Abstract
Free full text

Structural basis for Spt5-mediated recruitment of the Paf1 complex to chromatin
Associated Data
Significance
The polymerase associated factor 1 complex (Paf1C) is an RNA polymerase (pol) II accessory factor that broadly influences gene expression by regulating chromatin structure and the recruitment of RNA-processing factors during transcription. This study shows how phosphorylation of a repeated motif within an additional factor, Spt5, is recognized and used by the Plus3 domain within the Paf1C subunit Rtf1 (restores TBP function 1) to promote recruitment of Paf1C to the transcription machinery. Deletions of both the Rtf1 Plus3 domain and the C domain of Cdc73 (Cell Division Cycle 73) are required to abolish Paf1C-mediated histone modifications and chromatin occupancy suggesting that dual attachment points facilitate the association of Paf1C with RNA pol II.
Abstract
Polymerase associated factor 1 complex (Paf1C) broadly influences gene expression by regulating chromatin structure and the recruitment of RNA-processing factors during transcription elongation. The Plus3 domain of the Rtf1 subunit mediates Paf1C recruitment to genes by binding a repeating domain within the elongation factor Spt5 (suppressor of Ty). Here we provide a molecular description of this interaction by reporting the structure of human Rtf1 Plus3 in complex with a phosphorylated Spt5 repeat. We find that Spt5 binding is mediated by an extended surface containing phosphothreonine recognition and hydrophobic interfaces that interact with residues outside the Spt5 motif. Changes within these interfaces diminish binding of Spt5 in vitro and chromatin localization of Rtf1 in vivo. The structure reveals the basis for recognition of the repeat motif of Spt5, a key player in the recruitment of gene regulatory factors to RNA polymerase II.
Gene expression in eukaryotes uses numerous accessory factors to aid progression of RNA polymerase (pol) II through the transcription cycle and regulate transcript levels. Additionally, some of these factors, including the polymerase associated factor 1 complex (Paf1C), influence gene expression through their ability to modify chromatin structure or regulate RNA processing (1). Originally identified in yeast during a search for RNA pol II-associated factors (2), Paf1C is conserved throughout eukaryotes and is minimally composed of the subunits Paf1, Ctr9, Cdc73, Rtf1, and Leo1 (3–5). Human Paf1C contains an additional subunit, Ski8 (6), which also has an independent role in regulating exosome-mediated mRNA degradation (7). Paf1C colocalizes with RNA pol II from the promoter to the 3′ end of genes (8, 9) and mediates a diverse set of events, including transcription-coupled histone modifications (10–13), phosphorylation of the RNA pol II C-terminal domain (CTD) (14, 15), recruitment of termination and RNA 3′-end–processing factors (15–17), and maintenance of chromatin during transcription (18). Proper transcription-coupled ubiquitylation of histone H2B at lysine 123 in yeast is dependent on Paf1C recruitment to chromatin (19). Phenotypes caused by mutations that dissociate Paf1C from active genes argue that the ability of Paf1C to carry out its diverse set of activities in a controlled manner requires proper recruitment of Paf1C to actively transcribing RNA pol II (19–22).
Several Paf1C-interacting factors have been shown to play a role in the recruitment of Paf1C to transcribed genes. In humans, Paf1C promotes transcription elongation through direct interactions with RNA pol II as well as the elongation factor SII/TFIIS (23). Paf1C also copurifies with the elongation factor complex Spt4-Spt5 and the histone chaperone Spt16-Pob3/facilitates chromatin transcription (FACT) (3–5, 24), all of which travel with the elongating polymerase and could thus be mediating Paf1C recruitment. Spt5 is of particular note because it is the only RNA pol II-associated factor known to be conserved in all three kingdoms of life (NusG in bacteria, RpoE in archaea) (25). Outside of bacteria, Spt5 is found in complex with the small zinc-binding protein Spt4 (26), which stabilizes Spt5 and improves Pol II processivity (27). Recruitment of Spt4-Spt5 to polymerase is mediated by a direct interaction between the Pol II clamp domain and the Spt5 NGN domain (25). Similar to the RNA pol II CTD, Spt5 contains a series of short repeating sequence motifs at or near its C terminus, known as C-terminal repeats (CTR). Also similar to the RNA pol II CTD, the Spt5 CTR has been proposed to serve as a scaffold for the recruitment of elongation factors, such as TFIIF, FACT, Paf1C (5, 28–30), RNA 3′-end–processing factors (31), and mRNA-capping enzymes (32). Phosphorylation of the Spt5 CTR by the kinase Bur1/Cdk9 (29, 33–35) regulates its interactions with these factors.
The subunits Rtf1, Cdc73, and Leo1 have all been shown to play a role in recruitment of Paf1C to chromatin at active genes (14, 15, 22, 36). In Cdc73, a highly conserved C-terminal domain containing a Ras-like fold mediates binding to both phosphorylated CTR and CTD peptides in vitro and facilitates chromatin occupancy in vivo (20, 21). Within Rtf1, a central region called the ORF-Association Region (OAR) was shown to be required for recruitment of Paf1C to chromatin (22). The OAR, defined through a series of internal deletions within Rtf1, is a conserved domain also named the Plus3 domain after the presence of three invariant positively charged amino acids. NMR structural analysis and chemical perturbation studies revealed that the Plus3 domain adopts a Tudor domain fold and identified residues on the Plus3 surface required for interactions with DNA substrates that mimic the transcription bubble (37). A direct role for the Plus3 domain in recruitment of Paf1C to chromatin had remained elusive until a recent report demonstrating that the yeast Plus3 domain is both necessary and sufficient for binding to the C-terminal repeats of Spt5 (19). Further, deletion of the Spt5 CTR or loss of Bur1 activity impairs recruitment of full-length Rtf1 (21, 29, 30, 38, 39) and the isolated Plus3 domain to chromatin (19), demonstrating that modification of the Spt5 CTR is crucial for recruitment of Paf1 to active genes.
Here we provide structural insight into how the Spt5 CTR mediates recruitment of a transcription elongation factor to RNA pol II through the structure of the Plus3 domain from human Rtf1 in complex with a phosphorylated Spt5 CTR motif. The structure reveals the basis for recognition of the CTR motif as well as the molecular basis for phosphate-specific recognition. Peptide binding is driven by an extended interface with two parts, one that discriminates phosphorylation at the T4 position within a CTR repeat whereas a separate, hydrophobic surface interacts with position P5 and the Spt5 sequence between canonical CTR repeats. Both interaction surfaces observed in the Plus3–Spt5 crystal structure are critical for CTR peptide binding in vitro and together contribute to Paf1C recruitment to chromatin in yeast.
Results
A Distinct Conserved Surface Distinguishes the Plus3 Domain from Other Tudor-Containing Proteins.
To elucidate the mechanism by which the Plus3 domain of Rtf1 recognizes Spt5 and mediates recruitment of Paf1C, we crystallized and determined the structure of the Plus3 domain from human Rtf1 (residues 353–484), refining to an Rfree value of 24.0% against data to 2.12-Å resolution (see Table S1 and SI Materials and Methods for a complete description of the structure determination process). The Plus3 domain crystallized with two structurally similar monomers in the asymmetric unit (0.6-Å rmsd over 125 Cα atoms). Overall the fold is similar to that observed using NMR (1.0-Å rmsd over 123 pairs of Cα atoms) (37). Comparison with the structural database identified similarity between the β-sheet core of the Plus3 and members of the Tudor superfamily including Tudor, MBT, chromo-, PWWP, and the related PAZ domains (37). Tudor domains are best known as methyl-arginine and methyl-lysine–binding domains that play roles in a broad range of biological functions including histone modifications (40), chromatin remodeling (41), and RNA maturation (42). Methyl recognition is accomplished through a cage of two to four aromatic residues, typically contained within the Tudor domain itself, with enhanced peptide specificity achieved through neighboring sequences in Tandem (43) or Extended (44) Tudor domains. Although adopting a similar fold, the aromatic residues required for methyl recognition are not conserved in the Rtf1 Plus3 domain (Fig. 1A and Fig. S1), suggesting it is unlikely to bind these substrates.
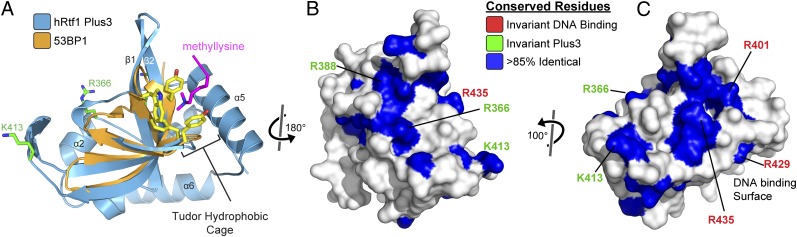
Sequence conservation on the Rtf1 Plus3 domain surface. (A) Structural overlap of the Rtf1 Plus3 domain (blue) with a Tudor-histone H4 methyllysine complex from p53-binding protein 1 (53BP1) (orange; PDB ID 2IG0) (43). The second Tudor fold from 53BP1 is not making contacts with the methyllysine and has been removed for clarity. Aromatic cage residues (yellow) in 53BP1 are indicated. (B and C) Surface representation of the Rtf1 Plus3 domain with residue positions that contain >85% sequence identity in an alignment of Rtf1 sequences colored blue. Residues involved in DNA recognition are labeled (red). The invariant Plus3 residues are labeled green.
We mapped sequence conservation onto the surface of the Rtf1 Plus3 domain from an alignment of nine Rtf1 sequences (Fig. 1 B and C). A surface on Rtf1 implicated in the recognition of DNA substrates that mimic the transcription bubble has been reported (37). This surface is located near the methyl recognition cage in Tudor domains (Fig. 1C) and contains a number of residues that are conserved within Rtf1. Our conservation mapping also identified an additional conserved surface on Rtf1, which contains two of the three Plus3 residues (R366 and R388). This surface is distinct from any known Rtf1 interaction surface, and we hypothesized that it may represent an additional binding site within the Plus3 domain.
Phosphospecific Recognition of a Spt5 CTR Motif.
The transcription elongation factor Spt5 exists as a dimer with Spt4 in a complex known as DSIF in humans and contains between one and fifteen CTR repeats. The CTR motifs can be phosphorylated by the kinase Bur1/Cdk9 (24, 29, 30), which was shown to enhance recruitment of Paf1C to chromatin (19, 21, 29, 30, 38, 39) and RNA pol II processivity during transcription elongation (24, 35). Recent biochemical and in vivo evidence has demonstrated a direct interaction between the Rtf1 Plus3 domain and the CTR of Spt5 in yeast (19). We sought to characterize the human Plus3–Spt5 interaction and determine whether this interaction was phosphospecific. To clarify the interpretation of biochemical results and provide a unique binding register for our structural work, we synthesized two Spt5 peptides corresponding to residues 779–790 of human Spt5. These peptides contain one complete CTR repeat including its spacer and were constructed either with or without a phosphothreonine at position T784 (Fig. 2A). We labeled both peptides with 6-carboxyfluorescein (CF) at their N terminus and monitored binding of recombinant human Rtf1 Plus3 domain to these peptides using fluorescence anisotropy (Fig. 2B). We found that the Plus3 domain binds the phosphorylated form of the Spt5 CTR (pCTR) with a calculated equilibrium dissociation constant (Kd) of 5.45 ± 0.38 µM (Fig. 2B). Binding to unphosphorylated peptide resulted in a negligible change in anisotropy, with an estimated Kd greater than 1 mM. These data suggest that the Plus3 domain can recognize and directly bind a single phosphorylated repeat of the Spt5 CTR and strongly suggest Paf1C recruitment to RNA pol II is mediated through a direct interaction with Spt5.
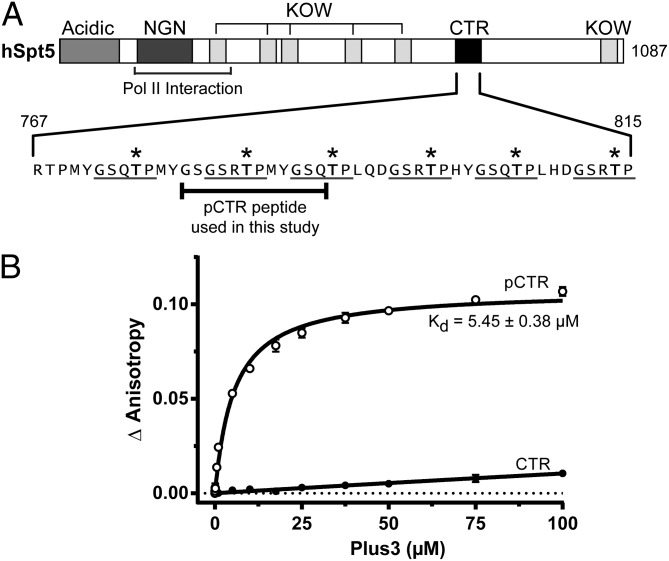
Human Rtf1 Plus3 domain recognizes phosphorylated Spt5 CTR. (A) Domain architecture of human Spt5 including acidic, NusG-N-terminal (NGN) domain, KOW (Kyprides, Ouzounis, Woese) domains, and the C-terminal repeat (CTR) regions. Spt5 sequence (767–815) containing the CTR repeats is expanded, with pentapeptide CTR motifs underlined and phosphocapable threonine residues indicated with an asterisk. (B) Increasing concentrations of purified Plus3 were titrated into 20 nM of fluorescein-labeled pCTR or CTR peptide, and binding was assessed via fluorescence anisotropy. Binding measurements were performed in triplicate, and the SD was plotted. The Kd was extrapolated using nonlinear regression.
Structure of the Plus3–Spt5 pCTR Complex.
To determine the molecular basis for phosphospecific recognition of a Spt5 CTR repeat by the Rtf1 Plus3 domain, we crystallized and determined the structure of a Plus3–pCTR complex (Fig. 3A). Phases were determined using molecular replacement and the Plus3 structure as a search model. The final model was refined at 2.43-Å resolution with Rwork and Rfree values of 17.7 and 22.4%, respectively. Six Plus3 molecules are contained within the asymmetric unit, four of which are in complex with a CTR motif (Fig. 3A). For clarity we are describing the complex that is best resolved and whose model is most complete; however, all four complexes adopt a similar conformation (maximum rmsd of 0.8 Å over 131 Cα atoms). The two remaining Plus3 proteins are making crystal contacts that occlude the peptide binding. The conformation of the Plus3 domain is not significantly altered as a result of peptide binding, with an rmsd of 0.8 Å over 133 Cα atoms.
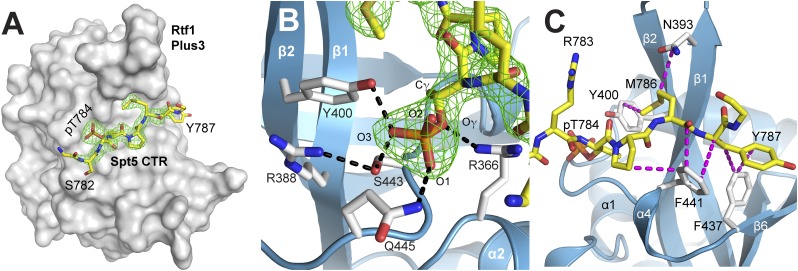
Rtf1 Plus3 uses an extended surface to recognize the Spt5 pCTR motif. (A) Surface view of the Plus3 domain (white) in complex with the Spt5 pCTR peptide (yellow). Omit map density (Fo-Fc, contoured at 4.0 σ, green) is shown for the peptide. The side chain of Spt5 R783 has been removed for clarity. (B) The phosphothreonine recognition pocket. The Spt5 pCTR peptide is shown in yellow with omit map density (Fo-Fc contoured at 4.0 σ) in green. Critical Plus3 residues involved in peptide recognition (white sticks) and the hydrogen bonds involved in recognition (black) are indicated. (C) The hydrophobic Spt5 pCTR-binding surface. Van der Waal interactions (magenta) between Plus3 residues (white) and the pCTR peptide (yellow) are shown. Spt5 residues pT784 and P785 are contained within the CTR motif, whereas residues M786 and Y787 are found within the spacer between CTR repeats and are making significant contacts with the Rtf1 Plus3 domain. Contacts between M786 and Plus3 residues G390 and G392 have been omitted for clarity.
The CTR peptide lies across a shallow groove formed by the β1-β2 central hairpin and the loop connecting β6 and α5 (Fig. 3A), burying 443 Å2 of surface area on the Plus3 domain. The Plus3–Spt5 interface is distant from the location of methyl binding in Tudor domains and largely overlaps the conserved surface observed earlier (Fig. 1B). Interactions between the Plus3 and the Spt5 pCTR peptide can be divided into two sections, a phosphothreonine recognition interface and a hydrophobic peptide-binding interface. Recognition of the CTR phosphothreonine (pT784) is accomplished through a series of hydrogen-bonding interactions that form a cage around the phosphate. Two of the conserved positively charged residues from which the Plus3 domain derives its name are found in this interface. One of these, R366, makes a hydrogen bond with the gamma oxygen of pT784, orienting the position of the threonine residue within the binding pocket (Fig. 3B). The positioning of pT784 is also conferred by hydrophobic interactions between the gamma carbon of the pT784 residue and the aromatic ring of Y400 and the Cα positions of S443 and F441. The other canonical Plus3 residue in this interface, R388, interacts with and likely orients the side chains of Y400 and S443, both of which are making direct hydrogen bonds to the O3 position of the phosphate moiety (Fig. 3B). Combined with residue Q445, which is making a direct hydrogen bond to the O1 oxygen, this network of hydrogen-bonding interactions recognizes three of the four oxygens within the pT784, providing a mechanism for phosphospecific recognition by the Plus3 domain.
The second portion of the Plus3–pCTR interface is hydrophobic, and unexpectedly, we found it primarily contained Spt5 residues from the spacer region between repeats (Figs. 2A and and3C).3C). The size of this spacer in humans is often conserved and typically contains residues with hydrophobic character (Fig. S2). In the complex we crystallized, Plus3 residues Y400, P398, N393, G390, and G392 combine to form a small pocket that accommodates Spt5 spacer residue M786. The first spacer position is occupied by a large hydrophobic residue such as Met, His, or Leu, any of which could be accommodated within this pocket. The second spacer position, Y787 in our peptide, is interacting through its aromatic ring with a hydrophobic surface on Rtf1 formed by residues F441, F437, and I391. Although the tyrosine in our peptide is the consensus residue at this position, it seems quite reasonable that the Plus3 surface could accommodate glutamine and histidine residues which are also found in this position. Outside of the phosphothreonine recognition surface, the central organizing residue on the Plus3 surface is F441. This residue makes van der Waals contacts with Spt5 residues P785, M786, and Y787 (Fig. 3C). Interactions with P785 are with the Cβ and Cγ positions explaining its preference for this cyclic residue, whereas interactions with M786 and Y787 are largely through the peptide backbone allowing for some flexibility in CTR sequence recognition. Together the structure of the Rtf1 Plus3 domain in complex with a pCTR peptide reveals the basis for phosphothreonine-specific recognition of Spt5, whereas interactions outside of pT784 can accommodate the observed variation in CTR sequence.
Identification of Critical Residues Within the Plus3 Domain That Mediate pCTR Binding.
The binding interface observed in our Plus3–pCTR complex is separate from both the proposed DNA-binding surface identified by NMR chemical perturbation studies (37) and the canonical methyllysine-binding site in Tudor domains (43), suggesting the Plus3 domain has evolved a separate binding surface specifically to interact with Spt5 (Fig. 1B). We next tested the importance of residues at the Plus3–pCTR interface for peptide binding in solution. Guided by our structural data, we used site-directed mutagenesis to alter amino acids that lie on the Plus3 surface, avoiding residues that may play a significant role in conferring protein stability. We tested the ability of purified WT and mutant Plus3 domains to interact with pCTR peptide using differential scanning fluorimetry (45, 46). In this assay, a hydrophobic fluorescent dye is used to monitor the change in melting temperature (ΔTm) conferred by the addition of ligand. We observe a ΔTm of 1.5 °C when using WT Plus3 and pCTR peptide compared with a ΔTm of 0.5 °C when using WT Plus3 and an unphosphorylated Spt5 peptide (Fig. 4A). In the absence of peptide, the thermal denaturation profiles of Plus3 variants were similar to WT, indicating that overall stability was unaffected for any of the proteins tested (Fig. S3). A R366A substitution, however, abolished pCTR binding, suggesting an important role in peptide binding for this residue. This role may be to orient the side chain of Spt5 T784, thereby positioning the attached phosphate group into the hydrogen-bonding cage and suggesting why the effect of a R366A mutant was stronger than that of Q445A which is also hydrogen-bonding to pT784 (Fig. 4A). Alanine substitutions within the hydrophobic interface at positions F437 and F441 significantly compromised peptide binding, whereas a R435A variant had a subtle effect, all consistent with their role in peptide recognition observed in the structure. Substitutions in conserved residues K413 and F440 located outside the binding interface had no effect (Fig. 4A and Fig. S3), further validating predictions based on the structure.
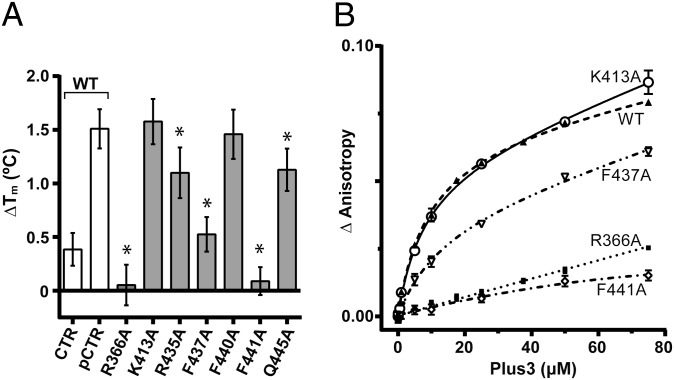
Substitutions of residues at the Plus3–pCTR interface affect peptide binding. (A) Increase in the unfolding temperature for Plus3 upon the addition of CTR peptide as measured by differential scanning fluorimetry. White bar indicate measurements performed using WT Plus3 protein with the indicated peptide, gray bars used the indicated Plus3 variant with pCTR peptide. Asterisks denote P values of <0.05. Error bars represent SD from 12 replicates. (B) Binding isotherms for human Plus3 or the indicated Plus3 variant to 6-CF-labeled pCTR peptide. Increasing concentrations of purified Plus3 variants were titrated into 20 nM of pCTR peptide, and binding was assessed via fluorescence anisotropy. Experiments were performed in triplicate, and the SD was plotted.
We used fluorescence anisotropy to test the importance of residues at the Plus3–pCTR interface using an alternative approach. R366A and F441A substitutions both severely reduced pCTR binding (Fig. 4B), in agreement with the results from differential scanning fluorimetry. These results suggest that both hydrogen bonding and hydrophobic interactions are critical for proper pCTR binding. Titrations using a F437A variant of Plus3 resulted in reduced affinity for pCTR, consistent with an important but not critical role in peptide recognition. Together, these data demonstrate that the pCTR-binding surface observed in the crystal is also being used for peptide binding in solution. Further it demonstrates that both the phosphothreonine recognition cage and the hydrophobic binding interface centered on F441 are critical determinants for Spt5 binding.
Rtf1 Plus3 Residues Are Important for Proper Chromatin Association.
Our structural and biochemical studies identified amino acids R366 and F441 of human Rtf1 as being critical for the Plus3–pCTR interaction in vitro. To test if these residues are important for the recruitment of Rtf1 to chromatin in vivo, we performed chromatin immunoprecipitation assays in Saccharomyces cerevisiae where roles for the Rtf1 Plus3, the Spt5 CTR, and the Bur1-Bur2 kinase in Paf1C recruitment have been well established (19, 21, 29, 30, 38, 39). Individually, alanine substitutions for S. cerevisiae Rtf1 residues R251 and Y327, which correspond to human Rtf1 residues R366 and F441, respectively, caused a modest decrease in Rtf1 occupancy on two active genes, PYK1 and PMA1, with the R251A substitution having the stronger effect (Fig. 5A). Interestingly, when both the R251A and Y327A substitutions were incorporated into Rtf1, an approximately additive decrease in chromatin occupancy of Rtf1 was observed. Consistent with the localization of the corresponding human Rtf1 residues on the surface of the Plus3 (Fig. 3), individual and simultaneous substitution of R251 and Y327 did not affect the stability of yeast Rtf1 (Fig. 5B). These results indicate that key residues on the Plus3–pCTR interface identified through structural analysis are relevant to recruitment of Rtf1 in vivo and support the idea that the phosphothreonine recognition cage and the hydrophobic binding interface make important individual contributions to the Plus3–pCTR interaction.
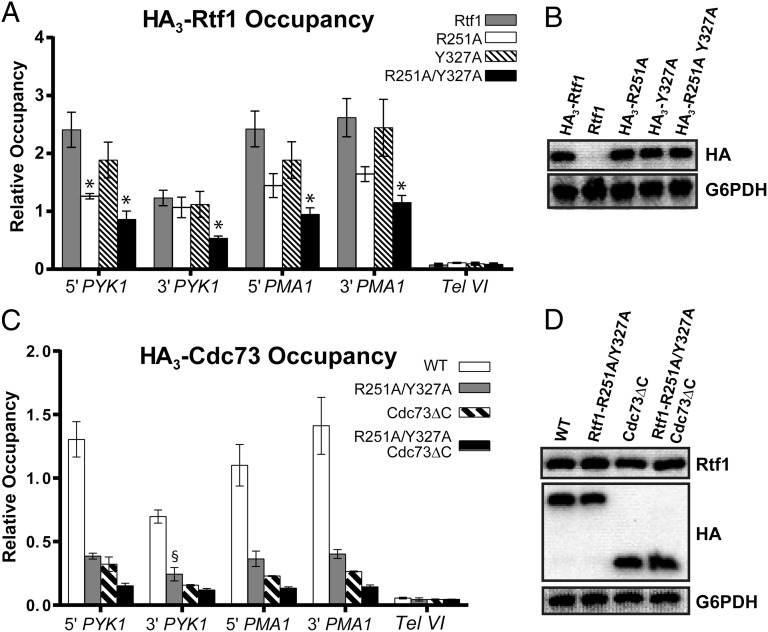
Substitutions at the Plus3–pCTR interface affect recruitment of Paf1C to chromatin. (A) ChIP analysis was performed on transformants of an rtf1 strain (KY619) containing plasmids expressing the indicated hemagglutinin (HA)-tagged derivatives of Rtf1. Error bars represent the SEM from three biological replicates, and asterisks denote P values of <0.05. (B) Western blot analysis of extracts prepared from yeast transformants used in A, using α-HA antibody to determine the levels of Rtf1 protein. Extract made from transformants of KY619 containing a plasmid expressing untagged Rtf1 (pLS20) serves as a negative control for the HA antibody. G6PDH levels serve as the loading control. (C) ChIP analysis of HA3-Cdc73 or HA3-Cdc73
C was performed on transformants of an rtf1Δcdc73Δ (KY2417) strain, containing plasmids expressing the indicated variants of Rtf1 and Cdc73. The error bars indicate the SEM. Chromatin occupancy for the single mutants are significantly different from both WT and the Rtf1-R251A/Y327A, Cdc73
C double mutant (P < 0.05), except where noted (§). ChIP analysis of an untagged control strain showed negligible levels of occupancy. (D) Western analysis of the extracts of strains used in C, using α-Rtf1 and α-HA to determine the Rtf1 and Cdc73 protein levels and α-G6PDH as the loading control.
Previous studies demonstrated that the carboxyl-terminal domain of Cdc73 (Cdc73 C domain) and the Plus3 domain of Rtf1 are both important for recruitment of Paf1C to chromatin (19–22), raising the possibility that the Cdc73 C domain and the Rtf1 Plus3–Spt5 CTR interaction surface function redundantly, at least in part, to tether Paf1C to elongating RNA pol II. To test this idea, we constructed yeast strains that contain a cdc73 C-domain deletion mutation, the rtf1-R251A, Y327A Plus3 domain mutation, or both mutations. ChIP analysis of Cdc73 occupancy, detected through an HA tag on Cdc73 or Cdc73C, revealed that simultaneous removal of the Cdc73 C domain and the Spt5 CTR interacting residues within the Plus3 domain led to a greater defect in Paf1C chromatin localization than either single mutant condition (Fig. 5C). Western analysis demonstrated that the mutant proteins were present at WT levels within the cells (Fig. 5D). Deletion of the Rtf1 Plus3 domain resulted in a pronounced reduction in H3 K4 trimethylation and H3 K79 di/trimethylation levels (Fig. S4). Histone H3 K36 trimethylation and H3 K4 dimethylation decreased modestly in this strain, whereas deletion of the C domain from Cdc73 resulted in only a modest change for all of the histone modifications that we tested. Importantly, these Paf1C-mediated histone modifications were nearly abolished in the absence of both domains (Fig. S4). These data further support the importance of the Plus3–Spt5 CTR interface in Paf1C recruitment and indicate that the association of Paf1C with RNA pol II is facilitated by dual attachment points.
Discussion
The universally conserved transcription elongation factor Spt4-Spt5 plays an important role in the recruitment of transcription elongation and RNA-processing factors to RNA pol II. The recruitment of these factors is regulated by Bur1/Cdk9-mediated phosphorylation of short repeated CTR motifs in Spt5 (28, 31). The CTR could then serve as a scaffold for the phosphorylation-dependent recruitment of factors to transcribed genes in a manner analogous to the CTD of RNA pol II. Structural data of RNA pol II CTD peptides in complex with several different interacting proteins (47, 48) have shown a remarkable diversity in the manner in which these proteins recognize specific residues within the CTD motifs. In contrast, the molecular basis for phosphospecific Spt5 CTR motif recognition has remained elusive.
Here we present structural insight into this important region of Spt5 in complex with the Plus3 domain of human Rtf1. Interestingly, CTR recognition is contained within a conserved surface distinct from its putative DNA-binding surface (37). The CTR-binding surface is also distinct from binding surfaces used by its structural homologs, and we believe this represents a variation in peptide recognition by a Tudor domain. Because the Plus3–pCTR interface is separate from the proposed DNA-binding surface, it is possible that the Plus3 domain of Rtf1 could bind DNA and the Spt5 CTR simultaneously. Interactions with DNA could then serve a regulatory role to either enhance or hinder Spt5 binding. A precedent for this type of regulation can be found in the chromodomain of MSL3, which requires recognition of both double-stranded DNA and histone H4 monomethylated on lysine 20 to facilitate chromatin recruitment (49).
The human CTR motif (GS[Q/R]TP) used in this study is also conserved in a diverse range of organisms including mouse, zebrafish, mosquito, Schizosaccharomyces pombe, and arabidopsis (35). The CTR in S. cerevisiae is divergent in sequence but retains similar chemical properties (Fig. S2B). The structural data described here suggest an expansion of the CTR motif to include sequences between traditional repeats. Interestingly, Spt5 residues G782 and S783 do not make significant contacts with the Plus3 domain in our structure; however, these residues are strongly conserved within the CTR motif. One explanation for this apparent discrepancy is that although these two positions are not important for Plus3 binding, they are recognized by other CTR-binding proteins providing evolutionary pressure to avoid changes at those positions within the CTR. Alternatively, the highly flexible GS pair may provide a malleable linkage between CTR motifs. This could be important for factors that may be simultaneously recognizing multiple CTR motifs. Although we cannot rule out this possibility for the Rtf1 Plus3 domain, we note that binding affinity for a single CTR motif already exceeds that of Scaf8, Pcf11, Nrd1, and Rtt103 for tandem CTD repeats (47, 50), suggesting that increased affinity provided by multiple CTR motifs may not be necessary at least for Rtf1 or that cooperative binding interactions between multiple factors play a more prominent role in CTD recognition (51). Last, it is possible that the conserved GS residues allow Spt5 sequences containing multiple CTR motifs to adopt additional structure such as has been observed with phosphorylated CTD peptides (47, 48).
Substitution of residues critical for CTR peptide binding disrupted the recruitment of Rtf1 to chromatin in yeast, demonstrating the importance of this conserved interaction surface and providing a molecular mechanism for CTR-mediated recruitment of Paf1C to chromatin. Studies in humans have demonstrated that Spt5 is capable of interacting with Paf1C without the need of CTR phosphorylation or the CTR itself (24) suggesting that there is an additional contact point within Paf1C for Spt4-Spt5. Future effort will be needed to reveal the details of this interaction. Our results on the Plus3–pCTR interaction shed light on the diverse mechanisms by which eukaryotes coordinate RNA synthesis with important cotranscriptional processes, including histone modification and recruitment of RNA-processing factors.
Materials and Methods
Full details of the methods used, including protein expression, purification, a complete description of the structural methods, fluorescence anisotropy, chromatin immunoprecipitation, westerns, plasmid construction, and yeast strains, are presented in SI Materials and Methods.
Differential scanning fluorimetry assays were performed following a method outlined in ref. 46. Each Plus3 variant was assayed at a final protein concentration of 33 µM in a reaction buffer containing 20 mM Tris pH 8.0, 100 mM NaCl, 5% (vol/vol) glycerol, 1 mM β-mercaptoethanol, and 1.7X SYPRO Orange (Invitrogen, S-6650) in the presence or absence of 100 µM peptide (pCTR or CTR). Protein unfolding was assessed by monitoring SYPRO Orange fluorescence at 570 nm as a function of temperature. Fluorescence data were analyzed using the Protein Thermal Shift Software v1.1 (Applied Biosystems), using the Boltzmann model to calculate the Tm.
Acknowledgments
The authors thank B. Van Houten, B. Tomson, C. MacGillivray, and A. Berman for technical assistance and advice. Funding support was provided by National Institutes of Health Grant R01 GM52593 (to K.M.A.).
Footnotes
The authors declare no conflict of interest.
This article is a PNAS Direct Submission.
Data deposition: The structure factors have been deposited in the Protein Data Bank, www.pdb.org (PDB ID codes 4L1P and 4L1U).
This article contains supporting information online at www.pnas.org/lookup/suppl/10.1073/pnas.1314754110/-/DCSupplemental.
References
Articles from Proceedings of the National Academy of Sciences of the United States of America are provided here courtesy of National Academy of Sciences
Full text links
Read article at publisher's site: https://doi.org/10.1073/pnas.1314754110
Read article for free, from open access legal sources, via Unpaywall:
https://www.pnas.org/content/pnas/110/43/17290.full.pdf
Citations & impact
Impact metrics
Citations of article over time
Article citations
Multiple direct and indirect roles of the Paf1 complex in transcription elongation, splicing, and histone modifications.
Cell Rep, 43(9):114730, 07 Sep 2024
Cited by: 0 articles | PMID: 39244754 | PMCID: PMC11498942
The Rtf1/Prf1-dependent histone modification axis counteracts multi-drug resistance in fission yeast.
Life Sci Alliance, 7(6):e202302494, 21 Mar 2024
Cited by: 0 articles | PMID: 38514187 | PMCID: PMC10958104
Mechanisms and Functions of the RNA Polymerase II General Transcription Machinery during the Transcription Cycle.
Biomolecules, 14(2):176, 01 Feb 2024
Cited by: 1 article | PMID: 38397413 | PMCID: PMC10886972
Review Free full text in Europe PMC
Spt5 C-terminal repeat domain phosphorylation and length negatively regulate heterochromatin through distinct mechanisms.
PLoS Genet, 19(11):e1010492, 08 Nov 2023
Cited by: 2 articles | PMID: 37939109 | PMCID: PMC10659198
New roles for elongation factors in RNA polymerase II ubiquitylation and degradation.
Biochim Biophys Acta Gene Regul Mech, 1866(3):194956, 17 Jun 2023
Cited by: 0 articles | PMID: 37331651 | PMCID: PMC10527621
Review Free full text in Europe PMC
Go to all (63) article citations
Data
Data behind the article
This data has been text mined from the article, or deposited into data resources.
BioStudies: supplemental material and supporting data
Protein structures in PDBe (3)
-
(1 citation)
PDBe - 4L1UView structure
-
(1 citation)
PDBe - 2IG0View structure
-
(1 citation)
PDBe - 4L1PView structure
Similar Articles
To arrive at the top five similar articles we use a word-weighted algorithm to compare words from the Title and Abstract of each citation.
The recruitment of the Saccharomyces cerevisiae Paf1 complex to active genes requires a domain of Rtf1 that directly interacts with the Spt4-Spt5 complex.
Mol Cell Biol, 33(16):3259-3273, 17 Jun 2013
Cited by: 42 articles | PMID: 23775116 | PMCID: PMC3753903
The Histone Modification Domain of Paf1 Complex Subunit Rtf1 Directly Stimulates H2B Ubiquitylation through an Interaction with Rad6.
Mol Cell, 64(4):815-825, 10 Nov 2016
Cited by: 58 articles | PMID: 27840029 | PMCID: PMC5131541
Spt5 Phosphorylation and the Rtf1 Plus3 Domain Promote Rtf1 Function through Distinct Mechanisms.
Mol Cell Biol, 40(15):e00150-20, 14 Jul 2020
Cited by: 6 articles | PMID: 32366382 | PMCID: PMC7364042
The Spt4-Spt5 complex: a multi-faceted regulator of transcription elongation.
Biochim Biophys Acta, 1829(1):105-115, 06 Sep 2012
Cited by: 86 articles | PMID: 22982195 | PMCID: PMC3545043
Review Free full text in Europe PMC
Funding
Funders who supported this work.
NIGMS NIH HHS (2)
Grant ID: R01 GM52593
Grant ID: R01 GM052593