Abstract
Free full text

Tsc1 promotes the differentiation of memory CD8+ T cells via orchestrating the transcriptional and metabolic programs
Significance
Enhancing the generation and function of memory T cells represents a crucial strategy to improve protective immunity against pathogens and tumors. The signaling pathway via mechanistic target of rapamycin (mTOR) has been implicated in the regulation of the differentiation of effector and memory T cells, but the upstream regulators or downstream mechanisms remain unclear. In this study, we provide insight into the mechanistic basis that controls mTOR signaling and memory T-cell responses. The deficiency of tuberous sclerosis 1 (Tsc1) in antigen-experienced T cells impairs the differentiation of memory T-cell precursors and the formation of memory T cells, associated with excessive mTOR activity and dysregulated cell metabolism. Our study establishes a molecular mechanism that links mTOR signaling and cell metabolism for memory T-cell development.
Abstract
Memory CD8+ T cells are an essential component of protective immunity. Signaling via mechanistic target of rapamycin (mTOR) has been implicated in the regulation of the differentiation of effector and memory T cells. However, little is understood about the mechanisms that control mTOR activity, or the effector pathways regulated by mTOR. We describe here that tuberous sclerosis 1 (Tsc1), a regulator of mTOR signaling, plays a crucial role in promoting the differentiation and function of memory CD8+ T cells in response to Listeria monocytogenes infection. Mice with specific deletion of Tsc1 in antigen-experienced CD8+ T cells evoked normal effector responses, but were markedly impaired in the generation of memory T cells and their recall responses to antigen reexposure in a cell-intrinsic manner. Tsc1 deficiency suppressed the generation of memory-precursor effector cells while promoting short-lived effector cell differentiation. Transcriptome analysis indicated that Tsc1 coordinated gene expression programs underlying immune function, transcriptional regulation, and cell metabolism. Furthermore, Tsc1 deletion led to excessive mTORC1 activity and dysregulated glycolytic and oxidative metabolism in response to IL-15 stimulation. These findings establish a Tsc1-mediated checkpoint in linking immune signaling and cell metabolism to orchestrate memory CD8+ T-cell development and function.
Memory CD8+ T cells play an important role in protective immunity with the capability to mount robust recall responses upon reexposure to antigens derived from tumor cells or infection. Recent studies have revealed developmental pathways and transcriptional programs important for the generation of long-lived memory cells (1). Antigen-activated CD8+ T cells rapidly expand and generate heterogeneous populations of effector cells, namely short-lived effector cells (SLECs) and memory-precursor effector cells (MPECs) (2, 3). SLECs (CD127loKLRG1hi) exhibit strong cytotoxicity with high expression of perforin and granzymes and are predisposed to cell death, whereas MPECs (CD127hiKLRG1lo) display the increased potential to survive and further differentiate into mature memory CD8+ T cells. The fate decision between SLECs and MPECs is important for effector functions and memory differentiation and is shaped by a number of transcription factors. For instance, transcription factors Eomes and Bcl6 promote the generation of MPECs, whereas Blimp1 and T-bet drive the transcriptional programs for SLEC differentiation (1).
T cells dynamically reprogram cellular metabolism to fulfill the bioenergetics and biosynthetic requirements for their survival, proliferation, and differentiation (4–6). Naïve and memory T cells use catabolic metabolism via oxidative phosphorylation, especially fatty acid oxidation, to produce ATP for their survival. In contrast, antigen-stimulated T cells switch to anabolism to support their rapid proliferation through up-regulating expression of genes involved in multiple metabolic pathways, including glycolysis, fatty acid and cholesterol biosynthesis, and amino acid transport (7–10). Emerging studies indicate that distinct metabolic pathways contribute to the fate decisions of effector and memory T cells. For instance, the increased glycolytic metabolism promotes effector T-cell generation (11), whereas oxidative phosphorylation and mitochondrial spare respiratory capacity facilitate memory T-cell differentiation (12, 13). Recent studies have also identified transcriptional regulators of cell metabolism that promote effector T-cell differentiation, including HIF1 and IRF4 (14–17). In contrast, how cell metabolism is regulated by immune signaling pathways in effector and memory T-cell differentiation remains unclear.
Mechanistic target of rapamycin (mTOR), an evolutionally conserved serine–threonine kinase and the catalytic component of mTORC1 and mTORC2 complexes, is a crucial controller of T-cell activation and function (18, 19). mTOR signaling has been implicated in the control of effector and memory T-cell differentiation (20–22). Inhibition of mTOR signaling by rapamycin promotes the generation of MPECs and their subsequent differentiation into memory T cells upon acute lymphocytic choriomeningitis virus (LCMV) infection (20). In vitro treatment of effector cells with rapamycin also enhances the developmental potential of memory cells through increasing the expression of Eomes at the expense of T-bet (21). Moreover, rapamycin enhances the ability of homeostatic proliferation-induced memory CD8+ T cells against tumor challenge via regulating the expression of Eomes and T-bet (22). Despite these studies of linking mTOR signaling to the regulation of memory T-cell differentiation, the upstream regulators of mTOR remain unresolved. Notably, deletion of Pten, a crucial negative regulator of Akt–mTOR signaling, does not cause significant defects in memory formation in LCMV infection (23). Moreover, whether mTOR or the canonical activator Akt impacts memory T-cell differentiation via metabolic pathways or other pathways such as cell migration is unclear (24). Of note, Akt regulates the differentiation and function of effector CD8+ T cells via orchestrating the transcriptional program instead of cellular metabolism (25). Therefore, the upstream and downstream mechanisms for mTOR-dependent regulation of memory generation remain to be defined.
Tuberous sclerosis 1 (Tsc1), a negative regulator of mTORC1 signaling (26), has been implicated in T-cell homeostasis and anergy (27–30). We and others have demonstrated that deletion of Tsc1 in naïve T cells disrupts their quiescence and survival and dampens T-cell–mediated primary responses to bacterial infection (27–29). To circumvent the crucial requirement of Tsc1 in naïve T-cell homeostasis, we developed a mouse model to specifically delete Tsc1 in antigen-experienced CD8+ T cells. We describe here that Tsc1 plays a critical role in promoting the differentiation and function of memory CD8+ T cells in Listeria monocytogenes infection. In contrast to the deletion of Tsc1 in naïve T cells, mice with Tsc1 deficiency in antigen-experienced CD8+ T cells retained normal effector responses but were markedly impaired in the recall response to antigen reexposure. Mechanistically, Tsc1 deficiency diminished the generation of MPECs while promoting the development of SLECs in a cell-intrinsic manner. The functional genomic analysis indicated that Tsc1 coordinated gene expression programs underlying immune function, transcriptional regulation, and cell metabolism. Furthermore, Tsc1 deletion led to excessive mTORC1 activity and dysregulated metabolism including glycolysis and oxidative phosphorylation in response to IL-15 stimulation. These findings establish a previously unappreciated role of Tsc1 in linking immune signaling and cell metabolism to orchestrate memory CD8+ T-cell development and function.
Results
Tsc1-Deficient T Cells Mount Normal Effector Responses but Are Impaired to Transition into Memory Cells.
Tsc1 is essential to maintain the quiescence of naïve T cells. T-cell–specific deletion of Tsc1 via CD4–Cre mice impairs naïve T-cell survival, homeostasis, and primary immune responses (27–29). Hence, this mouse model impedes the investigation of Tsc1 functions in antigen-specific effector and memory responses. To this end, we crossed mice carrying the floxed Tsc1 alleles (Tsc1fl/fl) with those expressing the Cre recombinase under control of the Granzyme B promoter (GzmB–Cre) (31) to specifically delete Tsc1 in antigen-experienced CD8+ T cells (called “Tsc1−/− mice” here). Under steady state, Tsc1−/− mice had normal distribution of CD4+ and CD8+ T cells (Fig. S1A). Homeostasis of these T cells was also undisrupted, as indicated by the normal expression of CD62L, CD44, and CD127 (Fig. S1 B and C), consistent with the absence of Tsc1 deletion in naïve CD8+ T cells (Fig. S1D).
To examine whether Tsc1 deficiency affects effector responses, we challenged wild-type Tsc1fl/fl mice (called “WT mice” here) and Tsc1−/− mice with L. monocytogenes expressing the chicken ovalbumin (LM–OVA), and assessed antigen-specific CD8+ T cells in various organs at day 9 postinfection (p.i.), the peak phase of effector cell expansion. Real-time PCR results showed that Tsc1 genomic DNA and mRNA were both effectively deleted (Fig. S2 A and B). However, the frequency and number of OVA-specific CD8+ T cells were similar between WT and Tsc1−/− mice in various organs examined (Fig. S2C). Also, Tsc1−/− effector cells in the spleen and liver showed slightly increased proliferation and largely normal death, as indicated by BrdU incorporation and Caspase-3 staining, respectively (Fig. S2 D and E). To measure the ability of antigen-specific CD8+ T cells to produce cytokines, we restimulated splenocytes from infected mice with the OVA257–264 [comprised of amino acids Ser-Ile-Ile-Asn-Phe-Glu-Lys-Leu (SIINFEKL)] peptide ex vivo. WT and Tsc1−/− splenocytes had similar frequency and number of antigen-specific T cells producing IFN-γ and TNF-α (Fig. S2F). Thus, loss of Tsc1 in antigen-experienced CD8+ T cells does not significantly affect effector responses.
After the rapid expansion of antigen-experienced CD8+ T cells, the majority of effector cells undergo cell death, whereas only a small subset survives and differentiates into memory cells (1). We investigated whether Tsc1 deficiency impairs the transition from effector to memory T cells by following the dynamics of OVA-specific T cells in the blood of WT and Tsc1−/− mice infected with LM–OVA. After clonal expansion, OVA-specific T cells in WT mice underwent the contraction phase, followed by the formation of long-lived memory cells (Fig. 1A). Consistent with the data described above, the frequency of Tsc1−/− OVA-specific T cells was largely normal at day 9, but the decline was much greater than that of WT cells (Fig. 1A). Therefore, loss of Tsc1 resulted in a progressive defect in the generation of memory T cells. To further address this question, we measured OVA-specific T cells in the spleen of WT and Tsc1−/− mice at different time points. At day 18 p.i., when Tsc1−/− splenocytes had yet to show significant reduction of antigen-specific CD8+ T cells (Fig. 1B), these cells already up-regulated Caspase-3 activity (Fig. S3A). In contrast, Tsc1−/− cells showed largely normal proliferation at this stage, as indicated by Ki-67 expression and BrdU incorporation (Fig. S3B). Moreover, at day 41 p.i., the number of OVA-specific CD8+ T cells was significantly reduced in the spleen and liver of Tsc1−/− mice (Fig. 1 B and C). We next examined cytokine production by memory CD8+ T cells at day 41 via restimulating splenocytes with the OVA peptide ex vivo. Consistent with the reduced number of memory CD8+ T cells, Tsc1−/− mice had fewer antigen-specific cells producing IFN-γ and TNF-α than WT mice (Fig. 1D). Therefore, Tsc1 depletion in antigen-experienced CD8+ T cells impairs the transition from effector responses to memory formation.
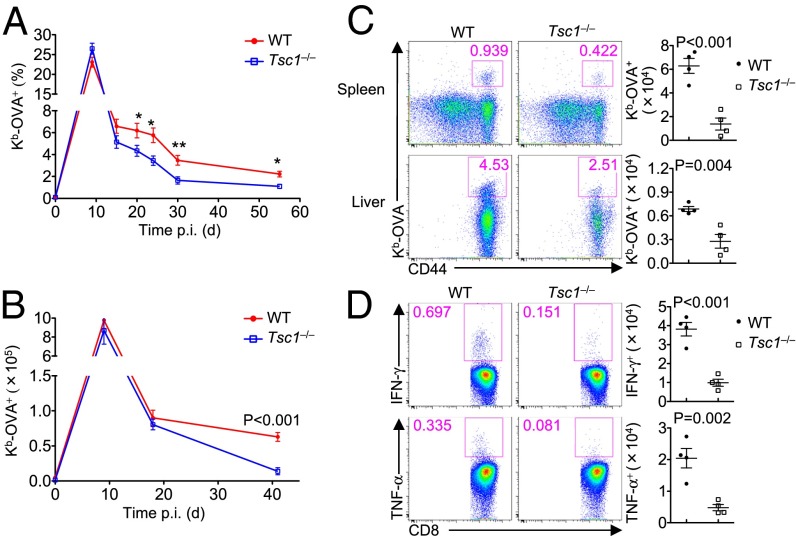
The absence of Tsc1 diminishes the generation of memory T cells. (A) Flow cytometry of the frequency of Kb-OVA+ CD8+ T cells in the blood from WT and Tsc1−/− mice infected with LM–OVA at the indicated time points (*P < 0.05, **P < 0.01). (B) Number of Kb-OVA+ CD8+ T cells in the spleen from WT and Tsc1−/− mice at days 0, 9, 18, and 41 p.i. (C) Flow cytometry of CD8+ T cells (Left) and the number of Kb-OVA+ CD8+ T cells (Right) in the spleen and liver from WT and Tsc1−/− mice at day 41 p.i. (D) Splenocytes from WT and Tsc1−/− mice at day 41 p.i. were restimulated with the SIINFEKL peptide for intracellular cytokine staining of IFN-γ and TNF-α. Shown are representative flow cytometry plots of CD8+ T cells (Left) and the number (Right) of IFN-γ+ and TNF-α+ CD8+ T cells. Data are representative of three independent experiments and are presented as the mean ± SEM.
Tsc1 Deletion Impairs the Recall Response of Memory Cells.
One hallmark of memory T cells is their ability to mount accelerated recall responses to antigen reexposure. To examine the role of Tsc1 in this process, we used LM–OVA to rechallenge WT and Tsc1−/− mice at day 35 after primary infection. At day 4 after the secondary infection, WT mice mounted robust recall responses in the blood, spleen, and liver (Fig. 2A). In contrast, the frequency and number of OVA-specific CD8+ T cells were significantly reduced in Tsc1−/− mice (Fig. 2A). We next assessed cytokine production of WT and Tsc1−/− splenocytes after restimulation with the OVA peptide ex vivo. Tsc1−/− mice had reduced frequency and number of T cells producing IFN-γ and TNF-α compared with WT mice (Fig. 2B). Therefore, Tsc1 deficiency impairs the recall response of memory cells.
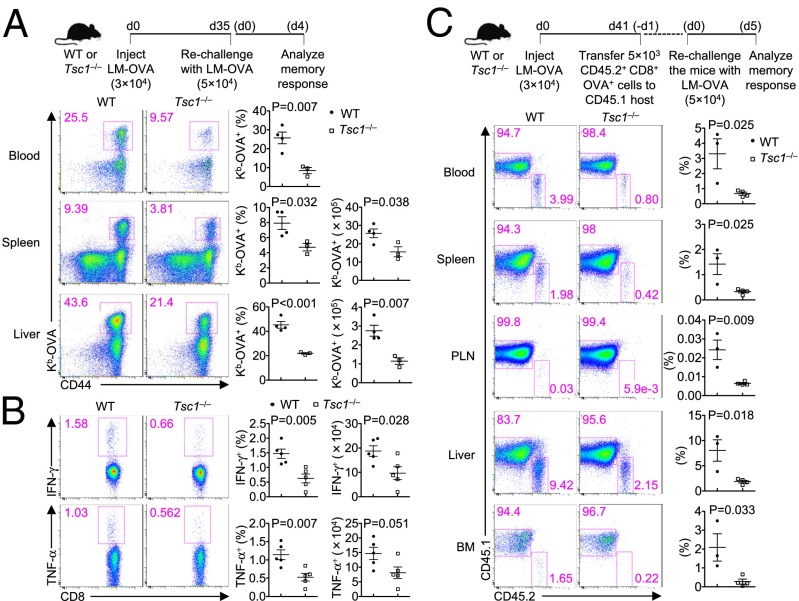
Tsc1 deficiency impairs the recall response of memory CD8+ T cells. (A) WT and Tsc1−/− mice were infected with LM–OVA and rechallenged with LM–OVA 35 d later. The recall response of memory CD8+ T cells was examined at day 4 after secondary infection. Shown are representative flow cytometry plots of CD8+ T cells (Left) and the frequency (Center) and number (Right) of Kb-OVA+ CD8+ T cells. (B) At day 4 after the secondary infection, splenocytes from WT and Tsc1−/− mice were isolated and restimulated with the SIINFEKL peptide for intracellular cytokine staining of IFN-γ and TNF-α. Shown are representative flow cytometry plots of CD8+ T cells (Left) and the frequency (Center) and number (Right) of IFN-γ+ and TNF-α+ CD8+ T cells. (C) Memory CD8+ T cells (CD45.2+) sorted from WT and Tsc1−/− mice at day 41 p.i. were transferred into naïve CD45.1+ recipients. At 24 h, the recipients were challenged with LM–OVA and analyzed 5 d later for CD45.1 and CD45.2 staining of CD8+ T cells (Left) and the frequency of CD45.2+ donor cells (Right). BM, bone marrow; PLN, peripheral lymph nodes. Data are representative of two independent experiments and are presented as the mean ± SEM.
One potential caveat of the experimental system above was that the defective recall response of Tsc1−/− memory T cells could be ascribed to the uneven numbers of memory T cells in WT and Tsc1−/− mice before rechallenge. To circumvent this possibility, we sorted OVA-specific memory T cells from WT and Tsc1−/− mice (CD45.2+) at day 41 p.i. and adoptively transferred equal numbers of WT and Tsc1−/− memory T cells into naïve recipients (CD45.1+), followed by LM–OVA infection 24 h later. At day 5 p.i., we assessed the frequency of donor-derived cells in various organs. The frequency of Tsc1−/− donor cells was significantly reduced compared with that of WT donor cells in all organs examined (Fig. 2C). We conclude that Tsc1 is required for the recall response of memory cells upon antigen reexposure.
A Cell-Intrinsic Requirement of Tsc1 in Memory Formation and Function.
Given the role of Tsc1 in the homeostasis of naïve T cells (27–29), it remains possible that the T-cell receptor (TCR) repertoire of Tsc1−/− T cells could be altered and contributed to the defects observed above. We therefore crossed Tsc1−/− mice onto the TCR-transgenic background (OT-I) in which CD8+ T cells expressed a SIINFEKL peptide-specific TCR. We mixed congenically marked naive OT-I and Tsc1−/−OT-I cells at a 1:1 ratio and cotransferred them to WT recipient mice, followed by LM–OVA infection. This adoptive transfer system also allowed us to exclude the effects of potential Tsc1 deletion in cells other than CD8+ T cells. Consistent with the normal effector responses observed in Tsc1−/− mice (Fig. S2), the frequencies of OT-I and Tsc1−/−OT-I cells were comparable at day 9 p.i. (Fig. 3A). However, at day 29 and 57 p.i., the percentage of Tsc1−/−OT-I cells was substantially lower than that of OT-I cells (Fig. 3A), indicative of a defect in the generation of memory cells. Upon LM–OVA rechallenge, the frequencies of Tsc1−/−OT-I cells were also significantly lower than those of OT-I cells in various organs examined (Fig. 3B). Moreover, among IFN-γ– or TNF-α–producing cells in the recipient mice, the majority of them were derived from OT-I instead of Tsc1−/−OT-I donor cells (Fig. 3 C and D). These results establish a cell-intrinsic role of Tsc1 in promoting the formation and function of memory CD8+ T cells.
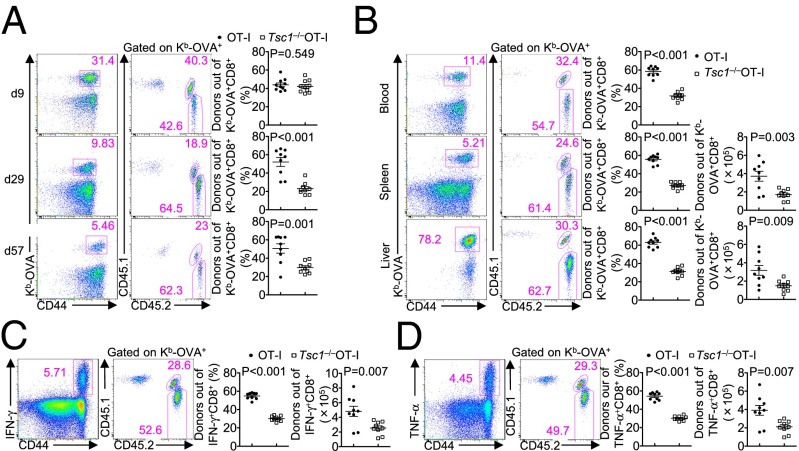
Tsc1 promotes memory T-cell generation through cell-intrinsic mechanisms. Naïve CD8+ T cells from OT-I+ (CD45.2+) and Tsc1−/−OT-I+ (CD45.1.2+) mice were isolated, mixed at a 1:1 ratio, and transferred to CD45.1+ recipients, followed by LM–OVA infection 1 d later. (A) Flow cytometry and frequencies of OT-I+ (CD45.2+) and Tsc1−/−OT-I+ (CD45.1.2+) donor T cells out of the total Kb-OVA+ CD8+ cells in the blood at days 9, 29, and 57 p.i. (B–D) At day 57 p.i., mice were rechallenged with LM–OVA and analyzed 3 d later by flow cytometry. (Right) The frequencies and numbers of OT-I+ (CD45.2+) and Tsc1−/−OT-I+ (CD45.1.2+) out of the total Kb-OVA+ CD8+ T cells from the blood, spleen, and liver (B) or out of splenic IFN-γ+ (C) or TNF-α+ (D) CD8+ T cells after restimulation with SIINFEKL peptide. Data are representative of three independent experiments and are presented as the mean ± SEM.
Impaired Differentiation of Memory Precursors in the Absence of Tsc1.
Aside from rapid expansion, effector cells differentiate into diverse subsets with distinct potentials for the formation of memory T cells: MPECs (CD127hiKLRG1lo) and SLECs (CD127loKLGR1hi) (2, 3). To investigate whether Tsc1 deficiency affects the differentiation of MPECs and SLECs, we examined the expression of CD127 and KLRG1 on WT and Tsc1−/− effector cells at day 9 p.i. We found that Tsc1−/− OVA-specific CD8+ T cells had markedly reduced frequency and number of MPECs, whereas the frequency and number of SLECs were increased (Fig. 4A). Similar findings were observed in Tsc1−/−OT-I T cells in the adoptive transfer system (Fig. S4A). Moreover, to exclude the potential role of Tsc1 in cell survival (Fig. S3A), we crossed Tsc1−/− mice with mice expressing a Bcl2 transgene in lymphocytes (Bcl2-transgenic, Bcl2-Tg) (29). Following LM–OVA infection, Tsc1−/−Bcl2-Tg T cells showed reduced MPECs but increased SLECs, compared with the Bcl2-Tg counterparts (Fig. S4B). Taken together, these results reveal an intrinsic effect of Tsc1 deficiency on the fate decisions between MPECs and SLECs.
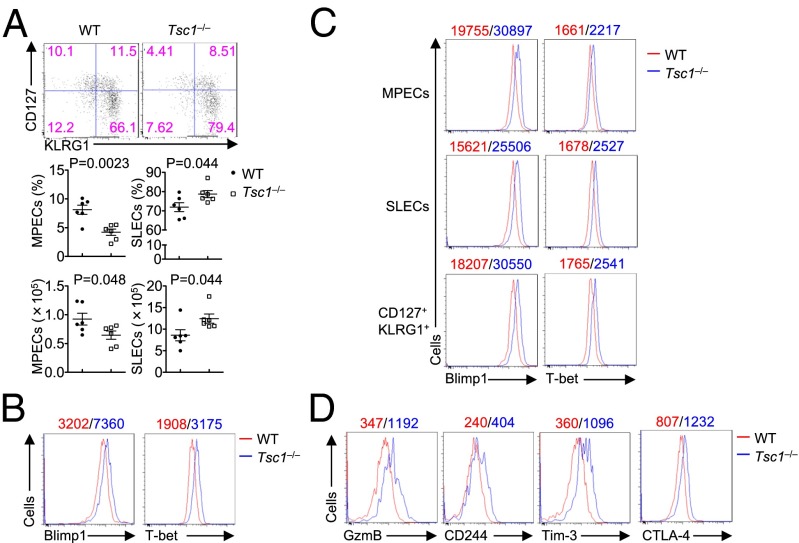
Tsc1 is required for the differentiation of MPECs. (A) Flow cytometry of CD127 and KLRG1 (Top) on Kb-OVA+ CD8+ T cells from WT and Tsc1−/− mice at day 9 p.i., and the frequency (Middle) and number (Bottom) of MPECs (CD127hiKLRG1lo) and SLECs (CD127loKLRG1hi). (B) Intracellular staining of Blimp1 and T-bet in splenic Kb-OVA+ CD8+ T cells from WT and Tsc1−/− mice at day 9 p.i. (C) Intracellular staining of Blimp1 and T-bet in MPECs, SLECs, and CD127hiKLRG1hi cells in splenic Kb-OVA+ CD8+ T cells from WT and Tsc1−/− mice at day 9 p.i. (D) Flow cytometry of GzmB, CD244, Tim-3, and CTLA-4 at day 9 p.i. in Kb-OVA+ CD8+ T cells from WT and Tsc1−/− mice. Mean fluorescent intensity (MFI) is presented above the plots (WT, red; Tsc1−/−, blue; B–D). Data are representative of three independent experiments and are presented as the mean ± SEM.
The differentiation of activated CD8+ T cells into MPECs and SLECs is controlled by the differential expression of specific transcription factors, with Eomes and Bcl6 important in promoting the differentiation of MPECs (1). The expression of these transcription factors in antigen-specific CD8+ T cells from WT and Tsc1−/− mice at day 9 p.i. was largely comparable (Fig. S5). However, Tsc1 deficiency considerably enhanced the expression of Blimp1 and T-bet, which are crucial factors for the development of SLECs and cytotoxic T-lymphocyte (CTL) functions (1) (Fig. 4B). The altered expression of Blimp1 and T-bet was observed in subdivided populations including MPECs, SLECs, and CD127+KLRG1+ cells (Fig. 4C), highlighting a direct effect of Tsc1 deficiency on these transcription factors. Moreover, Tsc1−/− CD8+ T cells had elevated expression of Granzyme B and immune inhibitory receptors CD244 (2B4), Tim-3, and CTLA-4 (32–34) (Fig. 4D). Therefore, consistent with the impaired memory development and MPEC formation, Tsc1 deficiency enhances the expression of effector-promoting transcription factors Blimp1 and T-bet and multiple immune inhibitory receptors.
Tsc1-Dependent Gene Expression Programs in Antigen-Specific CD8+ T Cells.
We performed functional genomics to identify additional pathways controlled by Tsc1 by comparing global gene expression profiles of WT and Tsc1−/− OVA-specific CD8+ T cells sorted from mice at day 9 p.i. Tsc1−/− cells contained a total of 420 increased probes and 378 decreased probes by greater than 0.5 log2 fold change between the comparison (Fig. 5A). The heat maps in Fig. 5B showed that Tsc1−/− CD8+ T cells differentially expressed multiple genes (with log2 fold change > 0.5) associated with the differentiation of effector and memory CD8+ T cells, including receptors and secreted factors (Il12rb2, Gzmb, Sell, and IL7r) and transcription factors (Tcf7, Bach2, and Klf4). Additionally, Tsc1−/− CD8+ T cells had altered expression of genes involved in various metabolic pathways, including glycolysis (Tpi1), cholesterol synthesis (Fdps, Hmgcs1, and Insig1), and fatty acid synthesis (Fads1 and Elovl7) (Fig. 5B). Therefore, Tsc1 coordinates the expression of immune response and metabolic genes in antigen-specific CD8+ T cells.
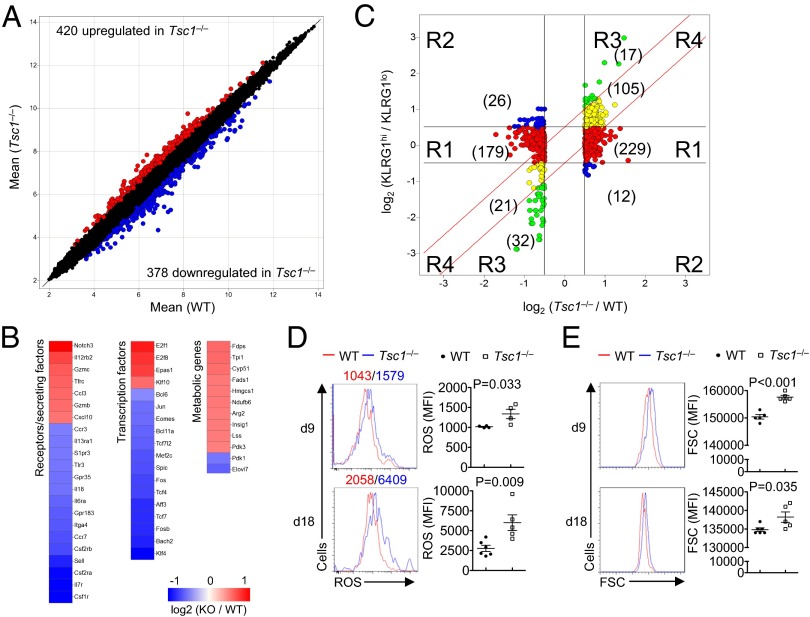
Tsc1-dependent gene expression profiles in antigen-experienced CD8+ T cells. (A) Scatterplot comparison of global gene expression profiles between WT and Tsc1−/− Kb-OVA+ CD8+ T cells sorted from mice at day 9 p.i.; transcripts with >0.5 log2 fold change are highlighted (WT, n = 4; Tsc1−/−, n = 5). (B) Heat maps of differentially expressed genes in Kb-OVA+ CD8+ T cells (with >0.5 log2 fold change), with red color denoting up-regulated genes in Tsc1−/− cells and blue color down-regulated genes in Tsc1−/− cells. (C) Comparison of expression changes in Tsc1−/− versus WT cells with those in KLRG1hi versus KLRG1lo cells. The Tsc1 target genes (>0.5 log2 fold change) were partitioned into four main clusters, shown and colored by regions (R1–R4). Numbers within parentheses indicate the number of probes within each subregion. (D and E) ROS production (D) and cell size (E) of splenic Kb-OVA+ CD8+ T cells from WT and Tsc1−/− mice at day 9 and 18 p.i. Data are representative of one experiment (A–C) and two independent experiments (D and E) and are presented as the mean ± SEM.
Given the effects of Tsc1 on the differentiation of MPECs and SLECs, it remains possible that the altered gene profiles of Tsc1−/− cells were simply secondary to the disrupted ratios of MPECs and SLECs. To address this issue, we compared Tsc1-dependent targets with the genes differentially expressed in KLRG1hi and KLRG1lo CD8+ T cells in the public database (35). Out of 798 probes with a log2 fold change > 0.5 in Tsc1−/− cells, 621 were matched to this database and were included for further analysis (Dataset S1). These Tsc1 targets were partitioned into four distinct clusters that differed in their relationship to the specific gene targets in KLRG1hi cells (Fig. 5C). A salient feature was that the majority of Tsc1 targets (408 out of 621 probes) fell into cluster 1 (red circles), in which their expression was comparable between KLRG1hi and KLRG1lo cells (<0.5 log2 fold change). Further, cluster 2 (blue circles) contained 38 probes that showed the opposite direction of change in expression, and cluster 3 (green circles) contained 49 probes that were differentially expressed in both types of comparisons, but to a greater extent in KLRG1hi cells compared with Tsc1−/− cells. Only cluster 4 (yellow circles, 126 probes) contained the target genes with equal magnitude of change (>0.5 log2 fold change) in both Tsc1−/− and KLRG1hi cells, thus representing concordant changes in both types of cells. Overall, ~80% of all Tsc1 gene targets are independent of KLRG1 expression (clusters 1–3), indicating a distinct gene expression program controlled by Tsc1.
We next used the ingenuity pathway analysis (IPA) system to analyze canonical pathways controlled by Tsc1 in activated CD8+ T cells by investigating the differentially expressed genes with a false discovery rate (FDR) < 0.1. Fig. S6A shows the top 11 canonical pathways affected by Tsc1 deficiency with P < 0.01, including oxidative phosphorylation and mitochondria dysfunction. Finally, to identify key networks regulated by Tsc1, we did a gene-set enrichment analysis (GSEA) to compare the gene expression profiles of WT and Tsc1−/− Kb-OVA+ CD8+ cells. This unbiased approach identified multiple pathways with significant enrichment, including cell cycle, cholesterol biosynthesis, and oxidative phosphorylation, all of which were up-regulated in Tsc1−/− CD8+ T cells (Fig. S6 B–D).
Given that oxidative phosphorylation was affected by Tsc1 deficiency in both GSEA and IPA analysis, we examined mitochondrial reactive oxygen species (ROS) production, which is associated with oxidative phosphorylation (5). Tsc1−/− CD8+ T cells showed increased ROS production at day 9 and 18 p.i. (Fig. 5D). Moreover, Tsc1−/− CD8+ cells had larger cell sizes than WT cells (Fig. 5E), indicative of increased cell growth. Altogether, these data indicate that Tsc1 coordinates diverse pathways involved in immune function, transcriptional regulation, and cell growth and metabolism.
Dysregulated mTORC1 and Metabolic Activities in Tsc1-Deficient CD8+ T Cells.
Cytokines IL-2 and IL-15 are critical for the development and maintenance of CD8+ effector and memory T cells, respectively (36). To understand upstream and downstream mechanisms underlying Tsc1-dependent memory T-cell differentiation, we used an in vitro culture system that mimics the programs of effector and memory T-cell differentiation (13, 37) and examined the effect of acute deletion of Tsc1 in this system. To this end, we crossed Tsc1fl/fl with Rosa26–Cre–ERT2 (CreER) and OT-I transgenic mice. We sorted naïve CD8+ T cells from Tsc1+/+ CreER+ OT-I and Tsc1fl/fl CreER+ OT-I mice and activated them with OVA and IL-2 in the presence of 4-hydroxytamoxifen (4-OHT) for 4 d, followed by an additional culture in either IL-2 or IL-15. The deletion of Tsc1 after 4-OHT treatment was confirmed by real-time PCR analysis (Fig. S7A), which was also reflected in the increased cell size (Fig. S7B). IL-2 and IL-15 induce distinct effects on T-cell growth and metabolic activities (13, 38), although how these metabolic programs are regulated is poorly defined. We therefore examined oxygen consumption rate (OCR) and extracellular acidification rate (ECAR), which denote mitochondria respiration and glycolysis, respectively, in cells cultured with IL-2 or IL-15. Consistent with previous observations (13), IL-15 mediated a stronger effect on OCR than IL-2 did, whereas IL-2 but not IL-15 potently elevated ECAR activity (Fig. 6 A and B). Importantly, Tsc1 deficiency markedly enhanced ECAR and OCR in cells cultured with IL-15, but effects were very modest in cells cultured with IL-2 (Fig. 6 A and B). Thus, Tsc1 deletion results in dysregulated metabolic activity during IL-15–mediated differentiation of memory T cells.
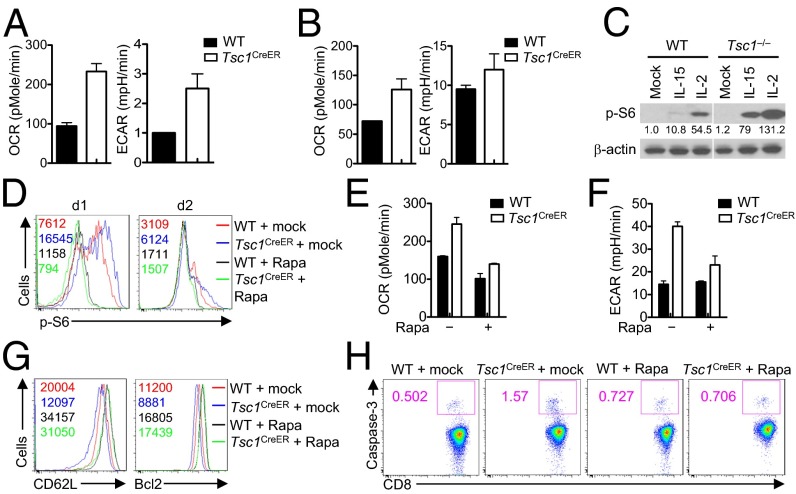
Tsc1 deficiency leads to dysregulated mTORC1 activity and metabolism in activated CD8+ T cells. (A and B) Naïve CD8+ T cells from OT-I+ Tsc1+/+ CreER+ (WT) and OT-I+ Tsc1fl/fl CreER+ (Tsc1CreER) mice were stimulated with OVA in the presence of IL-2 and 4-OHT for 4 d, and live cells were isolated and cultured with IL-15 or IL-2 for 5 d. OCR and ECAR were measured in IL-15– (A) and IL-2–cultured cells (B). (C) Phosphorylation of S6 in WT and Tsc1CreER cells activated with OVA and then stimulated with IL-2 or IL-15 for 18 h. Numbers below lanes indicate band intensity relative to that of β-actin (loading control). (D) Flow cytometry of S6 phosphorylation in WT and Tsc1CreER cells activated with OVA and then stimulated with IL-15 for 1 and 2 d in the absence or presence of rapamycin (Rapa). (E and F) OCR (E) and ECAR (F) of WT and Tsc1CreER cells activated with OVA and then stimulated with IL-15 for 24 h in the absence or presence of rapamycin. (G and H) Flow cytometry of CD62L, Bcl2 (G), and Caspase-3 activity (H) in WT and Tsc1CreER cells activated with OVA and then stimulated with IL-15 for 2–3 d in the absence or presence of rapamycin. Data are representative of three independent experiments and are presented as the mean ± SEM.
Moreover, compared with Tsc1-sufficient controls, Tsc1-deficient T cells up-regulated mTORC1 activity, as indicated by the increased phosphorylation of the ribosomal protein S6. Notably, the extent of up-regulation over control cells was much more profound in IL-15–stimulated conditions (~7.5-fold) compared with IL-2–stimulated conditions (~2.5-fold) (Fig. 6C). Furthermore, treatment of IL-15–stimulated cells with rapamycin considerably blocked the dysregulated mTORC1 (Fig. 6D), OCR (Fig. 6E), and ECAR activities (Fig. 6F), as well as T-cell glycolytic activity (Fig. S7C). Therefore, excessive mTORC1 activation in the absence of Tsc1 drives the metabolic dysregulation. Moreover, compared with IL-2, IL-15 has more of a stringent requirement for Tsc1 functions in actively suppressing mTORC1 and mTORC1-dependent metabolic activities.
Associated with dysregulated metabolism, Tsc1-deficient cells down-regulated CD62L and Bcl2 expression (Fig. 6G) but up-regulated Caspase-3 activity (Fig. 6H). Importantly, rapamycin treatment restored the excessive activity of mTORC1 and the dysregulated expression of CD62L, Bcl2, and Caspase-3 activity (Fig. 6 G and H). Therefore, Tsc1 links mTORC1 activation, cell metabolism, and immune regulation.
Discussion
In this study, we reveal an important function of Tsc1 in promoting the differentiation and function of memory CD8+ T cells in bacterial infection. By developing a mouse model to ablate Tsc1 specifically in antigen-experienced CD8+ T cells, we found that Tsc1 is dispensable for effector responses at the expansion phase, but is essential for the formation of memory CD8+ T cells and their recall responses to antigen reexposure in a T-cell–intrinsic manner. Mechanistically, deficiency of Tsc1 dampens the differentiation of MPECs, associated with the impaired transcriptional and metabolic programs. In particular, loss of Tsc1 results in excessive mTORC1 activity and dysregulated glycolytic and oxidative metabolism in response to IL-15 stimulation. These findings provide new mechanistic insight into T-cell memory formation and function and highlight that modulation of Tsc1 function is a potential strategy to improve the quality and quantity of memory responses.
Emerging studies highlight a pivotal role of mTOR signaling in the fate decisions of effector and memory T cells (20–23), but the upstream regulators of mTOR remain unresolved. Notably, mTOR can be activated by Akt-independent pathways in effector CD8+ T cells (14, 25), and deletion of Pten does not cause significant defects in memory formation (23). Here we circumvented the requirements of mTOR signaling in naïve T-cell homeostasis (27–29) and immediate TCR activation (10), by specific ablation of Tsc1 in antigen-experienced CD8+ T cells. Although Tsc1 deficiency elevates mTORC1 activity, this does not significantly impact the generation of effector CD8+ T cells at the peak phase of primary responses. Instead, Tsc1 function is essential to ensure proper differentiation between MPECs and SLECs, which is a prerequisite for the subsequent generation of memory T cells (1). Specifically, Tsc1 deficiency suppresses the formation of MPECs and reciprocally promotes the formation of SLECs. These effects were observed in multiple independent systems including direct challenge, adoptive transfer of OT-I cells, and Bcl2-Tg backgrounds, thereby highlighting a direct role of Tsc1 in cell fate decisions. Accordingly, Tsc1-deficient antigen-specific CD8+ T cells have increased expression of Blimp1 and T-bet, which likely suppress the function of transcription factors required for memory differentiation (1). Additionally, Tsc1 deletion up-regulates inhibitory receptors associated with T-cell exhaustion (32–34). Moreover, Tsc1 deficiency impairs the expression of genes involved in T-cell trafficking, such as l-selectin (Sell) and CCR7, which are required for the migration to central-memory T cells in the T-cell areas of secondary lymphoid organs (24). These results indicate that Tsc1 promotes memory T-cell responses, in part, by impinging upon the transcriptional programs required for memory precursor differentiation.
The metabolic programs are dynamically regulated to match the differentiation and function of T cells (4–6). For the fate decisions between effector and memory CD8+ T cells, the glycolytic and lipid synthetic metabolism promotes effector T-cell generation (7, 11), whereas oxidative phosphorylation and mitochondrial activity facilitate memory development (12, 13). From the microarray and bioinformatics analyses, we found that Tsc1−/− antigen-specific CD8+ T cells exhibit elevated expression of metabolic genes involved in glycolysis, lipid synthesis, and oxidative phosphorylation. Moreover, Tsc1-deficient T cells show enhanced glycolytic and oxidative phosphorylation rates in vitro that are closely linked with IL-15–mediated signaling. Although the increased glycolysis upon Tsc1 deletion is in agreement with impaired development of memory cells (11), the effect of the increased oxidative phosphorylation remains to be established. Notably, the IPA analysis indicates that Tsc1 deficiency impinges upon both oxidative phosphorylation and mitochondria function. Further, loss of Tsc1 results in excessive production of mitochondrial ROS. These findings indicate that Tsc1 functions to ensure proper regulation of the metabolic programs, the disruption of which may contribute to impaired memory T-cell differentiation.
We and others have previously established a key role of Tsc1 in the establishment of naïve T-cell quiescence (27–29). Although the exit from quiescence in response to TCR stimulation is essential for proper T-cell activation and effector responses (10), how memory T cells reestablish a quiescent state is unclear. The increased cell size and dysregulated metabolic activities of Tsc1-deficient T cells are consistent with an impaired quiescence state of memory T cells. Additionally, gene expression profiling indicated that Tsc1-deficient CD8+ T cells down-regulate the expression of transcription factors required for the maintenance of cell quiescence including Bach2 and Klf4 (39–41). Therefore, the failure to reestablish quiescence in Tsc1-deficient memory T cells is the likely basis for their poor recall responses to antigen rechallenge. This is reminiscent of the observation that abrogated quiescence of naïve T cells is associated with the defective initiation of primary immune responses (29).
In summary, Tsc1 functions as a critical controller of the generation and function of memory T cells. Tsc1 regulates the formation of memory T cells through shaping the fate decisions between MPECs and SLECs and by orchestrating the transcriptional and metabolic programs. Modulation of Tsc1 functions in T cells represents a potential strategy to enhance the quantity and quality of memory T cells against infections and tumors.
Materials and Methods
Mice were purchased from the Jackson Laboratory or described previously. All mice have been extensively backcrossed to the C57BL/6 background. Details of experimental procedures and reagents can be found in SI Materials and Methods.
Supplementary Material
Supplementary File
Supplementary File
Acknowledgments
The authors acknowledge J. Jacob and M. Li for GzmB–Cre mice. This work was supported by National Institutes of Health Grants AI105887, AI101407, CA176624, and NS064599.
Footnotes
The authors declare no conflict of interest.
*This Direct Submission article had a prearranged editor.
Data deposition: The data reported in this paper have been deposited in the Gene Expression Omnibus (GEO) database, www.ncbi.nlm.nih.gov/geo (accession no. GSE61591).
This article contains supporting information online at www.pnas.org/lookup/suppl/10.1073/pnas.1404264111/-/DCSupplemental.
References
Articles from Proceedings of the National Academy of Sciences of the United States of America are provided here courtesy of National Academy of Sciences
Full text links
Read article at publisher's site: https://doi.org/10.1073/pnas.1404264111
Read article for free, from open access legal sources, via Unpaywall:
https://www.pnas.org/content/pnas/111/41/14858.full.pdf
Citations & impact
Impact metrics
Citations of article over time
Alternative metrics
Smart citations by scite.ai
Explore citation contexts and check if this article has been
supported or disputed.
https://scite.ai/reports/10.1073/pnas.1404264111
Article citations
Cellular metabolism regulates the differentiation and function of T-cell subsets.
Cell Mol Immunol, 21(5):419-435, 02 Apr 2024
Cited by: 3 articles | PMID: 38565887 | PMCID: PMC11061161
Review Free full text in Europe PMC
TSC2 S1365A mutation potently regulates CD8+ T cell function and differentiation and improves adoptive cellular cancer therapy.
JCI Insight, 8(21):e167829, 08 Nov 2023
Cited by: 1 article | PMID: 37788104 | PMCID: PMC10721258
Regulation of CD8+ T memory and exhaustion by the mTOR signals.
Cell Mol Immunol, 20(9):1023-1039, 15 Aug 2023
Cited by: 8 articles | PMID: 37582972 | PMCID: PMC10468538
Review Free full text in Europe PMC
Effects of altered glycolysis levels on CD8+ T cell activation and function.
Cell Death Dis, 14(7):407, 08 Jul 2023
Cited by: 22 articles | PMID: 37422501 | PMCID: PMC10329707
Review Free full text in Europe PMC
Absence of TSC1 Accelerates CD8+ T cell-mediated Acute Cardiac Allograft Rejection.
Aging Dis, 13(5):1562-1575, 01 Oct 2022
Cited by: 0 articles | PMID: 36186130 | PMCID: PMC9466980
Go to all (48) article citations
Data
Data behind the article
This data has been text mined from the article, or deposited into data resources.
BioStudies: supplemental material and supporting data
Similar Articles
To arrive at the top five similar articles we use a word-weighted algorithm to compare words from the Title and Abstract of each citation.
Role of tumor suppressor TSC1 in regulating antigen-specific primary and memory CD8 T cell responses to bacterial infection.
Infect Immun, 82(7):3045-3057, 12 May 2014
Cited by: 15 articles | PMID: 24818661 | PMCID: PMC4097607
TSC1/mTOR-controlled metabolic-epigenetic cross talk underpins DC control of CD8+ T-cell homeostasis.
PLoS Biol, 17(8):e3000420, 21 Aug 2019
Cited by: 18 articles | PMID: 31433805 | PMCID: PMC6719877
Mammalian Target of Rapamycin Complex 2 Controls CD8 T Cell Memory Differentiation in a Foxo1-Dependent Manner.
Cell Rep, 14(5):1206-1217, 21 Jan 2016
Cited by: 78 articles | PMID: 26804903
Modulation of TSC-mTOR signaling on immune cells in immunity and autoimmunity.
J Cell Physiol, 229(1):17-26, 01 Jan 2014
Cited by: 26 articles | PMID: 23804073
Review
Funding
Funders who supported this work.
NCI NIH HHS (3)
Grant ID: R01 CA176624
Grant ID: P30 CA021765
Grant ID: CA176624
NIAID NIH HHS (4)
Grant ID: AI101407
Grant ID: R01 AI105887
Grant ID: AI105887
Grant ID: R01 AI101407
NINDS NIH HHS (2)
Grant ID: R01 NS064599
Grant ID: NS064599