tumor microenvironment in human patients with cancer has several critical implications for clinical translation. Early data have suggested that the presence of a T cell–inflamed tumor microenvironment may serve as a predictive biomarker for response to immunotherapies. This includes therapeutic cancer vaccines116, the anti–CTLA-4 monoclonal antibody ipilimumab117,118 and high-dose IL-2 (ref. 119). Prospective studies are needed to confirm these early findings, and one such study is ongoing by GlaxoSmithKline-Bio using their MAGE-3 protein vaccine platform5. This working model makes sense, as tumors that cannot support trafficking of activated T cells into the tumor microenvironment might not be able to respond clinically to a variety of current immunotherapies.
The identification of defined immunosuppressive pathways that are present in the tumor microenvironment in the subset of T cell–inflamed tumors has pointed toward therapeutic targets that are amenable to clinical intervention (Fig. 2). These include the PD-L1–PD-1 axis, IDO, Treg cells and T cell–intrinsic anergy. Blocking monoclonal antibodies against PD-1 or PD-L1 have entered phase 1 and/or phase 2 clinical trial testing in patients with advanced cancer. An ~30% response rate has been seen in patients with melanoma, with additional frequent clinical responses being observed in renal cell carcinoma and non–small cell lung cancer120,121. Preliminary biomarker data have indicated that clinical activity might be confined to the subset of patients with tumors that have PD-L1 expression and CD8+ T cell infiltration in the tumor microenvironment122. Phase 1 testing of small-molecule inhibitors that block IDO enzymatic activity also has completed123. Biologically active doses have been identified that reverse the tryptophan:kynurenine ratio in the blood, and phase 2 studies have been initiated. Reduction of Treg cell numbers is being pursued using agents that target surface expression of CD25. The IL-2–diptheria toxin fusion protein denileukin diftitox has been reported to reduce circulating numbers of Treg cells in cancer patients124,125, although not all studies have yielded positive results126. A multicenter phase 2 study is ongoing in patients with advanced melanoma. Anti-CD25 monoclonal antibodies also are being evaluated clinically. A single dose of the anti–human CD25 monoclonal antibody daclizumab has been reported to durably reduce the numbers of circulating Treg cells without impairing vaccine-induced T cell responses127. Regarding T cell anergy, preclinical models show that exposure to homeostatic cytokines can support proliferation of anergic T cells out of their dysfunctional state, both in vitro and in vivo32,128. Early phase clinical trials of IL-7, IL-15 and IL-21 are at various stages of completion129,130. A phase 2 clinical trial of IL-21 in melanoma demonstrated a clinical response rate of 22%131; tumor-based biomarkers were not analyzed in that study, but it is attractive to consider that induced proliferation of T cells may have restored key functional properties. The liberation of host IL-7 and IL-15 that occurs with lymphopenic conditioning regimens for adoptive T cell therapy supports homeostatic proliferation that results in expansion of T cells but also could maintain responsiveness of T cells and uncouple induction of anergy132–134.
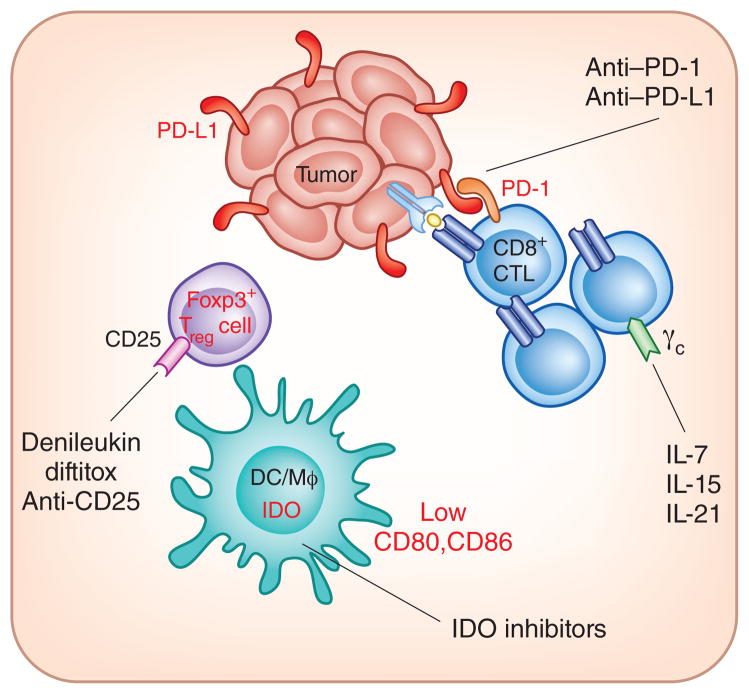
Therapeutic interventions being investigated that target immune inhibitory pathways in the tumor microenvironment. In melanoma, T cell–infiltrated tumors show the highest expression of PD-L1, IDO and Treg cells, and indirect evidence suggests T cell–intrinsic anergy as well. Monoclonal antibodies blocking PD-1–PD-L1 interactions, small-molecule inhibitors of IDO, CD25-targeting agents to deplete Treg cells and γc-binding cytokines to promote homeostatic proliferation of T cells and anergy reversal are all being investigated clinically.
It should be evident that, because the T cell–infiltrated tumor micro-environment phenotype contains multiple distinct factors that inhibit T cell effector functions, there could be compensation such that blockade of two or more pathways may be necessary for optimal therapeutic efficacy. Preclinical studies support this notion. Combinatorial blockade of CTLA-4 plus PD-L1, dual inhibition of LAG-3 and PD-1 or depletion of Treg cells plus homeostatic proliferation to uncouple anergy have all been shown to be synergistic in mouse models134–136. As such, a high priority is being placed on logical combination immunotherapies in cancer patients.
A major therapeutic barrier may remain for the patients showing the non–T cell–infiltrated tumor microenvironment phenotype (Fig. 3). It has not yet been well defined whether currently available immuno-therapies may help to modulate favorably the completely noninflamed tumor sites. These tumors appear to lack a type I interferon signature, chemokines for recruitment of T cells and evidence for T cells per se. The vasculature may be nonpermissive for entry by T cells, and the composition of stromal elements may prevent trafficking and/or function of T cells. Thus, therapeutic interventions for this subset of tumors may need to focus on strategies to induce appropriate tissue-based inflammation. Preclinical models have demonstrated that intratumoral administration of IFN-β137, introduction of the TNF superfamily member LIGHT58,138 or local radiation therapy137 all can favorably alter the tumor microenvironment and support improved trafficking of T cells. Recent work has indicated that radiation of tumors actually induces production of IFN-β and augments function of intratumoral DCs, and also results in improved accumulation of T cells that all are necessary for optimal therapeutic efficacy139. Targeted inhibition of specific oncogene pathways may favor priming of the immune system and recruitment of T cells. Mouse models have indicated that elimination of driver oncogene expression can lead to tumor regression, which is, in part, immune system–mediated140. Imatinib in GIST also appears to improve antitumor immune responses, in part via downmodulation of IDO141. In patients with melanoma, inhibition of B-Raf activity with vemurafenib can induce a T cell infiltrate in the tumor microenvironment within 1–2 weeks of therapy142. Several traditional chemotherapeutic drugs trigger innate immune activation and adaptive T cell responses that contribute to the therapeutic effect. This process involves immunogenic cell death, sensing of extracellular ATP and activation of antigen-presenting cells through TLRs and/or the inflammasome143–146.
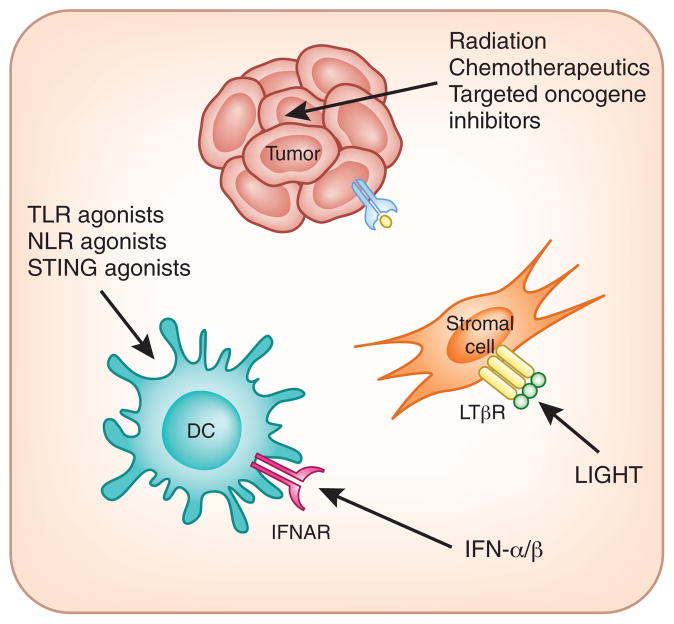
Therapeutic strategies being considered that may promote appropriate inflammation and/or innate immune activation in the tumor microenvironment. Non–T cell–infiltrated tumors may require novel interventions to trigger innate immune activation and to facilitate signals for effector T cell trafficking. Radiation therapy, some chemotherapy drugs and targeted oncogene pathway inhibitors may achieve this effect through immunogenic cell death and altered tumor cell biology. The TNF superfamily member LIGHT induces chemokine production by stromal cells via binding to the LTβR. Agonists of innate immune pathways including TLRs, NLRs and STING are being considered to activate DCs and initiate productive priming of T cells. Introduction of type I interferon into tumor sites also may activate dendritic cells through the IFN-αR and/or IFN-βR and has additional effects on the tumor vasculature. IFNAR
It is reasonable to consider that combination regimens consisting of strategies to improve innate immune system activation and T cell trafficking into the tumor microenvironment, along with vaccination or adoptive T cell transfer to increase the frequency of antitumor T cells and finally blockade of immune inhibitory pathways, all may be necessary to achieve clinical benefit in patients with the noninflamed tumor phenotype. Recent preclinical data have supported this notion, as combination treatment with local radiation and either anti–CTLA-4 or anti–PD-L1 has shown improved efficacy in vivo147,148.
Conclusions
Characterization of the tumor microenvironment in human patients with cancer reveals evidence for distinct immunologic phenotypes based on the presence or absence of T cell–based inflammation. These observations have generated candidate predictive biomarkers for response to immunotherapies and are guiding the identification of new immunotherapeutic interventions. T cell–infiltrated tumors may optimally respond to therapies targeting immune system inhibitory mechanisms. Non–T cell–infiltrated tumors may require additional interventions aimed at promoting optimal inflammation and innate immune activation in the tumor microenvironment. Combination immunotherapies are already entering the clinical arena with encouraging early phase clinical trial data.
Footnotes
COMPETING FINANCIAL INTERESTS
The authors declare no competing financial interests.
Reprints and permissions information is available online at http://www.nature.com/reprints/index.html.
References
Full text links
Read article at publisher's site: https://doi.org/10.1038/ni.2703
Read article for free, from open access legal sources, via Unpaywall:
https://europepmc.org/articles/pmc4118725?pdf=render
Citations & impact
Impact metrics
Article citations
Copper and Colorectal Cancer.
Cancers (Basel), 16(21):3691, 31 Oct 2024
Cited by: 0 articles | PMID: 39518128 | PMCID: PMC11544869
Review Free full text in Europe PMC
Exosome therapeutics for non-small cell lung cancer tumorigenesis.
Cancer Cell Int, 24(1):360, 30 Oct 2024
Cited by: 0 articles | PMID: 39478574 | PMCID: PMC11523890
Review Free full text in Europe PMC
Solute carrier family 4 member 4 (SLC4A4) is associated with cell proliferation, migration and immune cell infiltration in colon cancer.
Discov Oncol, 15(1):597, 28 Oct 2024
Cited by: 0 articles | PMID: 39467887 | PMCID: PMC11519258
Th17 cell function in cancers: immunosuppressive agents or anti-tumor allies?
Cancer Cell Int, 24(1):355, 27 Oct 2024
Cited by: 0 articles | PMID: 39465401 | PMCID: PMC11514949
Review Free full text in Europe PMC
Engineering dendritic cell biomimetic membrane as a delivery system for tumor targeted therapy.
J Nanobiotechnology, 22(1):663, 27 Oct 2024
Cited by: 0 articles | PMID: 39465376 | PMCID: PMC11520105
Review Free full text in Europe PMC
Go to all (2,268) article citations
Similar Articles
To arrive at the top five similar articles we use a word-weighted algorithm to compare words from the Title and Abstract of each citation.
Cancer Immunotherapy Targets Based on Understanding the T Cell-Inflamed Versus Non-T Cell-Inflamed Tumor Microenvironment.
Adv Exp Med Biol, 1036:19-31, 01 Jan 2017
Cited by: 166 articles | PMID: 29275462 | PMCID: PMC6693322
Review Free full text in Europe PMC
The role of dendritic cells in cancer.
Semin Immunopathol, 39(3):307-316, 16 Sep 2016
Cited by: 54 articles | PMID: 27638181
Review
Nonblocking Monoclonal Antibody Targeting Soluble MIC Revamps Endogenous Innate and Adaptive Antitumor Responses and Eliminates Primary and Metastatic Tumors.
Clin Cancer Res, 21(21):4819-4830, 23 Jun 2015
Cited by: 32 articles | PMID: 26106076 | PMCID: PMC4631684
The Dual Roles of Human γδ T Cells: Anti-Tumor or Tumor-Promoting.
Front Immunol, 11:619954, 16 Feb 2021
Cited by: 51 articles | PMID: 33664732 | PMCID: PMC7921733
Review Free full text in Europe PMC
Funding
Funders who supported this work.
NCI NIH HHS (3)
Grant ID: R01 CA161005
Grant ID: R01 CA118153
Grant ID: R01 CA127475