Abstract
Free full text

Type 2 diabetes mellitus: From a metabolic disorder to an inflammatory condition
Abstract
Diabetes mellitus is increasing at an alarming rate and has become a global challenge. Insulin resistance in target tissues and a relative deficiency of insulin secretion from pancreatic β-cells are the major features of type 2 diabetes (T2D). Chronic low-grade inflammation in T2D has given an impetus to the field of immuno-metabolism linking inflammation to insulin resistance and β-cell dysfunction. Many factors advocate a causal link between metabolic stress and inflammation. Numerous cellular factors trigger inflammatory signalling cascades, and as a result T2D is at the moment considered an inflammatory disorder triggered by disordered metabolism. Cellular mechanisms like activation of Toll-like receptors, Endoplasmic Reticulum stress, and inflammasome activation are related to the nutrient excess linking pathogenesis and progression of T2D with inflammation. This paper aims to systematically review the metabolic profile and role of various inflammatory pathways in T2D by capturing relevant evidence from various sources. The perspectives include suggestions for the development of therapies involving the shift from metabolic stress to homeostasis that would favour insulin sensitivity and survival of pancreatic β-cells in T2D.
Core tip: Immuno-metabolism, the confluence of metabolism and immune system has emerged as a chief breakthrough especially in the field of diabetes mellitus; a metabolic disorder of great magnitude. Activation of immune system by metabolic stress has opened new insights in the pathogenesis and progression of type 2 diabetes (T2D). The link between metabolic overload and activation of the immune system form the core tip of this review. Metabolic stress can cause pathologic activation of the immune system, thus metabolic disorders like T2D manifest and progress as an inflammatory disorder with severe consequences thereof.
INTRODUCTION
Diabetes mellitus, a life style disease affecting 8.3% of the adult population of the world and increasing at an alarming rate, is one of the most common non-communicable diseases of current era[1]. The burden of this disease is immense owing to transition in lifestyle and dietary habits, ageing of the population and urbanization in the setting of a genetically predisposed environment[2]. The fact that the number of subjects with diabetes mellitus has doubled over the past three decades has made this disease a global challenge[3]. The number of diabetes mellitus patients is projected to increase from 382 million in 2013 to 592 million by 2035, denoting a net increase of 55%[1]. The predominant form is type 2 diabetes (T2D) which accounts for nearly 90% of all diabetes cases.
Diabetes mellitus-not so sweet
T2D is a metabolic disorder characterized by insulin resistance and pancreatic β-cell dysfunction as a consequence of unsettled hyperglycemia[4,5]. In response to nutrient spill over in the setting of insulin resistance and eventual β-cell dysfunction, the general fuel homoeostasis of body is altered[2]. Insulin resistance in target tissues and a relative deficiency of insulin secretion from pancreatic β-cells are the major features of T2D. β-cell hyperplasia and hyperinsulinaemia in response to insulin resistance occur in the preclinical period of disease. Relative insulin deficiency as a consequence of failure of β-cells to compensate for insulin resistance, progresses into overt T2D[6].
Metabolic alterations associated with T2D are well characterised by epidemiological and research based studies. The pathogenesis and progression of T2D is ascribed to four mechanisms; increased advanced glycation end product (AGE) formation, increased polyol pathway flux, activation of protein kinase C (PKC) isoforms, and increased hexosamine pathway flux[7]. Till recently no common linking element was apparent for these mechanisms: however, recently production of superoxide emerged as a unifying mechanism for these four pathways. Downstream to oxidative stress, activation of inflammatory pathways has emerged as an imperative link between T2D and inflammation. Since, abundant data have elucidated the role of oxidative stress in T2D pathogenesis. In this review, we will evaluate the inflammatory component of T2D and underscore the link between metabolic alterations in T2D and inflammation.
T2D AS AN INFLAMMATORY CONDITION
Studies investigating the relation between inflammation and T2D have coalesced sufficient data implicating the role of inflammation towards the development of insulin resistance and pathogenesis of T2D[8,9]. Metabolism and immune system were conventionally regarded as two distinctive mechanisms governing nutrient disposal and body defense, respectively. Typically, little was known about the coordination and interplay between these two systems. However, present research has led to combining these distinct entities as studies perceive pathological activation of the immune system as a regulatory mechanism associated with multiple disorders underlying the metabolic syndrome[10]. Potency of steroid hormones as immune suppressors and hyperglycemic inductors, metabolic alterations associated with pyrexia, wasting syndrome initiated by chronic infections and of late, markers of acute-phase response have been associated with insulin resistance, insulin secretion defects, T2D and vascular complications of T2D[8,11-15].
T2D encompasses colossal cellular factors characteristic of triggering inflammatory signalling cascades. A detailed analysis of these molecules cannot be underscored in this review, however their particular roles in T2D has been outlined in Table Table1.1. Consequently, T2D at the moment is considered an inflammatory disorder triggered by disordered metabolism[16]. The probable history of diabetes involves a more or less latent prodromal period followed by progressive deterioration of glucose tolerance culminating into explicit disease. Progression of islet β-cell failure results in hypertrophy of pancreatic islets and proliferation of β-cells. This phase is associated with an inflammatory response precipitating into reduction of cells by apoptosis and fibrosis of islets. In fact, an analogy of sequence of events involving an incipient inflammatory phase is associated with other T2D complications also[17]. Hyperglycemia is regarded as the major upstream mechanism, and micro-inflammation is regarded as the subsequent downstream driving force of diabetes related complications[17]. Epidemiological data advocate that markers of inflammation are predictive of T2D[18]. The role of inflammation in insulin resistance is traced by the integration of metabolism and innate immunity via nutrient-sensing pathways mutual to pathogen-sensing pathways. Components of nutrition (free fatty acids, glucose, and amino acids) signal through collective receptors and pathways in a similar way as pathogens and/or cytokines. Cells of the immune system (macrophages) and metabolism (adipocytes) also share many functions like secretion of cytokines, and trans-differentiation into macrophages. Nutrients can activate macrophages and adipocytes through common receptors, such as toll-like receptors (TLRs) that sense broad classes of molecular structures common to pathogen groups, and are central to innate immunity and inflammation.
Table 1
Role of various inflammatory molecules in type 2 diabetes
Category | Molecule | Role |
Pro-inflammatory cytokines and signaling molecules | TNF-α | Increased levels related to IR and T2D Reduces insulin sensitivity by influencing the phosphorylation state of the insulin receptor |
IL-6 | Major pro-inflammatory cytokine that induces inflammation and IR leading to T2D | |
CRP | Elevated serum CRP associated with the incidence of T2D | |
IL-1 | Associated with obesity and IR Affects insulin signaling directly through the induction of SOCS-3 | |
IL-8 | Leads to IR via the inhibition of insulin-induced Akt phosphorylation in adipocytes | |
IL-1β | Mediates auto-inflammatory process resulting in β-cell death | |
Transcription factors | NF-κB | Increase the expression of genes encoding cytokines, chemokines, transcription factors and various receptors involved in IR and pathogenesis of T2D |
JNK | Promotes IR through phosphorylation of serine residues in IRS-1 | |
IKKβ | Leads to IR through transcriptional activation of NF-κB | |
Adipokines | Leptin | High leptin levels, reflecting leptin resistance predict increased risk of T2D |
Adiponectin | Low levels of this protective adipokine correlate with T2D. Adiponectin is downregulated by TNF-α | |
Resistin | Promotes IR and decreases insulin-stimulated glucose transporters in adipose tissue | |
Adipsin | Role in maintaining β cell function Lower levels of adipsin found in T2D patient | |
Visfatin | Visfatin binds to the insulin receptor at a site distinct from that of insulin and causes hypoglycaemia by reducing glucose release from liver cells and stimulating glucose utilization in adipocytes and myocytes | |
Chemokines | MCP-1 | MCP-1 expression in adipose tissue contributes to the macrophage infiltration into this tissue, IR and T2D |
IP-10/CXCL10 | Downstream effector of pro-inflammatory cytokines involved in T2D-related complications | |
CCR2 | Imitates tissue inflammation and IR | |
Toll like receptor | TLR2 and TLR4 | TLR2 and TLR4 play a critical role in the pathogenesis of IR and T2D |
Adhesion molecules | E-slectin/P-slectin | Lead to leukocyte recruitment in local tissue and contributes to inflammation, IR and T2D |
ICAM-1/VCAM-1 | Alters endothelial and sub-endothelial structure leading to reduced vascular permeability, reduced insulin delivery to peripheral insulin sensitive tissues and ultimately T2D | |
Nuclear receptors | PPARα, PPARγ, and PPARβ/δ | Mutations in PPAR genes associated with IR and T2D |
VDR | Regulates expression of insulin receptor preferentially by binding as a heterodimer with the RXR to VDREs in the promoter regions of insulin receptor gene |
IR: Insulin resistance; CRP: C-reactive protein; T2D: Type 2 diabetes; SOCS-3: Suppressor of cytokine-3 signalling; NF-κB: Nuclear factor κB; JNK: c-Jun NH2-terminal kinase; IRS-1: Insulin receptor substrate; IKKβ: Inhibitor of nuclear factor κB kinase subunit β; MCP: Monocyte chemoattractant protein-1; IP-10: Interferon gamma-induced protein 10; CXCL10: Chemokine (C-X-C motif) ligand 10; CCR2: Chemokine (C-C) motif receptor 2; ICAM-1: Intracellular adhesion molecule 1; VCAM-1: Vascular cell adhesion molecule 1; PPAR: Peroxisome proliferator activated receptor; VDR: Vitamin D receptor; RXR: Retinoid X receptor; VDREs: Vitamin D response elements.
ADIPOSE TISSUE AS A SITE OF INFLAMMATION
Clinical and experimental studies show that adipose tissue acts as a site of inflammation. The first insight came from the study on adipose tissue of obese mice exhibiting elevated production of TNF-α[11]. Consequently, increase in adiposity is associated with upregulation of genes encoding pro-inflammatory molecules and associated with accumulation of immune cells[19-21]. Adipocytes hoard excessive nutrient load and become hypertrophic gradually. Events initiating a pro-inflammatory response involve synergistic contributions of various mechanisms like an increase in nuclear factor κB (NF-κB) and c-Jun NH2-terminal kinase (JNK) activity by hypertrophied adipocytes, endoplasmic reticulum (ER) stress causing altered unfolded protein response (UPR), hypoxic stress in adipose tissue, activation of TLR by excess free fatty acids (FFAs), or increased chylomicron-mediated transport from the gut lumen into the circulation in a lipid-rich diet[16,22,23]. Stressed adipocytes produce various cytokines and chemokines promoting immune-cell activation and accumulation in adipose tissue[24]. A pro-inflammatory loop is formed by several macrophages by clustering around adipocytes, particularly with dead adipocytes forming crown-like structures[19,21,25]. Sustained accumulation of lipids in adipose tissues results in switching of macrophages from an anti-inflammatory “M2” (alternatively activated) to a pro-inflammatory “M1” (classically activated) phenotype[19,21,26,27]. The skew in balance results in an increased secretion of inflammatory molecules that subsequently stimulate the hypertrophied adipocytes resulting into a pro-inflammatory response[28]. The inflammatory response in macrophages is induced by adipocyte-derived FFAs via TLR or NOD-like receptor family, the pyrin domain containing 3 (NLRP3) dependent pathways[29,30]. Local hypoxia as a result of vasculature insufficiency in hypertrophied adipocytes has been proposed to stimulate expression of inflammatory genes in adipocytes as well as immune cells[31]. However, the hypothesis lacks confirmation in the situation of human obesity[32]. Instead, mechanisms like ER stress and autophagocytosis have been proposed as origin of local inflammatory signalling pathways in adipose tissue[22,33]. Recently, the role of the incretin hormone glucose-dependent insulinotropic peptide has also been implicated[34,35]. In addition to adipose tissue, a pro-inflammatory state in liver and skeletal muscle result in disruption of systemic insulin sensitivity and glucose homeostasis that are characteristic of T2D[36-38].
Metabolic inflammation is regulated by critical orchestration of innate and adaptive immune cell interactions[39,40]. Studies investigating immuno-metabolism have recognised that the inflammatory status of immune cells is dictated by their metabolic programming, mitigating the progression of T2D. T2D is preceded by an extensive period of disease development, and inflammation has been shown to be a precipitating factor underpinning insulin resistance, preceding T2D[41,42]. The progression of T2D involves an intricate interplay between metabolism and immunity. The progression of T2D has been causally linked to various types of immune cells but the primary sources of inflammatory effectors contributing to insulin resistance are macrophages[43-45]. Among various cell types, pre-adipocytes, adipocytes, T cells, dendritic cells and macrophages are major cell types involved in obesity-induced inflammation and insulin resistance[46]. Their prime functions are shown in Figure Figure1.1. The key inducers of cytokine release in metabolic organs leading to impaired insulin action are tissue-resident macrophages[47].
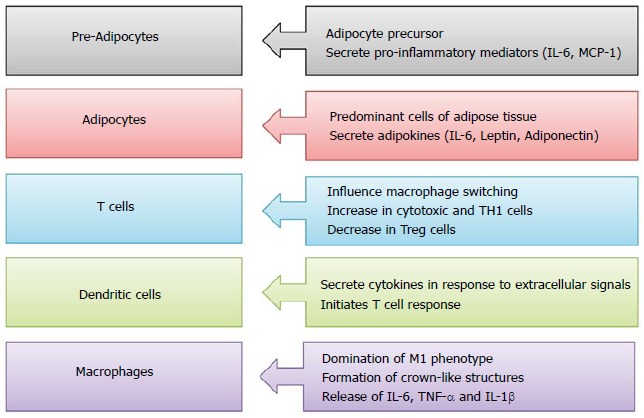
Functions of various immune cell types in pathogenesis of type 2 diabetes. IL: Interleukin; MCP-1: Monocyte chemoattractant protein-1; TNF-α: Tumor necrosis factor α.
Nutrient overload corresponds to increased infiltration of macrophages in metabolic tissues promoting a pro-inflammatory environment characterised by augmented TNF-α, IL-1β and inducible nitric oxide synthase (iNOS) levels. The accrual of these pro-inflammatory macrophages in metabolic organs like liver, adipose tissue and muscle directly supresses insulin action, thereby promoting hyperglycemia[48].
ROLE OF INFLAMMATION IN INSULIN RESISTANCE
Insulin is a key endocrine hormone produced by β-cells of pancreatic islets. Insulin is regarded as “hormone of abundance” owing to the array of functions it performs, the effects of which extend from metabolic to mitogenic activity (Figure (Figure2).2). It is likely that disruption of insulin-mediated pathways will have pleiotropic effects that are not confined to carbohydrate metabolism only. Various mechanism working separately or in synergy have been linked to the development of insulin resistance among which chronic inflammation represents as a triggering point[8].
Inflammation is an important component linking insulin resistance with nutrient overload and increased visceral adipocyte mass[42]. During an insulin-sensitive state, the signalling cascade of insulin upon binding to its receptor results in phosphorylation of tyrosine residues of the insulin receptor substrate 1 (IRS-1) ensuing in downstream insulin signalling[49]. However, in an insulin-resistance state, pro-inflammatory molecules activate various other serine kinases like JNK, inhibitor of NFκB kinase subunit β (IKK-β), extracellular-signal regulated kinase (ERK), ribosomal protein S6 kinase (S6K), mammalian target of rapamycin (mTOR), PKC and glycogen synthase kinase 3β[50]. The activation of these kinases inhibits insulin action by phosphorylating serine residues instead of tyrosine residues in the insulin signalling pathway[49].
The development of insulin resistance is linked to two prime transcription factor-sinalling pathways: JNK and IKKβ/NF-κB[51]. Activation of these two pathways involves a series of proinflammatory stimuli, many of which comprise of both activators and upregulators of NF-κB. In addition, these pathways are also activated by pattern recognition receptors like TLRs and receptors for advanced glycation end products (RAGE). Elevated levels of FFAs result in an increase in diacylglycerol (DAG) that activates PKC isoforms leading to concomitant activation of JNK and NF-κB pathways[52]. Further stimuli involve production of reactive oxygen species (ROS), ER stress and changes in adiposity[53-55].
The mechanisms in development of inflammation-induced insulin resistance are different for JNK and IKKβ. Unlike JNK that phosphorylates the serine residues of IRS-1, IKKB induces insulin resistance by transcriptional activation of NF-κB[56-59]. The physiological substrates of IKKβ are IκB protein inhibitors of NF-κB. IKKβ phosphorylation promotes proteosomal degradation of IκBα liberating NF-κB for nuclear translocation where it stimulates the expression of several target genes (Figure (Figure33)[9]. The products of these target genes of NF-κB induce insulin resistance. The production of inflammatory molecules further activates JNK and NF-κB pathways promoting a vicious loop of insulin resistance by feed-forward mechanism.
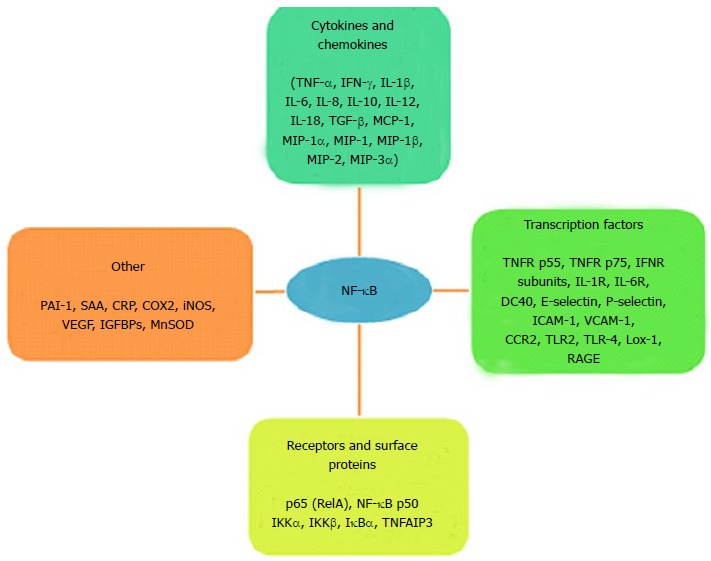
Target genes activated by NF-κB. TNF-α: Tumor necrosis factor-alpha; IFN-γ: Interferon-gamma; IL: Interleukin; TGF-β: Tumor growth factor-beta; MCP-1: Monocyte chemoattractant protein-1; MIP: Major intrinsic protein; TNFR: Tumor necrosis factor receptor; INFR: Interferon receptor; IL-R: Interleukin receptor; CD: Cluster of differentiation; ICAM: Intracellular cell adhesion molecule; VCAM: Vascular cell adhesion molecule; CCR: Chemokine CC receptor; TLR: Toll-like receptor; Lox: Lysyl oxidase; RAGE: Receptor advanced glycation end product; PAI: Plasminogen inhibitor activator; SAA: Serum amyloid; CRP: C-reactive protein; COX: Cyclo-oxygenase; iNOS: Inducible nitric oxide synthase; VEGF: Vascular endothelial growth factor; IGFBPs: Insulin-like growth factor binding protein; MnSOD: Manganese superoxide dismutase; RelA: Reticuloendotheliosis viral oncogene homolog A; NF-κB: Nuclear factor-kappa B; IKK: Inhibitor Kappa B kinase; IκBα: Inhibitor of NF-κB; TNFAIP3: TNF-α induced protein 3.
PANCREATIC ISLET INFLAMMATION IN T2D
Increasing evidence suggests the presence of an inflammatory milieu in pancreatic islets in T2D, such as increased cytokine levels, chemokine levels and immune cell infiltration. Evidence of islet inflammation was initially observed in hyperglycemia induced β-cell apoptosis[60]. Recent studies on human islets and monocytes have shown that the combination of hyperglycemia and elevated FFAs induces a more efficient pro-inflammatory phenotype[61,62]. Various T2D experimental animal models like db/db mice and Goto-Kakisaki rats showed increased infiltration by immune cells in the pancreatic islets[63]. Studies on experimental animal models elucidated islet inflammation and macrophage infiltration as an event occurring as early as eight weeks before the onset of frank diabetes[63]. Recruitment of macrophages is a consequence of phagocytic clearance owing to the death of islet β-cells[64]. Alternately, in a diabetic milieu endocrine cell-derived inflammatory molecules like IL-6 and IL-8 produced in islets are also attributed to increased macrophage infiltration[63]. Production of pro-inflammatory cytokines and secretion of chemokines by β-cells results in a vicious cycle speeding up islet inflammation. In humans, IL-1β secreted by infiltrating immune cells is related to the pathogenic process of T2D, as blockade of IL-1 has been associated with reduced hyperglycemia, improved β-cell function and reduced expression of inflammatory markers[65]. However, recent studies involving human islets have shown that induction of IL-1β plays a role in precipitating the clinical features of diabetes and is unlikely involved in initial pathogenesis[66-68]. The first study demonstrating the hyperglycemia-induced IL-1β secretion documented a pro-inflammatory response induced by a non-autoimmune mechanism in β-cells[12]. Ex vivo experiments on isolated human islets exposed to high glucose levels showed increased IL-1β production preceding activation of NF-κB, upregulation of Fas, fragmentation of DNA, and reduction of insulin secretion[69]. Upregulation of IL-1β plays a predominant role as a major cytokine regulating other chemokines and cytokines in islets of T2D patients[12,66,70,71]. This master cytokine elicits a broader response by recruitment of various immune cells and also by induction of IL-1β in β-cells, provoking a vicious inflammatory cycle[66]. The critical role of IL-1β in islet inflammation was recently confirmed by analysing global gene expression in pancreatic islets of humans that showed an association of a group of co-expressed modules enriched for IL-1 related genes with T2D and insulin resistance[72]. SFRP4 gene encoding the secreted frizzled-related protein 4 was one of the interesting genes that were overexpressed, likely mediating the effect of IL-1β on islets[72]. In islets of both T2D subjects as well as in animal models, an eminent number of immune cells along with cytokines and chemokines has been observed[63,66,73]. In fact, T2D animal models invariably exhibit islet immune cell infiltration[63,71].
ISLET INFLAMMATION AND β-CELL DEATH
Islet tissue sections of T2D subjects show well-defined fibrosis which is a hallmark of the late stage of a chronic inflammatory process. In clinically overt T2D subjects a decreased β-cell mass has been reported indicating a probable role in its pathogenesis[4,74]. Decreased β-cell mass in T2D has been attributed to pancreatic β-cell apoptosis and to β-cell dedifferentiation[75]. In slowly progressing T2D, the probability of detecting β-cell damage in pancreatic sections is low, thus very few studies on this aspect have been reported[4,76]. Several mechanisms like amyloid deposition in islets, presence of long-chain FFAs[77], and chronic hyperglycemia[60] has been implicated in β-cell apoptosis. Sustained gluco-lipotoxic conditions amplifies the β-cell stress responses by potentiating effects of elevated levels of FFAs, glucose causing ER stress and mTORC1 activation[78,79,54]. The underlying mechanism for hyperglycemia-induced β-cell apoptosis is attributed to the glucose-induced IL-1β production that upregulates the Fas receptor[80,81,12]. FFAs act as important effector molecules causing β-cell dysfunction by lipoapoptosis (a metabolic cause of programmed cell death). The most abundant saturated FFA in blood is palmitate that has direct lipotoxic effects on β-cells by inducing ER stress and ROS[82-85]. Ceramide, an effector molecule responsible for inducing lipoapoptosis of β-cells, is a metabolic product of FFAs that activates JNK[86-88]. Likewise, incomplete β-cell oxidation of fatty acids resulting in metabolites like DAG and triglycerides (TGs) also elicits final effector molecules contributing to FFA-induced lipotoxicity as well as insulin resistance[89-91]. In addition to this, FFA-induced activation of JNK by Src has also been reported in a recent study[92]. These studies show that islet inflammation contributes to β-cell dysfunction.
TRIGGERING OF THE INNATE IMMUNE SYSTEM IN T2D
Nutrient excess in metabolic tissues resulting in metabolic inflammation, i.e., a low-level pro-inflammatory milieu, has emerged as an important factor underlying the development of T2D[11-15,93,94]. Activation of innate immunity in T2D is linked to the activation of TLRs. These receptors have been implicated in diabetes-induced inflammation and vascular complications[95]. TLRs comprise the pattern-recognition receptors characteristic of the innate immune system. Various pathogen-associated molecular patterns (PAMPs) encompassing carbohydrates, proteins, nucleic acids and lipids, are recognised by TLRs followed by initiation of an immune response. TLR2, a receptor for pathogen lipoproteins and TLR4, a receptor of lipopolysaccharides, are activated by FFAs[96,29]. Binding of FFAs to TLRs has been postulated to directly induce a pro-inflammatory response[97,98]. Also, various indirect ways of TLR activation by FFAs has been postulated recently[99]. In vitro studies have demonstrated that, unlike the short chain FFAs, the long chain palmitate and oleate that comprise 80% of circulating FFAs are pro-inflammatory in various cell types[29,96,98,100,101]. Contemporary studies report the activation of TLR signalling by FFA-induced formation of lipid rafts that favour TLR dimerization in cell membranes[92,102]. Recently, fatty acid transporter CD36 binding to TLR2 and liver-derived glycoprotein fetuin-A binding to TLR4 were identified as endogenous ligands linking FFAs to TLRs, eliciting inflammation and prompting insulin resistance[103,104]. In addition, damage-associated molecular patterns (DAMPs) like high-mobility group box 1 (HMGB1) and AGEs also act as endogenous ligands which are recognised by TLRs, thereby activating pro-inflammatory pathways[105]. TLR2 is responsible for upregulation of inflammatory molecules like NF-κB, myeloid differentiating factor 88 (MyD88) and chemokine (C-C motif) ligand 2 (CCL2)[106]. TLR4 knockout mice have been shown to be protected from insulin resistance as well as from fat-induced inflammation[106]. TLR4 silencing by siRNA technology has been shown to attenuate the hyperglycemia-induced activation of IκB/NF-κB[107]. TLR5 is a receptor for bacterial flagellin that controls metabolic pathways through sensing gut microbiota. TLR5 knockout mice have been reported to exhibit increased adiposity along with hyperphagia, hypertension, hyperlipidemia and insulin resistance[108]. Activation of inflammatory pathways in a TLR-independent mechanism by metabolic stress involves generation of ROS that induce stress kinases and NLRP3 inflammasome (multiprotein complexes responsible for production of bioactive IL-1β) formation[109].
Both TLR-dependent and TLR-independent mechanisms function in concert. This finding is demonstrated by animal models of diabetes in which there is partly protection of pro-inflammatory cytokine production in case of deficiency of TLR2 or TLR4, whereas deficiency of a universal intracellular docking protein MyD88 required for TLR signalling, exerted total protection[61]. Apart from FFAs, systemic inflammatory responses are also elicited by elevated glucose levels[110]. Sustained hyperglycemia results in non-enzymatic glycation of lipids and proteins resulting in the formation of AGEs. AGEs stimulate the pattern recognition receptor RAGE. Numerous cell types, like macrophages, T cells, smooth muscle cells, neuronal cells, podocytes and cardiomyocytes, express RAGE[111]. RAGE activates the pleiotropic pro-inflammatory transcription factor NF-κB along with stress kinases ERK1 and ERK2[112]. Excessive glucose metabolized by oxidative phosphorylation to ATP results in ROS generation that tends to activate the NLRP3 inflammasome concomitantly with FFAs[67]. This results in release of active IL-1β along with IL-1-dependent cytokine and chemokine production[61].
FROM INNATE TO ADAPTIVE IMMUNITY IN T2DM
The role of specific or adaptive immunity comes from the recent clinical overlap between type 1 diabetes (T1D) and T2D such as younger age of onset in T2D and increasing body mass index (BMI) coinciding with increased incidence in T1D. Moreover, progressive decrease in β-cell mass observed in T2D and evidence of insulin resistance in T1D has blurred the etiology[113]. The argument supporting the involvement of autoimmunity in islets of T2D patients is evident from the presence of β-cell specific antibodies in nearly 10% of T2D patients and presence T cells reactive to β cell antigens in some patients[114]. The number of autoantigen-responsive T lymphocytes in islets from T2D patients has been reported to correlate with disease progression[114], however the exact role of islet autoimmunity in T2D requires further studies. A monogenic form of diabetes characterised by typical features of T1D like lean body mass, young age of onset, autoantibodies to β-cells, rapid disappearance of C-peptide and insulin requirement concomitantly with T2D-associated insulin resistance provides genetic support for the overlap between T1D and T2D[115]. The genetic alteration is attributed to an autosomal-dominant mutation in the SIRT1 gene, and the pathogenesis involves β-cell impairment and death, paralleling a state of activation of immune system[115]. As a consequence of insulin resistance, stress induced β-cell death results in the release of autoantigens along with alarmins (endogenous molecules released by necrotic cells causing activation of immune system). Alarmins have potentiating effects of promoting pathologic self-antigen presentation, resulting in enhanced adaptive immune response[116]. In light of these observations, sirtuins are recognised as novel regulators of immuno-metabolism in humans. Apart from SIRT1, SIRT2 has been recently linked to cytoskeleton remodeling and activation of NLRP3 in intracellular pathways[117]. Apart from the activation of innate immunity, the contribution of adaptive immune cells in inducing inflammation is now established in T2D at the cellular level.
Experimental animal models of insulin resistance have demonstrated a Th2/Th1 shift in favour of Th1, shifting the Treg/Th17 shift towards Th17 and shifting the CD8/CD4 ratio in favour of CD8 and finally reduction of T-cell receptor (TCR) diversity[118-121]. These studies have recently been extrapolated to human subjects[122] and confirm the observation that an increase pro-inflammatory stimuli (IFN-γ) causing M1 phenotype switching of adipose tissue macrophages result in the activation of a Th1 type response[121]. IFN-γ and IL-17 produced by these T cell populations interact directly with adipocytes in addition to contributing to a pro-inflammatory loop in cells of innate immunity. IFN-γ inhibits the JAK-STAT pathway, and IL-17 induces the secretion of IL-6 from adipocytes[123]. β-cells isolated from T2D patients exhibit increased IL-8 and decreased IL-10 secretion[124]. Recent studies regard the contribution of B-cell humoral immunity in adipose tissue inflammation. A study on experimental mice models involving B-cell knockout mice and anti-CD20 therapy showed a significantly improved metabolic phenotype and adipose tissue inflammation[120].
LINK BETWEEN ER STRESS AND INFLAMMATION IN T2D
Activation of ER stress and the UPR forms a convincing hypothesis for the induction of inflammatory pathways in T2D. ER stress in T2D occurs by virtue of nutrient overload, hypoxia and accumulation of unfolded proteins in metabolic organs[22]. Under normal conditions, the flux of proteins through ER is high, and in the setting of insulin resistance or glucotoxicity, a prolonged state of insulin need generates ER stress[125].
Three ER localized sensors control the activation of ER stress and UPR (Figure (Figure4):4): (1) the double-stranded RNA-activated protein kinase (PKR)-like ER kinase (PERK); (2) inositol-requiring kinase 1 (IRE1); and (3) activating transcription factor 6 (ATF6). ER stress by protein overload or accumulation of unfolded proteins causes dissociation of GRP78, and the subsequent binding to unfolded proteins in ER prevents their transport to cis Golgi.
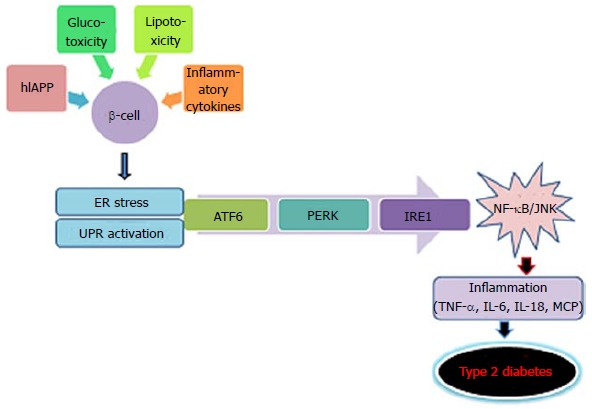
Mechanism of endoplasmic reticulum stress. ER: Endoplasmic-reticulum; ATF6: Activating transcription factor 6; PERK: Double-stranded RNA-activated protein kinase (PKR)-like ER kinase; IRE1: Inositol-requiring kinase 1; IL: Interleukin; MCP: Monocyte chemoattractant protein; TNF-α: Tumor necrosis factor α; JNK: c-Jun NH2-terminal kinase; NF-κB: Nuclear factor κB.
Prominently, UPR activation stimulates inflammatory stress kinases like JNK and IKK and their critical downstream transcriptional targets; activator protein 1 (AP-1) and NF-κB, respectively[126,127].
These transcription factors control the induction of inflammatory cytokines and chemoattractants that are known to have a direct link with the development of insulin resistance[128,129]. ER stress can also impair insulin signalling by activation of stress kinases (JNK, IKK) that can inhibit insulin receptor substrates by direct phosphorylation. Recently, death protein 5 (DP5) and p53-upregulated modulator of apoptosis (PUMA) have been reported as inducers of β-cell apoptosis by mediating ER stress[130]. ER stress can also cause induction of lipogenic genes that promote lipid accumulation and thereby contributes to the development of lipid-induced insulin resistance[131].
ER stress and UPR pathways
Triggering of inflammatory signals by three pathways of UPR is initiated by activation of JNKs and NF-κB in B cells. This activation acts as the linkage point between metabolic and immune pathways since the activation of these very kinases is analogous to that elicited by an immune response[94,132]. JNKs play an important role in T2D, as increased activity has been shown to promote insulin resistance [56,133].
The first responses for opposing ER stress involve decreasing the translation of proteins. This involves phosphorylation of α subunit of eIF2 by PERK. In humans and mice, loss of PERK expression is linked to dysregulation of the UPR response which is fundamental to ER stress, resulting in increased cell death and T2D[134]. A permanent form of neonatal diabetes in humans is related to elevated ER stress markers as a result of a mutation in PERK, confirming the pivotal role of PERK in regulating ER stress during fetal development[135-137].
A factor in the second pathway of UPR, IRE1, is a prime regulator of ER stress and is highly expressed in the pancreas. An in vitro knockdown study on IRE1 signalling showed a decreased synthesis of insulin[138,139]. Upon activation, IRE1 initiates activation of X-box binding protein 1 (XBP1) that leads to upregulation of ER expansion and biogenesis[140]. The critical role of XBP1 in achieving an optimal insulin secretion and glucose control was demonstrated in β-cell-specific XBP1-deficient mice that exhibited impaired pro-insulin processing and secretion, reduced β-cell proliferation and hyperactivation of IRE1[141].
The third pathway of UPR involves the activation of ATF6, the basic leucine zipper domain protein, that upregulates PERK1 and IRE1 pathways by suppressing the apoptotic UPR signalling cascade under chronic ER stress. The role of ATF6 activation in β-cell dysfunction has been concluded in studies that showed decreased expression of insulin gene by ER stress-induced ATF6 activation and a decrease in ER chaperones along with induction of apoptosis in ATF6 knockdown insulinoma cells[142,143].
ACTIVATION OF INFLAMMASOME IN T2D
Inflammasomes are multiprotein complexes in the intracellular machinery responsible for production of bioactive IL-1β in response to multiple stimuli[144]. NLRP is a subfamily of Nod-like receptors containing a central nucleotide binding and oligomerization (NACHT) domain with flanking C-terminal leucine-rich repeats (LRRs) and N-terminal caspase recruitment (CARD) or pyrin (PYD) domains[145]. The NOD-like receptor family, the pyrin domain containing 3 (NLRP3) inflammasome is in a pathway that controls the production of IL-1β and IL-18[146-148]. Unlike TLR, a potential role of NLR in metabolic abnormalities has not been extensively investigated. NLRP forms a constituent of the inflammasomes responsible for maturation and release of IL-1β, and thus is a relevant candidate for metabolic disorders and T2D[149]. NLRP3-dependent activation of inflammaosomes in diabetes was proposed by studies implicating the release of IL-1β as a consequence of elevated levels of glucose, FFAs and human islet amylopeptide (hIAPP)[16,150,151]. However, the effective metabolites involved in activation of inflammasomes are not clearly elucidated yet (Figure (Figure55).
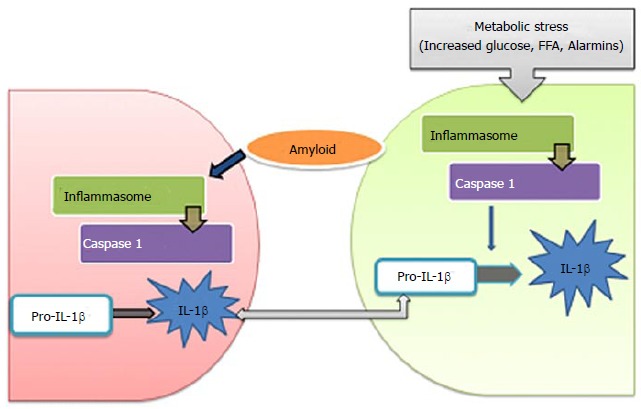
Activation of inflammasomes in type 2 diabetes (metabolic stress activates multiprotein complex, inflammasome in β-cells that induce caspase-1 to cleave pro-interleukin-1β (pro-IL-1β) into active IL-1β. β-cell-derived IL-1β promote the release of chemokines and recruitment of macrophages that are activated by human islet amyloid polypeptide, leading to deleterious concentrations of IL-1β. FFA: Free fatty acid; IL: Interleukin.
The NLRP3 inflammasome is a general metabolic alarmin stimulated by different endogenous and exogenous stimuli[152]. NLRP3 inflammasome activation is augmented in T2D patients[153]. Dysregulation of lipid metabolism, paving the way to aberrant lipid accumulation, as well as formation of oxidized LDL and cholesterol, triggers NLRP3 activation[30,153,154] similar to ER stress that acts as one of the important factors triggering NLRP3 activation[155,156]. In T2D subjects, increased oxidative stress also contributes to NLRP3 inflammasome activation[157,158].
Studies on obesity-induced inflammation and insulin resistance are also indicative of the role of NLRP3. In experimental models of calorie-restricted mice, a positive correlation has been observed between IL-1β/ NLRP3 mRNA and body weight[30] whereas disruption of NLRP3 gene in obese mice has revealed changes in metabolic profiles. Insulin resistance as a consequence of inflammasome activation is directly related to FFAs and LPS[109]. Apart from Insulin resistance, activation of inflammasomes is related to β-cell dysfunction, as NLRP3-knockout mice exhibit improved glycemic profiles after consumption of a high-fat diet, likely due to attenuation of IL-1β[67]. In response to hyperglycemia-induced increased production of ROS, NLRP3 activation occurs as a result of dissociation of thioredoxin interacting protein (TXNIP) from thioredoxin and its subsequent binding to NLRP3[67]. Nevertheless, shortage of TXNIP has shown effects on glucose metabolism in addition to the NLRP3 activation[159]. A substantial role of inflammasome activation in β-cell dysfunction was recently reported by ablation of NLRP3 that conferred protection to β-cell function and structure from injury inflicted by metabolic stress[160].
Secretion of IL-1β requires two induction stimuli; the first stimulus induces pro-IL-1β expression and the second inflammasome activation. Inflammasome activation triggers caspase-1 resulting in cleavage of pro-IL-1β and release of mature IL-1β. In T2D, the first stimulus comes from minimally-modified LDL in islets which prime the macrophages for processing of IL-1β by activation of TLR4 signalling. Recently, the second stimulus was recognized to regard islet hIAPP, secreted by β-cells in response to high glucose levels[151]. hIAPP was shown to direct NLRP3 activation by inducing β-cell injury. In islets, interaction of macrophages and β-cells is essential for the activation of inflammasomes. hIAPP, a soluble oligomer induces activation of NLRP3 and subsequent release of IL-1β from macrophages and dendritic cells which are primed with TLR4 agonists like LPS or modified LDL molecules[151]. The macrophages are attracted to islets by hIAPP-induced synthesis of chemokines (CCL2 and CXCL1). It has been reported that overexpression of hIAPP in islet grafts increases the recruitment of macrophages by 50%[161]. Recently the activation of inflammasomes in myeloid cells in T2D patients was elucidated. A study on untreated T2D subjects showed upregulation of IL-1β production and maturation in macrophages[153]. Treatment of macrophages with various alarmins like FFA, hIAPP, HMGB1and ATP resulted in release of inflammasome products. Studies have shown that T2D subjects exhibit elevated levels of circulating alarmin molecules thereby advocating a possible role of these molecules in NLRP3 inflammasome activation in myeloid cells[162].
PERSPECTIVES
The concept of chronic low-level inflammation in T2D has given an impetus to the field of immune-metabolism. Elucidation of various cellular mechanisms linking inflammation to insulin resistance and β-cell dysfunction has revolutionized insights in the molecular pathogenesis of diabetes. Insights into intricate pathways provide a platform to tackle the distinct pathway without compromising immuno-surveillance. Nutritional and therapeutic interventions aimed at controlling/inhibiting the escalating pro-inflammatory response can help in attenuating the pathogenesis and progression of T2D. Well-designed studies should offer the development of novel targeted therapeutics to deal with the disease burden of T2D and its associated complications.
Footnotes
Supported by Department of Science and Technology, Government of India to Iqra Hameed, No. Wos-A LS 509/2012.
Conflict-of-interest: All the authors declare that they have no conflict of interest.
Open-Access: This article is an open-access article which was selected by an in-house editor and fully peer-reviewed by external reviewers. It is distributed in accordance with the Creative Commons Attribution Non Commercial (CC BY-NC 4.0) license, which permits others to distribute, remix, adapt, build upon this work non-commercially, and license their derivative works on different terms, provided the original work is properly cited and the use is non-commercial. See: http://creativecommons.org/licenses/by-nc/4.0/
Peer-review started: August 30, 2014
First decision: September 30, 2014
Article in press: December 31, 2014
P- Reviewer: Bener A S- Editor: Tian YL L- Editor: A E- Editor: Wu HL
References
Articles from World Journal of Diabetes are provided here courtesy of Baishideng Publishing Group Inc
Citations & impact
Impact metrics
Article citations
Dietary acid load adopts the effect of ApoB ins/del genetic variant (rs11279109) on obesity trait, cardiovascular markers, lipid profile, and serum leptin level among patients with diabetes: a cross-sectional study.
Sci Rep, 14(1):25650, 27 Oct 2024
Cited by: 0 articles | PMID: 39465244 | PMCID: PMC11514203
Tracing links between micronutrients and type 2 diabetes risk: the singular role of selenium.
Front Endocrinol (Lausanne), 15:1422796, 14 Oct 2024
Cited by: 0 articles | PMID: 39469571 | PMCID: PMC11513319
Predicting diabetes in adults: identifying important features in unbalanced data over a 5-year cohort study using machine learning algorithm.
BMC Med Res Methodol, 24(1):220, 27 Sep 2024
Cited by: 0 articles | PMID: 39333899 | PMCID: PMC11430121
Serum Uric Acid and Microalbuminuria: Predictors of Renal Dysfunction in Type 2 Diabetes Patients in South-Western Uganda.
Cureus, 16(9):e69843, 21 Sep 2024
Cited by: 0 articles | PMID: 39435249 | PMCID: PMC11492550
Blended Tea Ameliorates T2DM via Modulation of Gut Microflora.
Plant Foods Hum Nutr, 79(4):851-859, 17 Aug 2024
Cited by: 0 articles | PMID: 39153160
Go to all (179) article citations
Similar Articles
To arrive at the top five similar articles we use a word-weighted algorithm to compare words from the Title and Abstract of each citation.
Stress and the inflammatory process: a major cause of pancreatic cell death in type 2 diabetes.
Diabetes Metab Syndr Obes, 7:25-34, 03 Feb 2014
Cited by: 84 articles | PMID: 24520198 | PMCID: PMC3917922
Review Free full text in Europe PMC
Expert Group Consensus Opinion: Role of Anti-inflammatory Agents in the Management of Type-2 Diabetes (T2D).
J Assoc Physicians India, 67(12):65-74, 01 Dec 2019
Cited by: 10 articles | PMID: 31801334
The Role of HMGB1 in the Pathogenesis of Type 2 Diabetes.
J Diabetes Res, 2016:2543268, 22 Dec 2016
Cited by: 52 articles | PMID: 28101517 | PMCID: PMC5215175
Review Free full text in Europe PMC
Diabetes and branched-chain amino acids: What is the link?
J Diabetes, 10(5):350-352, 13 Feb 2018
Cited by: 116 articles | PMID: 29369529