Abstract
Free full text

Umbilical cord-derived mesenchymal stem cells regulate thymic epithelial cell development and function in Foxn1−/− mice
Abstract
Thymic microenvironments are essential for the maturation of thymocytes, which can be anatomically compartmentalized into cortical and medullar regions. The absence of the gene encoding the transcription factor forkhead box n1 (Foxn1) causes epithelial differentiation to stall in the precursor stage, resulting in the formation of an abnormal thymus. In this study, we used human umbilical cord-derived mesenchymal stem cells (UC-MSCs) to treat Foxn1−/− mice, and then analyzed the maturation and distribution of thymic epithelial cells in the Foxn1−/− thymic rudiment and the thymopoiesis of this newly developed rudiment. Our data showed a well-organized cortex-medulla architecture and an obvious improvement in the maturation of thymic epithelial cells along with the appearance of UEA-1+MHCIIhi thymic epithelial cells in the rudiment. We further demonstrated improved thymopoiesis and the enhanced export of mature T cells with increased numbers of regulatory T cells into the peripheral blood. Furthermore, we observed that MSCs can engraft into thymic tissue and express many cytokines or proteins, particularly keratinocyte growth factor (KGF) and CD248, which are essential for thymic development. Collectively, our data identified a new mechanism for MSCs, which may provide a proper microenvironment for the reconstitution and functional maturation of the thymus in Foxn1−/− mice. Additionally, we elicited additional insights into the therapeutic efficacy of MSCs in several autoimmune diseases.
Introduction
The thymus is the primary site of T-cell development and plays a critical role in the adaptive immune system, with athymia resulting in profound immunodeficiency.1 The thymus is a heterogeneous epithelial environment where morphologically and phenotypically distinct epithelial compartments support thymocyte development.2 The thymic microenvironment is principally comprised of thymic epithelial cells (TECs) and cells with mesenchymal origin.3 TECs are critical for almost all stages of thymocyte development, and the forkhead box n1 (Foxn1) gene is a key regulator of TEC differentiation.4 With the loss of Foxn1, aging was induced with a reduced number of naive T cells.5 Mesenchyme accounts for the functional development of epithelial cells and the maintenance of epithelial architecture and function.6 It has been demonstrated that the mesenchyme was required for the successful reconstitution of functional thymic tissue.7
Mesenchymal stem cells (MSCs), a heterogeneous subset of stromal stem cells, exist in many fetal and adult tissues and can differentiate into cells of the mesodermal lineage as well as other embryonic lineages.8 MSCs have been used to treat many diseases, such as graft-versus-host disease, systemic lupus erythematosus and rheumatoid arthritis, which are caused, at least in part, by abnormal thymic function and an over-reactive immune system.9,10 The well-known mechanisms of MSC efficacy in the treatment of these diseases include their anti-inflammatory function and immunoregulatory effect on the immune system.11 Furthermore, umbilical cord derived-MSCs (UC-MSCs) can also increase the percentage of regulatory T cells (Treg cells) in the peripheral blood of treated patients with rheumatoid arthritis.12 Because Treg cells are primarily generated in the thymus, we hypothesized that the therapeutic efficacy of MSCs could be mediated by regulating thymic function in patients with those diseases. Compared to other sources, UC-MSCs have well-documented self-renewal and multipotent differentiation properties and possess immunoregulatory capacities.13 We therefore chose UC-MSCs as the source of cells for the current study.
Because the Foxn1 gene acts to regulate the growth and/or differentiation, but not the determination, of thymic epithelial progenitors,4 the absence of the Foxn1 gene results in an inability of the thymic epithelia to attract thymocyte precursors and causes epithelial differentiation to remain at the precursor stage.14 Although the Foxn1−/− mouse is a T-cell immunodeficient mouse that lacks the Foxn1 gene, there is a lymphatic tissue-like thymic rudiment in the thymus region that contains typical thymic lymphocytes surrounded by mesenchymal reticulum cells and groups of epithelial cells.15 In this study, we used Foxn1−/− mice as a model to first investigate the effects of MSCs on epithelial development and the functional maturation of the thymus in an immunodeficient genetic background. Our data demonstrated that the thymic rudiment architecture was reconstructed and that thymic function was improved in Foxn1−/− mice along with an increased expression level of KGF after serial MSC infusion, suggesting that MSC infusion is a potential therapeutic choice for the regeneration and maintenance of thymic function in many clinical conditions with degraded thymic function.
Materials and methods
Animal model
Foxn1−/− mice were obtained from the Chinese Academy of Military Medical Sciences. Mice were maintained on an irradiated sterile diet and autoclaved acidified water under specific pathogen-free condition. The research was conducted in accordance with the internationally accepted principles for laboratory animal use and care as found in the US guidelines (NIH publication #85-23, revised in 1985). Experiments were performed in compliance with the national ethical guidelines for the care and use of laboratory animals. Three-week-old Foxn1−/− mice (male) were randomly divided into the treated group and untreated group. The treated group were injected intraperitoneally with UC-MSCs (2×106 cells/0.5 ml/mouse), twice a week, whereas the untreated group received D-Hanks's solution. Mice were sacrificed at different time points after the initial infusion (6, 8 and 10 weeks, respectively, 12 mice/group).
Isolation and characterization of UC-MSCs
The umbilical cords were obtained from local maternity hospitals with donors' informed consent. Human tissue collection for research was approved by the institutional review board of the Alliancells Institute of Stem Cells and Translational Regenerative Medicine. UC-MSCs were isolated and cultured from umbilical cord tissue by a tissue explant method according to a previous protocol.16 The UC-MSCs were incubated in DMEM-F12 medium containing 10% fetal bovine serum (FBS) in a humidified incubator maintained at 37 °C in a 5% CO2 humidified atmosphere, and once 70%–80% confluence had been reached, the cells were detached with 0.1% trypsin-EDTA.
The phenotype of MSC was analyzed by flow cytometry using the following monoclonal antibodies: CD34-FITC, CD45-PE, HLA-DR-PE, CD90-PE CD73-PE (Becton Dickinson Biosciences, San Jose, CA, USA) and CD105-PE (Tianjin Sungene Biotech, Tianjin, China). A nonspecific IgG1 isotype was used as a control. For CD248 analysis, MSCs were incubated with rabbit anti-human CD248 polyclonal antibody (Abnova, Taipei, Taiwan) for 30 min and then stained with goat anti-rabbit IgG-FITC (Invitrogen) for 30 min. Samples were analyzed using a FACS Calibur flowcytometer (Becton Dickinson Biosciences).
For osteogenic and adipogenic differentiation, MSCs were seeded in 24-well tissue culture plates (Corning Incorporated, Corning City, NY, USA), the culture medium was changed with a StemPro Osteogenesis/Adipogenesis Differentiation Kit (Invitrogen), and the cells were cultured for 2–3 weeks. Osteogenesis and adipogenesis differentiation was analyzed by Alizarin Red-S and Oil-Red-O (Sigma, St Louis, MO, USA), respectively.
Histology and immunofluorescence staining
Freshly removed thymus tissues were embedded in Tissue-Tek OCT compound (Sakura Finetek, Tokyo, Japan), frozen in a freezing microtome (Leica microsystems, NuBloch, Germany) and then cut into 4 µm-thick frozen sections. Serial sections were air dried and fixed in ice-cold acetone for 10 min and were then stained by hematoxylin and eosin staining for histological analysis. Freshly cut frozen sections were fixed in ice-cold acetone for 10 min, then washed using 1× PBS for 5 min three times. Five percent bovine serum albumin (BSA) was used as a blocking buffer, and then the specimens were incubated overnight at 4 °C with the following primary antibodies: anti-mouse Keratin 5 (Covance Research Products, Berkeley, CA, USA), Troma-1 mAb (rat anti-Keratin 8; Rolf kemler, Developmental Studies Hybridoma Bank, University of Iowa, Iowa City, IA, USA), PE ant-mouse CD4 (BD Pharmingen, San Diego, CA, USA) and FITC anti-mouse CD8 (Abcam, Cambridge, MA, USA) as well as biotinylated UEA-1 (Vector Laboratories, Burlingame, CA, USA)with strepavidin-PE (BD). Control slides were incubated with the corresponding isotype antibodies PE-rat IgG1 and FITC-rat IgG1 (BD). After washing, sections were incubated for 30 min with directly conjugated secondary reagents. DAPI (2-(4-Amidinophenyl)-6-indolecarbamidine dihydrochloride) was used in the final step for nuclear staining (Vector Laboratories, Burlingame, CA, USA). Microscopic analysis was performed with an Olympus IX51 microscope (Olympus, Center Valley, PA, USA).
Flow cytometry
Freshly isolated suspension thymocytes, splenocytes or peripheral blood cells (106) were used for each sample. Cells were blocked for 15 min at 4 °C with purified anti-mouse CD16/CD32 (eBioscience, San Diego, CA, USA) and then stained using the following antibodies: PE anti-mouse CD4 (BD), FITC anti-mouse CD8a (BD), FITC anti-mouse CD4 (BD), APC anti-mouse CD25 (BD), PE anti-mouse Foxp3 (eBioscience) and Foxp3 Staining Buffer Set (eBioscience). Cells were analyzed on a flow cytometer (FACS Calibur; BD).
Thymic stromal cells were isolated by digesting thymic lobes with collagenase I (0.25 mg/ml+1 mg/ml)+NB4 (Serva, Heidelberg, Germany) and DNase I (10 µg/ml) for 45 min and were then centrifuged at 1000 r.p.m. for 5 min. Trophoblast stem cell were washed and stained with anti-CD45–APC (BD), anti-MHC class II-FITC (eBioscience), and anti-UEA-1–biotin (Vector Laboratories,) followed by streptavidin-PE (BD) for flow cytometry analysis.
T-cell receptor excision circle (TREC) analysis
Spleens were weighed and frozen at −80 °C for later analysis. Murine Rec-Jα-TRECs were determined using real time PCRas described previously.17 Total DNA was extracted and purified with the DNeasy Blood & Tissue Kit (Qiagen, Milan, Italy). The sequences of the primers are as follows: mRec primer(5′-GGGCACACAGCAGCTGTG-3′), Jαprimer (5′-GCAGGTTTTTGTAAAGGTGCTCA-3′) and mRec-Jα fluorescent probe (5′-FAM-CACAAGCACCTGCACCCTGTGCA-TAMRA-3′) (Invitrogen).18 The PCR reaction contained, 1500 ng DNA, 0.5 µM of each primer, 0.1 µM fluorescent probe and Taqman mix (Roche Applied Science, Penzberg, Upper Bavaria, Germany). Amplifications were performed in triplicate on a LightCycler 2.0 (Roche) at 95 °C for 10 min followed by 40 cycles of 95 °C for 10 s, 60 °C for 35 s, 72 °C for 1 s and a final step at 40 °C for 30 s.
Thymus development-associated cytokine expression in UC-MSCs
Total RNA was extracted using Trizol reagents, and cDNA was synthesized by using ReverTra Ace qPCR Reverse Transcriptase MIX Kit (Toyobo Co., Ltd., Osaka, Janpan).The primers were synthesized by Invitrogen as follows: KGF forward primer: 5′-AAGAACTGCCCTTCCTCA-3′, reverse primer: 5′-AAGTCCTCCTCTGCCCTC-3′ ghrelin19 forward primer: 5′-CCATGCCCTCCCCAGGGACC-3′, reverse primer: 5′-ATCACTTGTCGGCTGGGGCCTC-3′ IL-1520 forward primer: 5′-ATGGGTTCTTGGTCTCAC-3′, reverse primer: 5′-ACTCAAAGCCACGGTAAA-3′ growth hormone-1 forward primer: 5′-CCTACCAGGAGTTTGAAGAA- 3′, reverse primer: 5′-CAGGCTGTTGGCGAAGAC-3′ growth hormone receptor forward primer: 5′-TAGTATTTCCCATTTATCGC-3′, reverse primer: 5′- TGTTGTCAGGCTGTTGTG-3′ and IL-7 forward primer: 5′-CCCCTTGGGATGGATGAA-3′, reverse primer: 5′-ATGATGACCGCAACTGGA-3c. The first-strand cDNA was amplified using PCR in 50 µl reactions with the following program: denaturation for 5 min at 95 °C, followed by 30 amplification cycles of 1 min at 95 °C, 30 s at 55 °C and 30 s at 72 °C, and a 5 min final extension at 72 °C. RT-PCR products were separated on 1.5% agarose gels and visualized by staining with NA-Red (Beyotime Biotechnology, Nantong, JiangSu, China) and photographed using a ChemiDoc XRS System (Bio-Rad, Hercules, CA, USA). The relative mRNA expression of each gene was normalized to β-actin (forward primer: 5′-CCTGGCACCCAGCACAAT-3′, forward primer: 5′-GGGCCGGACTCGTCATAC-3′).
UC-MSCs derived from four different donors were seeded onto Nunc Lab-Tek II Chamber Slide System (ThermoFisher Scientific, Waltham, Massachusetts), 2×104 cells/well. After 24 h, cells and frozen sections of the thymic tissues were fixed with 4% paraformaldehyde, permeabilized with 0.1% Triton X-100 and blocked with 3% normal donkey serum, and were then incubated overnight at 4 °C with goat anti-human KGF/FGF7 antibody (R&D Systems, Minneapolis, MN, USA) at 10 µg/ml. After washing, sections were incubated for 60 min with an Alexa Fluor 488 donkey anti-goat secondary antibody (Invitrogen). DAPI was used in the last step for nuclear staining. Microscopic analysis was performed with a spinning-disk confocal microscope (Nikon Imaging, Tokyo, Japan).
CellTracker CM-DiI-labeled UC-MSCs injection into Foxn1−/− mice
CellTracker CM-DiI (Invitrogen, Carlsbad, CA, USA) was used to label UC-MSCs according to the labeling procedure.21 Foxn1−/− mice were injected intraperitoneally with CM-Dil-labeled UC-MSCs, 2×106 cells/mice. The mice were then killed at 24 h, 72 h and 168 h after injection, respectively. Thymic tissues were taken and immediately frozen to obtain frozen sections. DAPI was used to stain nuclear and microscopic analysis was performed with an Olympus IX51 microscope.
Statistical analysis
Analysis of statistics was carried out with SPSS (version 17.0) software using t-tests. All data are presented as the mean±s.d., and P<0.05 was considered significant.
Results
UC-MSC isolation and characterization
Human MSCs were isolated from umbilical cords and pooled from a group of four individual donors. The cells were cultured and expanded in vitro in the ultimate conditions. At the end of culture, flow cytometric analysis was performed with a panel of surface markers, which confirmed that the cells were positive for CD73, CD90 and CD105, and negative for CD11b, CD45, HLA-DR, CD19 and CD34 (Figure 1a). To further confirm the multipotentiality of UC-MSCs after in vitro culture, we assessed their abilities to differentiate into cells of osteogenic and adipogenic lineages. As previously described, UC-MSCs were cultured in the appropriate inducing media for 2 or 3 weeks, and the lipid vacuoles were stained with Oil Red O in the differentiated adipocytes (Figure 1b), whereas the osteogenic differentiation of MSCs was stained with AlizarinRed (Figure 1c). These data demonstrate that the UC-MSCs maintained their stem cell characteristics after in vitro culture and expansion.
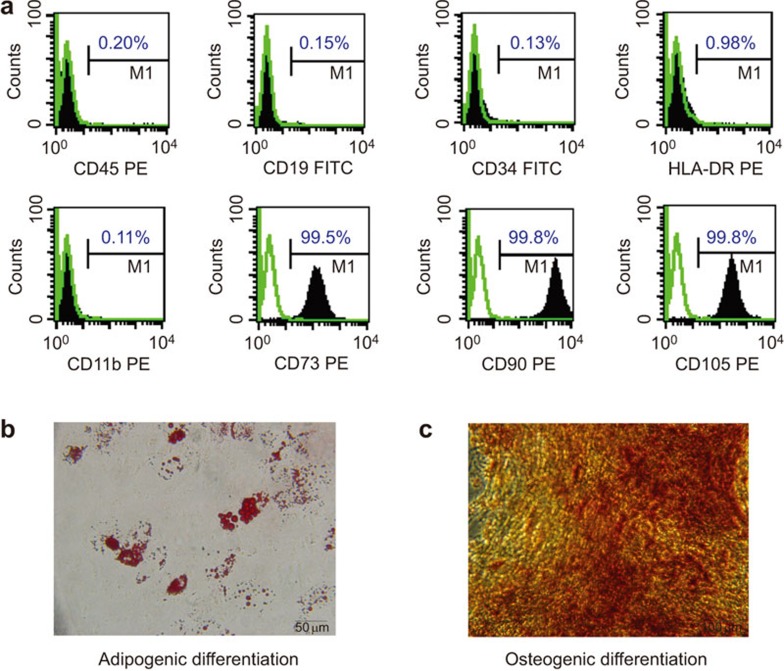
Characterization of UC-MSCs. (a) Flow cytometric analysis of cell surface markers on UC-MSCs. (b) adipogenesis differentiation of UC-MSCs was analyzed by Oil-Red-O staining. (c) Osteogenesis differentiation was analyzed by Alizarin Red-S staining. US-MSC, umbilical cord-derived mesenchymal stem cell.
UC-MSCs promote the enlargement of the thymic rudiment in Foxn1−/− mice
The Foxn1−/−gene mutation results in abnormalities of thymic development, and the thymus-like organ in Foxn1−/− mice is composed of condensed lymphatic-like tissue. The structure is surrounded by a thin fibrous capsule and a poorly demarcated sinusoidal space underneath the capsule with mature adipose tissue on the medial side.15 To study whether MSCs promote thymic development, we analyzed thymic tissue in terms of size and histological morphology with H&E staining in mice from both the treated and untreated groups at different time points. There were no significant differences in the appearances and weights of thyme between the groups. However, H&E staining revealed that a larger area of full normal thymic structure and a more organized corticomedullary architecture was clearly observed in the treated mice compared to the untreated group (Figure 2). These data strongly indicate that MSCs can rebuild the thymic architecture of the Foxn1−/− mice at as early as 6 weeks after their administration and continued to improve after repetitive cell infusion.
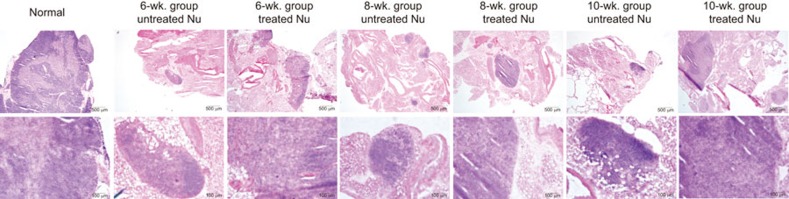
UC-MSCs promote thymic enlargement in Foxn1−/− mice. Hematoxylin and eosin-stained frozen sections of thymi from untreated, treated and normal BALB/c mice at different time points. Untreated nude mice thymi showed completely disorganized CMJs. After UC-MSC administration, thymi were greatly enlarged, and the corticomedullary architecture became organized in the treated thymus. CMJ, corticomedullary junction; US-MSC, umbilical cord-derived mesenchymal stem cell.
UC-MSC administration increases thymopoiesis and enhances thymic T-cell export
Because the thymic architecture clearly improved with time after the UC-MSCs infusions (Figure 2), the effect of UC-MSCs on thymopoietic capacity was further investigated. Although in normal thymic tissue the majority of thymocytes are CD4/CD8 double positive, in Foxn1−/− mice, the double-positive T cells were almost absent due to a lack of interactions between the thymic epithelium and the thymocyte progenitors. After UC-MSC administration, however, the CD4+CD8+ thymocytes expanded with only a few single-positive cells appearing in the medulla (Figure 3a). Additionally, the total thymic cellularity of the treated Foxn1−/− mice increased by 2.5-fold compared to the untreated mice by 8 weeks after UC-MSC administration (Figure 3b). An additional flow cytometric analysis revealed that the absolute numbers of thymic T-cell subtypes also increased significantly after treatment (Figure 3c–e).
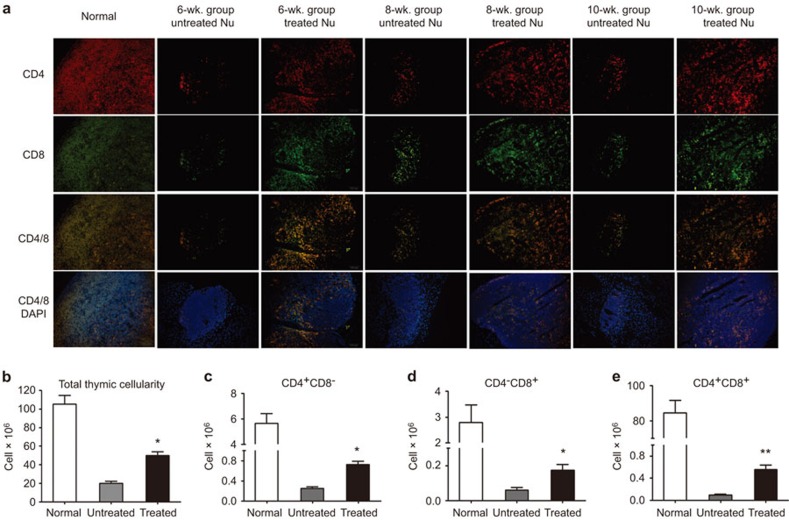
UC-MSCs increase thymocyte numbers in Foxn1−/− mice. (a) The distribution of CD4+ (red) and CD8+ (green) thymocytes in thymi. The orange area represents double-positive cells (CD4+CD8+). Single-positive cells appeared in the medulla. (b–e) Thymi were analyzed by flow cytometry 8 weeks after administration. (b) Total thymocyte numbers (cells×106). (c–e) Total numbers of CD4+CD8−, CD4−CD8+, and CD4+CD8+cells per thymus, respectively. Mean±s.d.; *P<0.05 versus untreated controls, with five mice per group for each time point. US-MSC, umbilical cord-derived mesenchymal stem cell.
TRECs are generated during T-cell receptor gene recombination and can be used as a quantitative measurement of the output of newly generated naive T cells.22 To further evaluate the thymocyte efferent from the newly developed thymi, we analyzed the expression of TRECs in the splenocytes, peripheral blood cells and thymic stromal cells. As shown in Figure 4a–c, the relative expression levels of TRECs in thesplenocytes and peripheral blood cells were significantly increased in the treated Foxn1−/− mice compared to the untreated group, although the TREC expression levels in the thymic stromal cells did not change significantly. These results demonstrated that administration of UC-MSCs could increase thymopoiesis and the emigration of recently generated T cells.
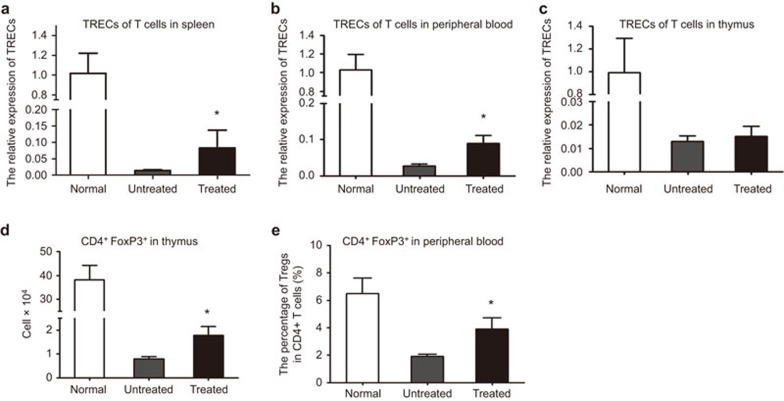
UC-MSCs enhance thymic T-cell export in Foxn1−/− mice. (a–c) Relative expression of TRECs was enumerated after treatment: in spleens (a), in peripheral blood (b) or in thyme (c). There were significantly more TREC+ splenocytes in the 8-week treated group than in the untreated group, 6 mice/group, *P<0.05. (d–e) The percentage of CD4+CD25+Foxp3+ regulatory T cells within CD4+ T cells in the thymi (d) or in the peripheral blood (e). The surface phenotype of cells gated on CD4 expression was analyzed. The percentage of Tregs increased after UC-MSC treatment in the 8-week treatment group, 6 mice/group, *P<0.05. TREC, T-cell receptor excision circle; Treg, regulatory T cells; US-MSC, umbilical cord-derived mesenchymal stem cell.
UC-MSCs increase Treg cells in the thymi and peripheral blood of Foxn1−/− mice
CD4+CD25+Foxp3+ Treg cells, which represent 5%–10% of peripheral CD4+ T cells in healthy adult mice,23 play a key role in maintaining self-tolerance and immune balance by suppressing the effector functions of CD4+ T cells.24 Accumulating data have demonstrated that the deficiency of CD4+CD25+Foxp3+ Treg cells is closely correlated with the development of autoimmune diseases.25 To assess the variation of Treg cells before and after MSC infusion, we analyzed the percentage of Treg cells in the peripheral blood and the absolute numbers of Treg cells in the thymus. As shown in Figure 4d–e, both the percentage of Treg cells in the peripheral blood and the absolute numbers of Treg cells in the thymus were significantly increased in treated Foxn1−/− mice compared to the corresponding untreated group.
The reconstitution of the cortex/medulla and the differentiation of TECs in the Foxn1−/− thymus
Foxn1−/− mice have very small thymi with an abnormal architecture that lacks cortical and medullary domains, and in which most TECs display an intermediate progenitor phenotype.26,27 Keratin 8 (K8) and keratin 5 (K5) are markers of the cortical and medullary areas of TECs, respectively, and can be used to characterize TEC localization and organization. In the normal thymus, medullary TECs form compact medullary compartments (K5+) that are surrounded by K8+ cells in the cortex. In our study, we analyzed thymic organization over periods of 6, 8 or 10 weeks after UC-MSCs infusions. Anti-keratin staining demonstrated that the untreated thymi fromFoxn1−/− mice consisted of clusters of epithelial cells. In contrast, the thymi of the treated groups showed well-organized cortexes (K5−K8+ TECs) and distinct medullary regions (K5+K8− TECs). In addition, in the treated thymi, TECs displayed a dense mesh-like structure in both the cortical and medullary regions, and the structure in the corticomedullary junction was close to normal (Figure 5). This finding indicated that MSCs may promote TEC differentiation and regulate their proliferation to rebuild the thymic architecture of Foxn1−/− mice.
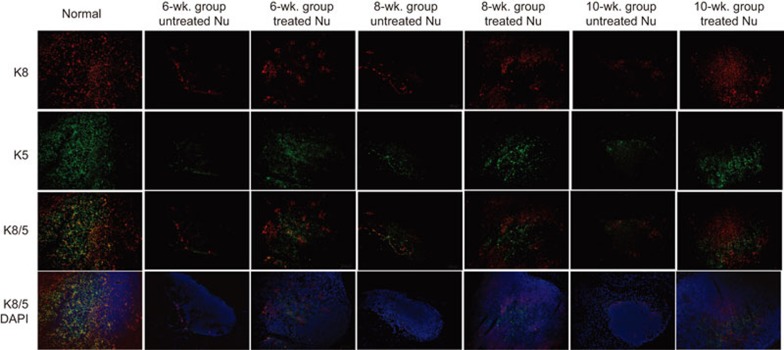
Progressive thymic epithelium cells in the groups. Cryosections were stained for keratin 8 (red) and keratin 5 (green). Scale bar=100 µm. In the 6-week groups, treated thymi showed more keratin 8+ and keratin 5+ thymic epithelial cells than the untreated thymi, but no organization in the cortical or medullary regions was observed. In the 8-week groups, treated thymi revealed a tendency towards an organized corticomedullary architecture. In the 10-week groups, treated thymi display an organized corticomedullary architecture and a corticomedullary junction similar to normal thymi.
UC-MSCs lead to the maturation of medullary epithelial cells in Foxn1−/− mice
Because UEA-1+ TECs, which represent the mature medullary TEC subset, were reported to be associated with medullary compartment organization,28 and UEA-1+ TECs never developed in Foxn1−/− mice,27 we further analyzed the expression of UEA-1+ TEC cells to assess whether UC-MSCs altered the thymic microenvironment at the cellular level. Well-organized UEA-1+ cells appeared in the medulla of the treated thymi that appeared similar to normal thymi, whereas only a few UEA-1+ cells were observed scattered in the untreated Foxn1−/−thymus (Figure 6a). Because MHC class IIis regulated by Foxn1andrepresents functional maturation for TECs,29 we further analyzed two stromal cell subsets defined as UEA-1+MHCIIhi and UEA-1+MHCIIlowcells. Although a previous study demonstrated that Foxn1−/− stromal cells were MHC class II-negative/low,30 in our study, both subsets of UEA-1+MHCIIlow and UEA-1+MHCIIhi cells were detected by flow cytometry after MSC infusions (Figure 6b). Taken together, those data highly suggested that MSC administration may affect the differentiation of epithelial progenitor cells leading to an expansion of both mature and immature TECs.
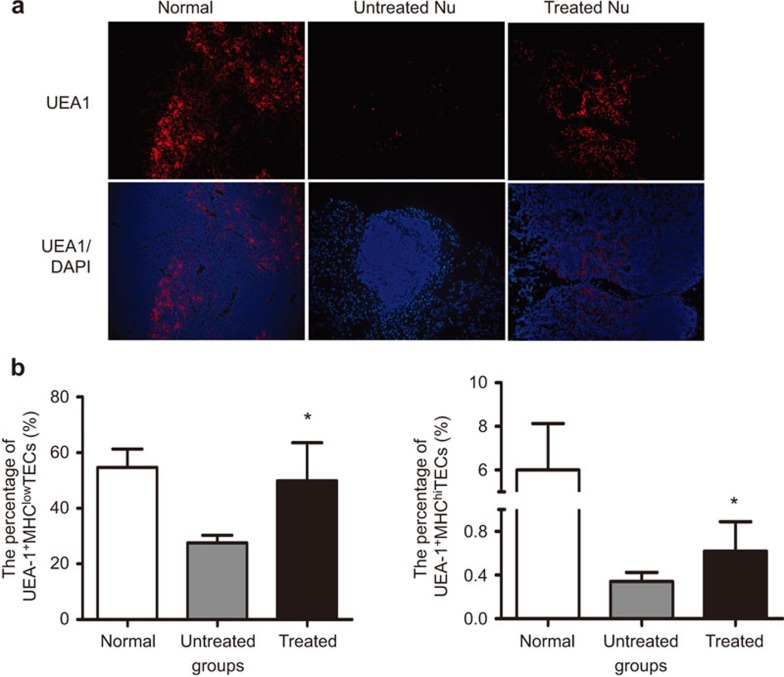
UC-MSCs increase UEA-1+ TECs in Foxn1−/− mice. (a) Frozen thymic sections were stained with DAPI (blue) and UEA-1 (red). Scale bar=100 µm. The treated thymus has more UEA-1+ TECs within medullary regions than that of the untreated 6-week group. (b) Summary of the percentage of UEA-1+MHClo and UEA-1+MHChi TECs within CD45− stromal cells analyzed by flow cytometry. Gated CD45− stromal cells were analyzed and revealed that both subpopulations significantly increased in the treated thymi compared to the untreated thymi at 8 weeks post UC-MSC administration (6 mice/group), *P<0.05. DAPI, 2-(4-Amidinophenyl)-6-indolecarbamidine dihydrochloride; TEC, thymic epithelial cell; US-MSC, umbilical cord-derived mesenchymal stem cell.
Expression of cytokines and growth factors in UC-MSCs
It was well documented that MSCs can extravasate from the blood vessels and spread into many tissues after intravenous administration31 mediated by the adhesion molecules expressed on their surface.8 In our study, we used CM-Dil-labeled UC-MSCs to visualize their distribution in the thymus, demonstrating that UC-MSCs could migrate to and engraft in the thymus and that they were mainly found at the rim of the thymus with some scattering into the center for at least 1 week (Figure 7a). Flow cytometric and immunofluorescence analysis revealed that UC-MSCs express CD248, which may be required for optimal postnatal thymic growth and regeneration following infection-dependent thymic atrophy32 (Figure 7b). Additionally, using an RT-PCR assay we found that UC-MSCs express high levels of various cytokines and growth factors that are considered to be critical for thymus development, including KGF, ghrelin and IL-15 (Figure 7c). The concentration of KGF in cell culture supernatants was 202 pg/ml. As shown in Figure 7d, KGF was expressed in the cytoplasm of almost all UC-MSCs. After infusion, there were human KGF+ MSCs detected in the treated thymus. These results indicate that the application of UC-MSCs resulted in an improved stromal microenvironment, which contained the cytokines necessary for the further development of the thymic rudiment in Foxn1−/− mice.
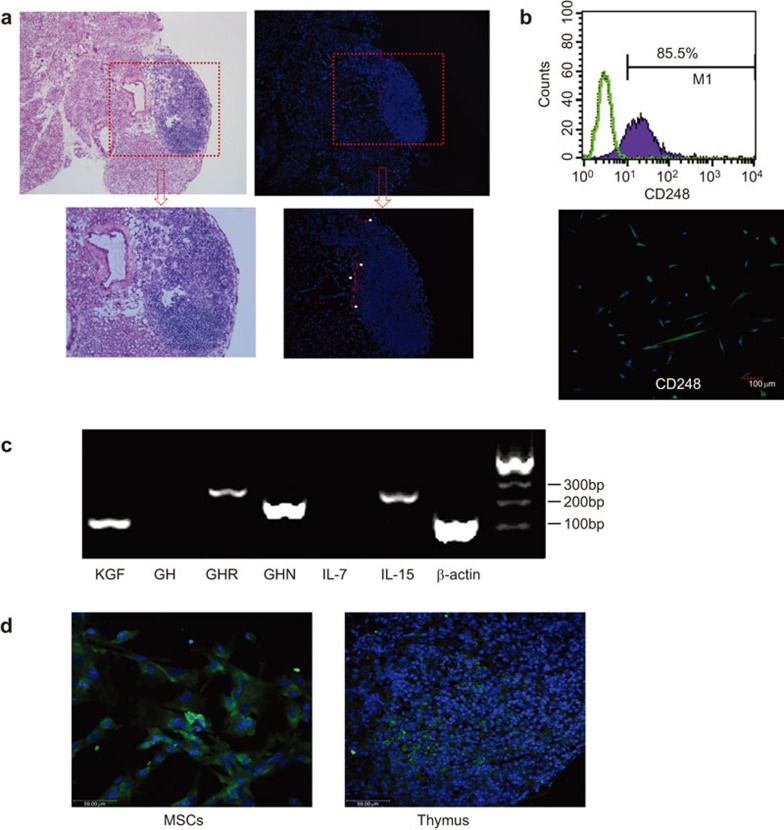
UC-MSCs can engraft into the thymus and express several proteins, which may enhance thymic development. (a) CM-Dil-labeled UC-MSCs migrated and engrafted into the thymi and were found primarily at the thymic rim. (b) Flow and immunofluorescence analysis revealed that UC-MSCs expressed CD248, which may be required for optimal post-natal thymic growth and regeneration following infection-dependent thymic atrophy. (c) RT-PCR analysis of the different cytokine mRNA expression levels in UC-MSCs; MSCs express high levels of ghrelin, KGF, GHR and IL-15, and low levels of GH and IL-7. (d) Frozen thymic sections stained with DAPI (blue) and anti-human KGF (green). Foxn1−/− mice were injected with UC-MSCs intraperitoneally, 2×106 cells/mice. After 72 h, thymi were collected and cut into frozen sections. DAPI, 2-(4-Amidinophenyl)-6-indolecarbamidine dihydrochloride; KGF, keratinocyte growth factor; GH, growth hormone; GHR, growth hormone receptor; GHN, ghrelin; US-MSC, umbilical cord-derived mesenchymal stem cell.
Discussion
This study demonstrated that the administration of UC-MSCs restored the thymic architecture of Foxn1−/− mice as shown by the maturation of the medullary epithelial cells and the increased thymopoietic capacity and the correlated export of mature T cells into the periphery. Our data indicated that the possible molecular mechanisms may be via the secretion of various cytokines, especially KGF, by UC-MSCs within the thymus that may modulate the thymic microenvironment.
Foxn1 is a critical regulator of fetal thymic development33 and is required for initial TEC differentiation in the fetal thymus.34 Foxn1 levels, therefore, have significant effects on thymic phenotypes.35 A reduction in Foxn1 expression has been shown to cause thymic compartment degeneration, a loss of specific TEC subsets and reduced T-cell production.35 The deletion of Foxn1 inhibited the development of mature TECs and reduced the number of total thymocytes, which is observed during normal thymic aging.5 In our study, we chose the Foxn1−/− mouse as a model to investigate the biological function of MSCs in T-cell regulation. Although Foxn1−/− mice lack this gene, the thymic rudiment still contains some precursor cells that have been committed to thymic epithelial lineages.4 In our study, we only observed the enlargement of the remaining thymic tissue in Foxn1−/−mice as shown in Figure 2, and within this area, we found a distribution of mature TECs in both the cortical and medullary regions, a normal corticomedullary junction structure, and UEA-1+MHCIIhi TECs, which indicated that UC-MSCs alone could promote the functional maturation of residual thymic epithelial precursors in the Foxn1−/− thymus.
The expression of specific cytokines by stromal cells and the corresponding expression of their cognate receptors on thymocytes results in paracrine interactions between these two cell populations during thymocyte differentiation.36 In this work, we confirmed that UC-MSCs express CD248, KGF, ghrelin, IL-15 and growth hormone. Of these, CD248 expression is required for optimal postnatal thymic growth and regeneration following infection-dependent thymic atrophy.32 Furthermore, KGF, also known as fibroblast growth factor 7, mediates the proliferation and differentiation of epithelial cells, including TECs, which express the KGF receptor FGFR2IIIb.37 From results demonstrating that KGF ameliorated age-related thymic insufficiency by restoring TEC organization and function, we can conclude that KGF administration can protect the thymus from aging and enhance T lymphopoiesis in young and old mice.38 In our results, we demonstrated the engraftment of MSCs in the thymus with KGF expression in situ. Additionally, the chemokines SCF, IL-6 and GM-CSF may promote thymic cell growth and cellular interactions, ghrelin may increase recent thymic emigration,39 and IL-15 may stimulate lymphocyte proliferation.20 Therefore, the underlying mechanism by which UC-MSCs promote T cell growth and the restoration of thymic architecture may be the expression of these cytokines, which are essential for thymic development.
Because KGF acts only on immature TEC progenitors, the stromal response to MSCs is limited to the enlargement of residual cells in the Foxn1−/− thymus as shown in Figure 2. This expansion of thymic epithelial cells, however, is sufficient to support thymopoiesis as shown by the detection of CD4/CD8 double positive cells in the thymus and the high TREC content in the splenocytes from Foxn1−/− mice6 weeks after MSC administration, which is consistent with a previous observation that KGF treatment for 6 weeks resulted in increases in the thymic export of mature T cells to the spleen and the establishment of regular thymic homeostasis.40
Furthermore, we detected an increased percentage of Treg cells in the spleen and peripheral blood after the administration of MSCs to Foxn1−/− mice, which was consistent with the improved thymopoiesis and T-cell emigration. MSCs have been administered to treat several autoimmune diseases, including systemic lupus erythematosus 10 and rheumatoid arthritis,41 which are caused by thymic dysfunction with low level of Treg cells in the peripheral blood.42,43 It is well known that Treg cells are an essential element in maintaining self-tolerance and preventing autoimmunity44,45 and are primarily generated from the thymus. In a previous study, we demonstrated that the level of Treg cells increased after UC-MSC treatment in active RA patients.12 Based on those results, we proposed the novel hypothesis that the efficacy of MSCs in the treatment of autoimmune diseases could be mediated by Treg function in the restoration of the thymic dysfunction in those patients.
In summary, the administration of UC-MSCs results in the robust regeneration of the thymic microenvironment and an improvement in thymic function that ensures an increase in T-cell development. This therapeutic effect of UC-MSCs may provide a promising method for the treatment of several diseases, including autoimmune diseases, age-associated thymus involution and the thymic damage caused by chemotherapy, ionizing radiation exposure and infections (e.g., HIV-1).
Acknowledgments
We thank Professor Dr Martin John Evans (University of Cardiff, UK) for constructive instruction and all members of the laboratory for their valuable discussion. The authors declare no competing financial interests. This work was supported by the National Natural Science Foundation of China (Grant: 30872618) and State High-tech Research and Development Plans (Grants: 2011AA020103 and 2011AA020109).
Notes
The authors indicate no potential conflicts of interest.
References
- Miller JF. Immunological function of the thymus. Lancet. 1961;2:748–749. [Abstract] [Google Scholar]
- Erickson M, Morkowski S, Lehar S, Gillard G, Beers C, Dooley J, et al. Regulation of thymic epithelium by keratinocyte growth factor. Blood. 2002;100:3269–3278. [Abstract] [Google Scholar]
- Gill J, Malin M, Holländer GA, Boyd R. Generation of a complete thymic microenvironment by MTS24+ thymic epithelial cells. Nat Immunol. 2002;3:635–642. [Abstract] [Google Scholar]
- Blackburn CC, Augustine CL, Li R, Harvey RP, Malin MA, Boyd RL, et al. The nu gene acts cell-autonomously and is required for differentiation of thymic epithelial progenitors. Proc Natl Acad Sci USA. 1996;93:5742–5746. [Europe PMC free article] [Abstract] [Google Scholar]
- Guo J, Feng Y, Barnes P, Huang FF, Idell S, Su DM, et al. Deletion of FoxN1 in the thymic medullary epithelium reduces peripheral T cell responses to infection and mimics changes of aging. PLoS ONE. 2012;7:e34681. [Europe PMC free article] [Abstract] [Google Scholar]
- Itoi M, Tsukamoto N, Yoshida H, Amagai T. Mesenchymal cells are required for functional development of thymic epithelial cells. Int Immunol. 2007;19:953–964. [Abstract] [Google Scholar]
- Anderson G, Jenkinson EJ, Moore NC, Owen JJ. MHC class II-positive epithelium and mesenchyme cells are both required for T-cell development in the thymus. Nature. 1993;362:70–73. [Abstract] [Google Scholar]
- Uccelli A, Moretta L, Pistoia V. Mesenchymal stem cells in health and disease. Nat Rev Immunol. 2008;8:726–736. [Abstract] [Google Scholar]
- Le Blanc K, Rasmusson I, Sundberg B, Götherström C, Hassan M, Uzunel M, et al. Treatment of severe acute graft-versus-host disease with third party haploidentical mesenchymal stem cells. Lancet. 2004;363:1439–1441. [Abstract] [Google Scholar]
- Sun L, Akiyama K, Zhang H, Yamaza T, Hou Y, Zhao S, et al. Mesenchymal stem cell transplantation reverses multiorgan dysfunction in systemic lupus erythematosus mice and humans. Stem Cells. 2009;27:1421–1432. [Europe PMC free article] [Abstract] [Google Scholar]
- Augello A, Tasso R, Negrini SM, Cancedda R, Pennesi G. Cell therapy using allogeneic bone marrow mesenchymal stem cells prevents tissue damage in collagen-induced arthritis. Arthritis Rheum. 2007;56:1175–1186. [Abstract] [Google Scholar]
- Wang L, Wang L, Cong X, Liu G, Zhou J, Bai B, et al. Human umbilical cord mesenchymal stem cell therapy for patients with active rheumatoid arthritis: safety and efficacy. Stem Cells Dev. 2013;22:3192–3202. [Abstract] [Google Scholar]
- Baksh D, Yao R, Tuan RS. Comparison of proliferative and multilineage differentiation potential of human mesenchymal stem cells derived from umbilical cord and bone marrow. Stem Cells. 2007;25:1384–1392. [Abstract] [Google Scholar]
- Ritter MA, Boyd RL. Development in the thymus: it takes two to tango. Immunol Today. 1993;14:462–469. [Abstract] [Google Scholar]
- Holub M, Rossmann P, Tlaskalova H, Vidmarova H. Thymus rudiment of the athymic nude mouse. Nature. 1975;256:491–493. [Abstract] [Google Scholar]
- Liu GY, Xu Y, Li Y, Wang LH, Liu YJ, Zhu D. Secreted galectin-3 as a possible biomarker for the immunomodulatory potential of human umbilical cord mesenchymal stromal cells. Cytotherapy. 2013;15:1208–1217. [Abstract] [Google Scholar]
- Weinberg K, Blazar BR, Wagner JE, Agura E, Hill BJ, Smogorzewska M, et al. Factors affecting thymic function after allogeneic hematopoietic stem cell transplantation. Blood. 2001;97:1458–1466. [Abstract] [Google Scholar]
- Fry TJ, Sinha M, Milliron M, Chu YW, Kapoor V, Gress RE, et al. Flt3 ligand enhances thymic-dependent and thymic-independent immune reconstitution. Blood. 2004;104:2794–2800. [Abstract] [Google Scholar]
- Voruganti VS, Tejero ME, Proffitt JM, Cole SA, Cox LA, Mahaney MC, et al. Characterization of ghrelin in pedigreed baboons: evidence for heritability and pleiotropy. Obesity (Silver Spring) 2008;16:804–810. [Abstract] [Google Scholar]
- Holland AM, van den Brink MR. Rejuvenation of the aging T cell compartment. Curr Opin Immunol. 2009;21:454–459. [Europe PMC free article] [Abstract] [Google Scholar]
- Kruyt MC, de Bruijn J, Veenhof M, Oner FC, van Blitterswijk CA, Verbout AJ, et al. Application and limitations of chloromethyl-benzamidodialkylcarbocyanine for tracing cells used in bone tissue engineering. Tissue Eng. 2003;9:105–115. [Abstract] [Google Scholar]
- Loeffler J, Bauer R, Hebart H, Douek DC, Rauser G, Bader P, et al. Quantification of T-cell receptor excision circle DNA using fluorescence resonance energy transfer and the LightCycler system. J Immunol Methods. 2002;271:167–175. [Abstract] [Google Scholar]
- Itoh M, Takahashi T, Sakaguchi N, Kuniyasu Y, Shimizu J, Otsuka F, et al. Thymus and autoimmunity: production of CD25+CD4+ naturally anergic and suppressive T cells as a key function of the thymus in maintaining immunologic self-tolerance. J Immunol. 1999;162:5317–5326. [Abstract] [Google Scholar]
- Shevach EM, McHugh RS, Piccirillo CA, Thornton AM. Control of T-cell activation by CD4+ CD25+ suppressor T cells. Immunol Rev. 2001;182:58–67. [Abstract] [Google Scholar]
- Yamazaki S, Inaba K, Tarbell KV, Steinman RM. Dendritic cells expand antigen-specific Foxp3+ CD25+ CD4+ regulatory T cells including suppressors of alloreactivity. Immunol Rev. 2006;212:314–329. [Abstract] [Google Scholar]
- Xiao S, Manley NR. Impaired thymic selection and abnormal antigen-specific T cell responses in Foxn1(Delta/Delta) mutant mice. PLoS ONE. 2010;5:e15396. [Europe PMC free article] [Abstract] [Google Scholar]
- Su DM, Navarre S, Oh WJ, Condie BG, Manley NR. A domain of Foxn1 required for crosstalk-dependent thymic epithelial cell differentiation. Nat Immunol. 2003;4:1128–1135. [Abstract] [Google Scholar]
- Naquet P, Naspetti M, Boyd R. Development, organization and function of the thymic medulla in normal, immunodeficient or autoimmune mice. Semin Immunol. 1999;11:47–55. [Abstract] [Google Scholar]
- Gray D, Abramson J, Benoist C, Mathis D. Proliferative arrest and rapid turnover of thymic epithelial cells expressing Aire. J Exp Med. 2007;204:2521–2528. [Europe PMC free article] [Abstract] [Google Scholar]
- Bleul CC, Corbeaux T, Reuter A, Fisch P, Mönting JS, Boehm T. Formation of a functional thymus initiated by a postnatal epithelial progenitor cell. Nature. 2006;441:992–996. [Abstract] [Google Scholar]
- Devine SM, Cobbs C, Jennings M, Bartholomew A, Hoffman R. Mesenchymal stem cells distribute to a wide range of tissues following systemic infusion into nonhuman primates. Blood. 2003;101:2999–3001. [Abstract] [Google Scholar]
- Lax S, Ross EA, White A, Marshall JL, Jenkinson WE, Isacke CM, et al. CD248 expression on mesenchymal stromal cells is required for post-natal and infection-dependent thymus remodelling and regeneration. FEBS Open Bio. 2012;2:187–190. [Europe PMC free article] [Abstract] [Google Scholar]
- Nehls M, Pfeifer D, Schorpp M, Hedrich H, Boehm T. New member of the winged-helix protein family disrupted in mouse and rat nude mutations. Nature. 1994;372:103–107. [Abstract] [Google Scholar]
- Nehls M, Kyewski B, Messerle M, Waldschütz R, Schüddekopf K, Smith AJ, et al. Two genetically separable steps in the differentiation of thymic epithelium. Science. 1996;272:886–889. [Abstract] [Google Scholar]
- Chen L, Xiao S, Manley NR. Foxn1 is required to maintain the postnatal thymic microenvironment in a dosage-sensitive manner. Blood. 2009;113:567–574. [Europe PMC free article] [Abstract] [Google Scholar]
- Wolf SS, Cohen A. Expression of cytokines and their receptors by human thymocytes and thymic stromal cells. Immunology. 1992;77:362–368. [Abstract] [Google Scholar]
- Finch PW, Rubin JS. Keratinocyte growth factor/fibroblast growth factor 7, a homeostatic factor with therapeutic potential for epithelial protection and repair. Adv Cancer Res. 2004;91:69–136. [Abstract] [Google Scholar]
- Goldberg GL, Zakrzewski JL, Perales MA, van den Brink MR. Clinical strategies to enhance T cell reconstitution. Semin Immunol. 2007;19:289–296. [Europe PMC free article] [Abstract] [Google Scholar]
- Dixit VD, Yang H, Sun Y, Weeraratna AT, Youm YH, Smith RG, et al. Ghrelin promotes thymopoiesis during aging. J Clin Invest. 2007;117:2778–2790. [Europe PMC free article] [Abstract] [Google Scholar]
- Rossi SW, Jeker LT, Ueno T, Kuse S, Keller MP, Zuklys S, et al. Keratinocyte growth factor (KGF) enhances postnatal T-cell development via enhancements in proliferation and function of thymic epithelial cells. Blood. 2007;109:3803–3811. [Europe PMC free article] [Abstract] [Google Scholar]
- Gonzalez MA, Gonzalez-Rey E, Rico L, Büscher D, Delgado M. Treatment of experimental arthritis by inducing immune tolerance with human adipose-derived mesenchymal stem cells. Arthritis Rheum. 2009;60:1006–1019. [Abstract] [Google Scholar]
- Koetz K, Bryl E, Spickschen K, O'Fallon WM, Goronzy JJ, Weyand CM. T cell homeostasis in patients with rheumatoid arthritis. Proc Natl Acad Sci USA. 2000;97:9203–9208. [Europe PMC free article] [Abstract] [Google Scholar]
- Kayser C, Alberto FL, da Silva NP, Andrade LE. Decreased number of T cells bearing TCR rearrangement excision circles (TREC) in active recent onset systemic lupus erythematosus. Lupus. 2004;13:906–911. [Abstract] [Google Scholar]
- Fontenot JD, Rudensky AY. A well adapted regulatory contrivance: regulatory T cell development and the forkhead family transcription factor Foxp3. Nat Immunol. 2005;6:331–337. [Abstract] [Google Scholar]
- Sakaguchi S. Naturally arising CD4+ regulatory t cells for immunologic self-tolerance and negative control of immune responses. Annu Rev Immunol. 2004;22:531–562. [Abstract] [Google Scholar]
Articles from Cellular and Molecular Immunology are provided here courtesy of Nature Publishing Group
Full text links
Read article at publisher's site: https://doi.org/10.1038/cmi.2013.69
Read article for free, from open access legal sources, via Unpaywall:
https://www.nature.com/articles/cmi201369.pdf
Citations & impact
Impact metrics
Citations of article over time
Alternative metrics

Discover the attention surrounding your research
https://www.altmetric.com/details/135566449
Smart citations by scite.ai
Explore citation contexts and check if this article has been
supported or disputed.
https://scite.ai/reports/10.1038/cmi.2013.69
Article citations
Mesenchymal stem cells reverse thymus aging by reprogramming the DNA methylation of thymic epithelial cells.
Regen Ther, 27:126-169, 26 Mar 2024
Cited by: 0 articles | PMID: 38571892 | PMCID: PMC10988135
Mesenchymal stromal cells ameliorate chronic GVHD by boosting thymic regeneration in a CCR9-dependent manner in mice.
Blood Adv, 7(18):5359-5373, 01 Sep 2023
Cited by: 4 articles | PMID: 37363876 | PMCID: PMC10509672
Potential role of mesenchymal stem cells in T cell aging.
J Mol Med (Berl), 101(11):1365-1378, 26 Sep 2023
Cited by: 0 articles | PMID: 37750918
Review
A new era of gene and cell therapy for cancer: a narrative review.
Ann Transl Med, 11(9):321, 09 May 2023
Cited by: 2 articles | PMID: 37404986 | PMCID: PMC10316111
Review Free full text in Europe PMC
The Lineage Differentiation and Dynamic Heterogeneity of Thymic Epithelial Cells During Thymus Organogenesis.
Front Immunol, 13:805451, 22 Feb 2022
Cited by: 10 articles | PMID: 35273595 | PMCID: PMC8901506
Go to all (14) article citations
Data
Similar Articles
To arrive at the top five similar articles we use a word-weighted algorithm to compare words from the Title and Abstract of each citation.
Foxn1 maintains thymic epithelial cells to support T-cell development via mcm2 in zebrafish.
Proc Natl Acad Sci U S A, 109(51):21040-21045, 03 Dec 2012
Cited by: 26 articles | PMID: 23213226 | PMCID: PMC3529077
Thymopoiesis in mice depends on a Foxn1-positive thymic epithelial cell lineage.
Proc Natl Acad Sci U S A, 107(38):16613-16618, 07 Sep 2010
Cited by: 83 articles | PMID: 20823228 | PMCID: PMC2944754
Foxn1 is required to maintain the postnatal thymic microenvironment in a dosage-sensitive manner.
Blood, 113(3):567-574, 31 Oct 2008
Cited by: 146 articles | PMID: 18978204 | PMCID: PMC2628364
FOXN1 in thymus organogenesis and development.
Eur J Immunol, 46(8):1826-1837, 01 Aug 2016
Cited by: 62 articles | PMID: 27378598 | PMCID: PMC4988515
Review Free full text in Europe PMC