Abstract
Free full text

Zwitterionic gel encapsulation promotes protein stability, enhances pharmacokinetics, and reduces immunogenicity
Significance
A protein modification technology has been developed in this study to overcome current problems faced by protein therapy. After being individually encapsulated in a super-hydrophilic zwitterionic gel, a therapeutic protein showed exceptional stability and long in vivo circulation half-life. More importantly, no immune response against either the protein or the polymer was observed following repeated injections. This technology will benefit patients by reducing administration frequency and mitigating adverse reactions and could allow more immunogenic proteins to enter into human therapeutic or protective applications.
Abstract
Advances in protein therapy are hindered by the poor stability, inadequate pharmacokinetic (PK) profiles, and immunogenicity of many therapeutic proteins. Polyethylene glycol conjugation (PEGylation) is the most successful strategy to date to overcome these shortcomings, and more than 10 PEGylated proteins have been brought to market. However, anti-PEG antibodies induced by treatment raise serious concerns about the future of PEGylated therapeutics. Here, we demonstrate a zwitterionic polymer network encapsulation technology that effectively enhances protein stability and PK while mitigating the immune response. Uricase modified with a comprehensive zwitterionic polycarboxybetaine (PCB) network exhibited exceptional stability and a greatly prolonged circulation half-life. More importantly, the PK behavior was unchanged, and neither anti-uricase nor anti-PCB antibodies were detected after three weekly injections in a rat model. This technology is applicable to a variety of proteins and unlocks the possibility of adopting highly immunogenic proteins for therapeutic or protective applications.
As an emerging class of therapeutics, protein drugs offer the advantages of higher specificity and potency than small molecules due to their structural complexity (1). However, this complexity increases their vulnerability to environmental changes and immune system recognition and thus hinders their formulation and delivery. Additionally, the immunogenicity of many protein drugs not only limits their therapeutic efficacy, but also threatens patients’ lives with adverse effects including anaphylaxis and infusion reactions (2). As a result, only minimally immunogenic proteins can be developed into human therapeutics, which greatly restricts the possibilities and diversity of protein therapies. Numerous efforts have been made to humanize nonhuman sourced proteins, but limited successes have been achieved compared with the bright initial prospects.
Conjugating polyethylene glycol (PEG) to proteins, known as PEGylation, is thus far the most successful tactic used to mitigate an immune response to therapeutic proteins (3–5). When it was first introduced to alter protein immunogenicity in the 1970s, PEG was thought to be nonimmunogenic and nonantigenic (6). However, several studies in the subsequent decades have found anti-PEG antibodies after treatment with PEGylated therapies, with titers strongly related to modification density and the immunogenicity of the anchoring protein (5, 7). The haptenic character of PEG is considered the main culprit in the therapeutic efficacy loss of PEGylated products. Recent clinical studies of Pegloticase (PEGylated uricase) in refractory chronic gout patients unequivocally demonstrated that anti-PEG antibodies correlate with a reduction in drug effectiveness—Pegloticase loses efficacy in more than 40% of patients, and the presence of anti-PEG antibodies doubles the risk of infusion reactions (8, 9). Similar results have also been observed for other PEGylated proteins in clinical use, including PEG-asparaginase (10) and Peginterferon alfa (11). PEGylated nanoparticles such as liposomes also stimulate a strong anti-PEG response, and these antibodies were found to cross-react with different PEGylated products (12, 13). Even more concerning, the prevalence of anti-PEG was found to be 22–25% in a sample of 350 healthy blood donors, attributed to daily exposure of PEG-containing consumer products (14). Altogether, these findings raise obvious concerns regarding the current and future efficacy of PEGylated drugs. An alternative to this gold standard strategy is urgently needed to supplement or replace PEGylation.
Based on the extensive studies of PEGylation, we believe that two issues are responsible for its immune response: the immunogenicity/antigenicity of PEG itself and limited PEG coverage of protein surfaces. Systematic studies have shown that PEG immunogenicity strongly correlates with the hydrophobic characteristics of this amphiphilic polymer (15–17); we thus hypothesize that a truly hydrophilic polymer should do a better job. Zwitterionic materials have emerged as an important class of extremely hydrophilic biomaterials, demonstrating particularly high hydration and ultra-low protein adsorption (18–20). Carboxybetaine is unique among these zwitterionic materials due to its structural similarity to glycine betaine, a naturally occurring compound in the human body (18). Surfaces modified with polycarboxybetaine (PCB) have exhibited protein adsorption below 0.3 ng/cm2 from 100% blood plasma or serum (21). In a more recent study, zwitterionic hydrogels comprising carboxybetaine monomer and cross-linker were shown to resist the foreign body reaction when implanted in mice for 3 months, due to the ultra-hydrophilic and biocompatible nature of the gel (22). Moreover, PCB-coated gold nanoparticles were shown not to induce a measurable antibody response in rats after repeated i.v. injections (23). These findings motivated us to test polymer-protein constructs using PCB as a minimally- or nonimmunogenic alternative to PEGylation. In terms of methodology, PEGylation processes conjugate multiple linear polymers to a protein surface to camouflage surface epitopes. Due to the limited number of conjugation sites available, or as a tradeoff to preserve protein activity, conjugated polymers may be insufficient to conceal all “danger signals.” The anchoring protein may in turn amplify the immunogenicity of conjugated polymers, causing the inverse correlation reported between the graft density and immune response (7). To overcome this limitation, we adopted bifunctional CB monomers to cross-link conjugated polymers into a “mesh” network, designed to possibly mask protein surfaces completely and maximize surface hydration. We evaluated the in vitro and in vivo performance of this zwitterionic polymer network encapsulation (ZPNE) technology by choosing highly immunogenic fungal-derived uricase as a model therapy and compared the stability, pharmacokinetics (PK), and immunogenicity to its PEGylated counterpart.
Results and Discussion
Zwitterionic Encapsulation of Uricase.
In our hypothesis, there are two crucial aspects to the design of protein protection strategies: the adoption of a superhydrophilic biomaterial and the comprehensive coverage of protein surface epitopes. A cross-linked carboxybetaine-based gel coating meets these criteria. However, incorporating a conventional cross-linker (e.g., ethylene glycol dimethylacrylate) may introduce unwanted hydrophobicity and compromise the hydrophilicity of the cross-linked gel coating. To avoid this detrimental possibility, we designed a bifunctional CB cross-linker carboxybetaine acrylamide cross-linker (CBAAX) (Fig. 1A) to maximize surface hydration. This carboxybetaine cross-linker has proven to be a key for PCB hydrogels to be capable of resisting the foreign body reaction (22) and restrain mesenchymal stem cell differentiation (24). To realize ZPNE in this work, each protein was encapsulated in a hydrogel nanoparticle through surface acrylation and in situ free radical polymerization reactions. Basic characterization data are shown in Fig. 1 and Table S1. Both PEGylated uricase and ZPNE-modified uricase (denoted as uricase-PCBX) shared a similar hydrodynamic size of around 30 nm, much larger than the ~10-nm diameter of the native uricase tetramer. Zeta potential measurements of uricase-PCBX showed a neutral surface charge (−2.2 mV), indicating more comprehensive surface coverage than that of uricase-PEG (−8.4 mV). Atomic force microscopy (AFM) results showed that uricase-PCBX nanoparticles have a mean diameter of 28.6 ± 6.1 nm, consistent with those results from dynamic light scattering (DLS) measurements. Gel permeation chromatography (GPC) results revealed that five PEG molecules were conjugated to each uricase subunit. It should be noted that due to the difficult “graft-to” conjugation of a large polymer to a protein, this was the maximum conjugation number obtained after conjugation conditions were optimized. In contrast, it was much easier to achieve higher degrees of surface modification with the ZPNE technique. To maintain a high residual activity (>90%), we limited the surface conjugation number to around 12. The whole modification process is simple and applicable to a wide variety of proteins. The core-shell structure of these zwitterionic nanocapsules exploits a highly hydrated polymer shell to render the protein core “stealth” to its surroundings and conceivably to immune system recognition. Compared with conventional protein-polymer conjugates, this core-shell structure provides better protection for its inner cargo.
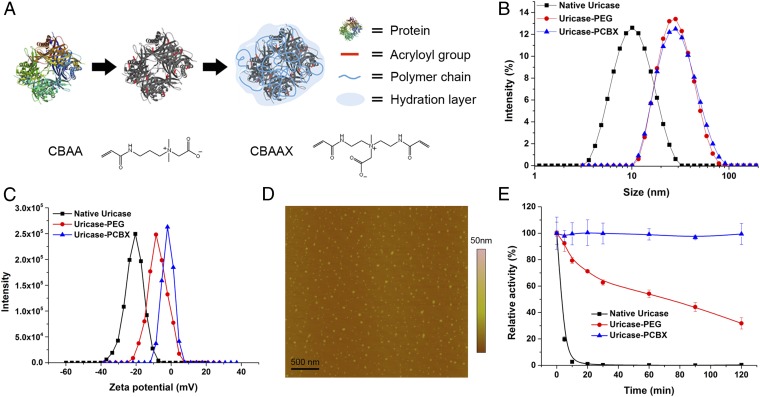
Zwitterionic protein encapsulation and characterization. (A) Molecular structure of CBAA monomer and CBAAX cross-linker and the process of ZPNE modification of a protein surface. (B) Size distributions and (C) zeta potentials of modified uricase vs. native uricase. (D) AFM image of formed zwitterionic nanocapsules. (E) Thermal stability study of modified uricase vs. native uricase, as retained activity after incubation at 65 °C.
Table S1.
Characteristics of uricase samples
Sample | Conjugation number | Hydrodynamic size (nm)* | PDI | Zeta potential (mV) | Activity (%) |
Native uricase | — | 10.9 | 0.17 | −20.8 | 100 |
Uricase-PEG | 5† | 30.6 | 0.21 | −8.4 | 93 |
Uricase-PCBX | 12‡ | 31.3 | 0.20 | −2.2 | 91 |
In Vitro Protein Stability.
Preparation of therapeutic proteins is often prone to instability during storage and use, and enhanced structural stability is a desirable attribute of modification technologies. Thermal stress tests at elevated temperatures are a facile approach to evaluate differences in protein stabilities in a relatively short time (25–27). A protein conjugate or nanocapsule stable at higher temperatures is likely to be stable at ambient temperatures for a longer period. To test the stability of modified proteins to environmental stressors, their enzymatic activities were assayed after incubation at 65 °C for different time periods and presented as the percentage of preheating activity retained. As shown in Fig. 1E, uricase-PCBX has exceptional thermal stability and retained 100% activity after 2 h of incubation. In contrast, uricase-PEG only exhibited a modest stabilizing effect.
The present result, that uricase-PCBX remains stable at 65 °C, suggests that it would not require low-temperature storage or formulation with stabilizing excipients as is the case for the native protein. This high stability is important when considering situations in which low-temperature storage or transportation is unavailable, and may help reduce the high cost of therapeutic protein production and use. In a previous study, PCB was shown to impart a superior protein-stabilizing effect and increase the substrate affinity to protein binding sites when similar protein conjugation structures were tested (26). The CB monomer unit is a derivative of glycine betaine, a natural molecule found in many living systems as a stabilizing agent. Encapsulating a protein in a PCB gel network is analogous to placing it into a highly concentrated protein stabilizing solution, equipping it with a protective microenvironment that accompanies the protein both in vitro and in vivo. Furthermore, the nanocapsule structure has been reported to stabilize enzymes through multiple covalent attachments between the enzyme core and polymer shell, which effectively hinder conformational changes in adverse environments (27, 28). Combining these two distinct mechanisms, it is unsurprising that PCB gel encapsulation affords superior stability to protein cargos.
PK Study.
Since the 1970s, PEGylation has been the gold standard method to alter PK properties of both proteins and a variety of nanoparticle platforms for drug delivery (3, 29). Surface attachment of PEG significantly prolongs in vivo circulation times by increasing hydrodynamic size to avoid rapid renal clearance (for small particles) and reducing interactions with both blood components (opsonization) and immune cells. The PK profile of ZPNE-modified uricase was compared with this gold standard in healthy Sprague–Dawley rats through repeated i.v. injections. Fig. 2 shows the circulation profiles of native and modified uricase after each injection. All concentration-time curves fit a two-compartment model. PK parameters are listed in Table 1. On the initial injection, uricase-PEG exhibited an elimination half-life (t1/2β) of 38.2 h, threefold higher than the 13.9 h t1/2β of native uricase. The area under curve (AUC∞) of PEGylated uricase is five times that of the native enzyme, roughly in agreement with values reported in the literature (30). In comparison, uricase-PCBX showed exceptionally prolonged circulation behavior, with a t1/2β of 134.9 h, a 3.6-fold improvement over uricase-PEG, and 10-fold higher than the native enzyme. Moreover, the AUC∞ of uricase-PCBX was 4.2 times that of uricase-PEG, indicating its significantly higher systemic availability.
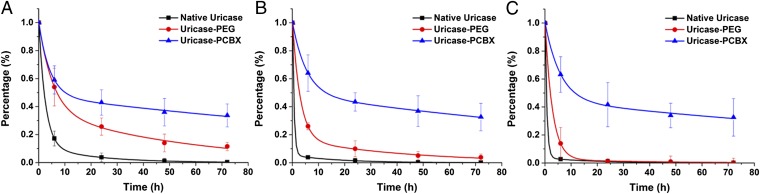
Blood circulation profiles of modified uricase vs. native uricase. Circulation curves after the (A) first i.v. administration, (B) second administration, and (C) third administration.
Table 1.
PK parameters after repeated injections
Parameters | Native uricase (different injections) | Uricase-PEG (different injections) | Uricase-PCBX (different injections) | ||||||
1 | 2 | 3 | 1 | 2 | 3 | 1 | 2 | 3 | |
t1/2α (h) | 1.7 | 0.4 | 0.5 | 3.7 | 2.1 | 1.9 | 2.9 | 3.7 | 4.0 |
t1/2β (h) | 13.9 | 12.5 | 13.2 | 38.2 | 30.6 | 24.8 | 134.9 | 121.3 | 145.5 |
AUC∞ (μg/mL × h) | 296 | 85 | 90 | 1,394 | 590 | 237 | 5,796 | 5,658 | 6,126 |
CL (mL/h) | 3.38 | 14.17 | 16.68 | 0.72 | 2.03 | 6.34 | 0.17 | 0.21 | 0.24 |
MRT (h) | 11.6 | 11.2 | 9.7 | 48.1 | 33.6 | 11.5 | 190 | 170 | 203 |
Uricase is a large tetrameric protein with a molecular weight of ~130 kDa. The rapid clearance of native uricase is considered to be associated with an irreversible uptake process, likely by the reticuloendothelial system (RES), rather than renal filtration (31). Conjugating a total of 20 large PEG molecules (each with a molecular weight of 10 kDa) to uricase can effectively cover a large portion of its surface, minimizing nonspecific uptake clearance. This phenomenon is closely related to material hydration and nonfouling character. As a well-known class of ultra-low fouling materials, zwitterionic polymers have indeed been used to extend the circulation lifetime of nanoparticles (32–34). Apart from material properties, recent research has shown surface coverage density to be another important factor affecting particle uptake by immune cells and in vivo circulation behavior (35). A high surface grafting density of PEG molecules, exceeding the minimum for a brush conformation, is required for nanoparticles to evade immune cell uptake and clearance. However, in the case of protein modification, there is a certain limit to conjugation density due to the restricted number of available functional groups on the surface of a protein. A cross-linked hydrogel “mesh,” which does not require a high number of conjugation sites, can effectively overcome this problem. A thin hydrogel layer can ideally mask the entire protein surface, making it “stealth” to surroundings and slowing down RES clearance. The results of this study confirm our hypothesis that greatly extended circulation profiles of protein therapeutics can be realized by PCB nanocapsule encapsulation. Patients could benefit greatly from this advance by enjoying a reduced administration frequency compared with PEGylated products.
Uricase is administered chronically in clinical practice to treat refractory chronic gout, so repeated injections were performed to evaluate the long-term performance of ZPNE-modified and PEGylated proteins. The second and third injections were given at days 7 and 14 after the initial injection. It should be noted that severe infusion reactions were observed 3–5 min after the second and third injections of both native uricase and uricase-PEG, with symptoms including hypopiesia and dyspnea. In contrast, no adverse effects were observed following the uricase-PCBX injections. Although the calculated t1/2β of native uricase appears comparable after each injection, the sampling frequency was likely inadequate for its rapid elimination; its accelerated elimination after repeated injections is more clearly reflected in the significantly reduced AUC∞. Following the second and third injections of uricase-PEG, an obvious accelerated blood clearance (ABC) phenomenon was noted, with t1/2β decreasing to 30.6 and 24.8 h, and AUC∞ values dropping 2.8-fold and 5.9-fold, respectively. This accelerated elimination process is most likely due to the induction of anti-PEG antibodies, which will be discussed in the following section. Unlike PEGylated uricase, the PK profiles of uricase-PCBX in this study did not show any significant change following three injections, indicating that uricase-PCBX is likely to maintain its superior PK performance in repeated applications.
Detection of Antibodies.
Serum collected at day 35 after the first injection was tested for both anti-uricase and anti-polymer antibodies using direct ELISA. As shown in Fig. 3 A and B, IgG was the major isotype of anti-uricase observed after repeated injections. The measured antibody titers are listed in Table S2. Due to the strong immunogenicity of the fungal enzyme, the native uricase group developed extremely high IgG antibody titers along with relatively high titers of IgM. In the group treated with PEGylated uricase, the titers of anti-protein antibodies were significantly lower than the native enzyme but still clearly elevated. In contrast, neither anti-uricase IgG nor IgM were detected in the rat group treated with uricase-PCBX. Our results here are consistent with clinical findings, showing that PEGylation cannot completely prevent the generation of anti-protein antibodies, at least for highly immunogenic uricase (9). This finding supports our hypothesis that comprehensive surface coverage of a protein with a stealth polymer network can effectively render the protein “invisible” to the immune system and thus mitigate antibody induction.

Detection of antibodies by direct ELISAs. (A) Anti-uricase IgM, (B) anti-uricase IgG, (C) anti-polymer IgM, and (D) anti-polymer IgG. Serum from each rat is plotted separately.
Table S2.
Antibody titers detected by direct ELISAs
Sample name | Rat no. | Anti-uricase IgM | Anti-uricase IgG | Anti-polymer IgM | Anti-polymer IgG |
Native uricase | 1 | 1:3,200 | >1:25,600 | — | — |
2 | 1:3,200 | >1:25,600 | — | — | |
3 | 1:3,200 | >1:25,600 | — | — | |
Uricase-PEG | 1 | 1:200 | 1:12,800 | 1:6,400 | 1:800 |
2 | 1:400 | 1:1,600 | 1:6,400 | 1:800 | |
3 | 1:400 | 1:3,200 | 1:6,400 | 1:800 | |
Uricase-PCBX | 1 | <200 | <200 | <200 | <200 |
2 | <200 | <200 | <200 | <200 | |
3 | <200 | <200 | <200 | <200 |
The severe infusion reactions and accelerated drug elimination seen in the native uricase group is apparently related to strong production of anti-uricase antibodies. However, for PEGylated protein therapeutics, clinical trial evidence suggests anti-PEG antibodies rather than anti-protein antibodies are primarily responsible for infusion reactions and efficacy loss over chronic injections (8, 9). Accordingly, strategies to suppress the production of anti-polymer antibodies remain a critical challenge in the development of polymer-protein conjugates. Uricase-PEG and uricase-PCBX groups were tested for anti-PEG and anti-PCB anti-polymer antibodies, respectively, and the results are shown in Fig. 3 C and D. Notably, at day 35, the major anti-PEG response comes from IgM (titers 1:6,400) rather than IgG (titers 1:800), suggesting differences in adaptive immunity pathways for the protein and polymer components in the conjugates. Compared with the strong response to PEGylated uricase, no IgG or IgM were detected against the polymeric component of uricase-PCBX.
As repeated injections are commonly needed for therapeutic proteins, the antibody response issue should not be overlooked and merits careful evaluation. It should be noted that in the earlier work establishing PEGylation as a method to alter protein immunogenicity (6), neither anti-BSA nor anti-PEG antibodies were detected after injecting BSA-PEG conjugates into a rabbit model. Three reasons may account for their observations. First, BSA shares a large degree of similarity with rabbit serum albumin (RSA) (36), which makes it less immunogenic as a model protein. In a later study, the generation of antibodies against PEGylated proteins was shown to be strongly related to the protein immunogenicity (7). This result explains why PEGylated nonhuman enzymes, e.g., uricase and asparaginase, have been reported to present the most severe issues in clinical trials resulting from anti-PEG antibodies (8–10). Second, a high degree of modification can be easily attained with BSA. The relationship between modification degree and immunogenicity of conjugates was also clearly demonstrated in the later study (7). Serum albumin is a class of protein with extraordinary ligand binding capacity (36), but this is not the case for most therapeutic proteins. Finally, immunological assays of the time, e.g., immunodiffusion and complement fixation tests, were not capable of detecting low-titer antibodies.
Because there is no standard method to evaluate anti-polymer antibodies, and especially as no positive control is available for the detection of anti-PCB antibodies, we validated our results using a surface plasmonic resonance (SPR) sensor. SPR is an ultra-sensitive technique used to study specific/nonspecific protein adsorption on surfaces with nanogram-level detection limits (21, 37). Compared with ELISA, SPR analysis is more straightforward, as the procedures are simpler, and each step can be carefully controlled and monitored. To detect anti-PEG or anti-PCB antibodies, we modified the surface of gold chips with poly(ethylene glycol) methyl ether methacrylate (PEGMA) or PCB polymer brush layers. Both PEGMA and PCB are nonfouling materials that resist nonspecific protein adsorption from diluted serum. When serum samples flow over the modified chip surface, only the polymer-specific antibodies can bind to the surface and generate an SPR signal through specific adsorption (Fig. 4A). The results are shown in Fig. 4. Sera obtained preinjections were used as a negative control. When sera from uricase-PEG–treated rats were flowed over a PEGMA modified chip, adsorption curves showed typical specific binding kinetics with adsorbed mass increasing linearly with time. Protein binding reached 40–50 ng/cm2 in 15 min under experimental conditions. No nonspecific binding was detected on the same chip from either control serum or uricase-PCBX serum, indicating all adsorption came from anti-PEG antibodies. In contrast, none of the sera showed detectable adsorption on a PCB-coated chip surface, suggesting that there is no antibody in the sera that can recognize and bind to PCB polymers.

Detection of antibodies by an SPR sensor. (A) Scheme showing the detection setup. The gold chip surface was modified with either PEGMA or PCB polymer brushes. The polymer brush serves dual functions, providing a nonfouling background while serving as the investigated antigen. (B) Summary of the SPR detection results. (C) Sensor signal-time curves on PEGMA-coated chips, each curve representing one serum sample. No uricase-PCBX serum showed any adsorption onto a PEGMA surface, though only one typical curve is shown. (D) Sensor signal-time curves on PCB-coated chips.
Biodistribution of Modified Uricase.
To examine the biodistribution of modified uricase samples following chronic use, we gathered blood and tissue samples from a treated rat 72 h after the third injection for further analysis. The third doses of uricase, uricase-PEG, and uricase-PCBX were labeled with a fluorescent probe for this test, whereas unlabeled formulations were given for the initial and second doses to avoid interference. As shown in Fig. 5, the data are presented as recovered fluorescence per gram of tissue. Uricase-PCBX sustained a high serum concentration, whereas all three samples accumulated primarily in the liver. Native uricase was observed to have less organ accumulation compared with protected enzymes three days after injection. This phenomenon can be explained by their different levels of protease stability. Native, unprotected uricase was readily degraded by proteases after liver accumulation, but protected uricase was partially shielded from participation in this metabolic process. As uricase nanocapsules were prepared using amide-containing cross-linkers, they are expected to degrade through slow hydrolysis. One potential advantage of this persistence is that these nanocapsule constructs may retain their ability to catalyze the oxidation of uric acid even after distribution into organs.
Conclusions
In summary, we demonstrate here that a polycarboxybetaine polymer network mesh coating on a protein effectively improves protein stability, extends PK, and mitigates immune response with superior efficacy to PEGylation. PCB-encapsulated uricase did not suffer the accelerated blood clearance encountered by PEGylated uricase during repeated injections in a rat model, and neither anti-uricase nor anti-PCB antibodies were detected in the serum. With its superior ability to mitigate protein immunogenicity, this reported technique makes it possible to adopt highly immunogenic proteins for human therapeutic or protective applications.
Materials and Methods
Protein Encapsulation and Polymer Conjugation.
For ZPNE, uricase was first modified to introduce acryloyl group onto its surface. The reaction was performed by dissolving 20 mg uricase into 10 mL 50 mM Hepes buffer (pH 8.5), followed by adding 75 µL N-acryloxysuccinimide (NAS) dimethyl sulfoxide (DMSO) solution (20 mg/mL) dropwise. The reaction was stirred at 4 °C for 2 h. The polymer encapsulation was done via in situ radical polymerization by adding 400 mg CBAA monomer, 80 mg CBAAX, 10 mL Hepes buffer, 15 mg ammonium persulfate (APS), and 60 µL tetramethylethylenediamine (TEMED) into the former reaction solution. After stirring for another 2 h, the reaction mixture was concentrated and washed extensively by PBS 7.4 using 300-kDa molecular weight cutoff centrifugal filters.
Uricase-PEG conjugates were prepared in 50 mM Hepes buffer (pH 8.5), with uricase concentration at 1 mg/mL and mPEG-NHS concentration at 10 mg/mL The reaction was stirred at 4 °C overnight. Then the conjugates were concentrated and washed extensively by PBS, pH 7.4, using a 300-kDa molecular weight cutoff centrifugal filter. The protein residue activity was measured by a commercially available uricase activity kit (Life Technologies).
PK and Biodistribution Study.
The PK of native and modified uricase is studied using Spraque–Dawley rats (male, body weight 74–100 g) as the animal model. Each sample has three duplicates to generate statistical significance. All animal experiments adhered to federal guidelines and were approved by the University of Washington Institutional Animal Care and Use Committee. For PK studies, each protein sample was administered into the rat via tail vein injection at the dose of 25 U/kg body weight. Blood samples were collected from the tail vein at 5 min, 6 h, 24 h, 48 h, and 72 h after the injection. The blood samples were put in heparinized vials and centrifuged, and the enzyme content in plasma was estimated by enzyme activity. The i.v. injections and bleeding procedure were repeated three times with 1 wk as time interval between each injection. Five weeks after the first injection, 5 mL blood was drawn by using cardiac punch, and serum was prepared for antibody detections. All PK parameters were calculated using PKSolver following the instructions.
For the biodistribution study, all rats were given unlabeled samples for the first and second dose, followed by FITC-labeled samples for the third dose. At 72 h after injection, all rats were killed, and the heart, liver, spleen, lung, kidney, and blood were collected for further analysis. The collected organ tissues were homogenized using a tissue ruptor, followed by centrifugation at 3,200 × g for 30 min at room temperature. The fluorescence of particles in the tissues was measured using a microplate reader and compared with a standard curve generated using FITC-labeled samples added to untreated homogenized tissues.
Statistics.
The Student t test was chosen to compare two small sets of quantitative data when data in each sample set were related, with P < 0.05 being considered as statistically significant.
SI Materials and Methods
Materials.
Recombinant uricase from Candida sp. and all chemicals were purchased from Sigma-Aldrich unless otherwise noted and were used as received. Methoxy polyethylene glycol succinimidyl carbonate, molecular weight 10 kDa (mPEG-NHS, 95%), was obtained from Nanocs. CBAA monomer was synthesized following a previously published method (37). Synthesis of CBAAX is described here.
Synthesis of CBAAX.
A solution of acryloyl chloride (5.3 mL, 65.1 mmol) in 20 mL dichloromethane (DCM) was added dropwise to a solution of N-methyl-2,2′-diaminodiethylamine (4 mL, 31.0 mmol) and N,N-Diisopropylethylamine (DIPEA) (11.9 mL, 68.3 mmol) in 50 mL DCM at 0 °C for a period of 30 min. The reaction mixture was allowed to warm to room temperature and stirred for 2 h. The reaction mixture was then washed with (2 × 25 mL) water. The aqueous layer was re-extracted with DCM (3 × 75 mL). The combined organic layers were dried using sodium sulfate, filtered, and concentrated in vacuo to leave a residue that was further purified by column chromatography to give compound 2 (5.2 g) in 75.3% yield. 1H NMR (300 MHz, D2O) δ 6.02–5.97 (m, 4H), 5.56–5.51 (m, 2H), 3.14 (t, J = 6.7 Hz, 4H), 2.37 (t, J = 6.7 Hz, 4H), 2.07 (s, 3H).
Compound 2 (3.2 g, 10.5 mmol) was dissolved in 20 mL acetonitrile, and tert-butyl bromoacetate (4.2 mL, 31.6 mmol) was added to it. The reaction contents were stirred for 18 h at 65 °C until the TLC showed complete consumption of starting material. The reaction mixture was concentrated to dryness in vacuo and further purified by column chromatography to give compound 3 (4.3 g) in 72.6% yield. 1H NMR (300 MHz, DMSO-d6) δ 6.31–6.08 (m, 4H), 5.67 (dd, J1 = J2 = 2.4 Hz, 2H), 4.48 (s, 2H), 3.75–3.54 (m, 8H), 3.29 (s, 3H), 1.45 (s, 9H).
Compound 3 (2.0 g, 4.7 mmol) was dissolved in 15 mL DCM, and 15 mL TFA was added to it. The reaction contents were stirred overnight at room temperature. After complete hydrolysis, the reaction mixture was concentrated in vacuo and coevaporated with DCM (3 × 15 mL). The resulting residual mixture was further dissolved in 15 mL methanol, and 4 g IRN-78 resin was added to it for complete neutralization. The reaction mixture was then filtered over celite and concentrated to dryness in vacuo. The residue was dissolved in water and lyophilized on a freeze dryer to give compound 4 in 84.1% yield. 1H NMR (300 MHz, DMSO-d6) δ 6.31–6.05 (m, 4H), 5.63 (dd, J1 = J2 = 2.4 Hz, 2H), 3.70 (s, 2H), 3.69–3.50 (m, 8H), 3.21 (s, 3H).
Measurement of Thermal Stability.
Modified protein samples (protein concentration 40 μg/mL in 0.1 M Tris buffer, pH 8.0) were incubated at 65 °C in water bath. At different time points, each sample was taken out and quenched in ice bath until measurement. After the final samples were taken out at 2 h, activities of all samples were measured using the uricase activity kit following the manufacturer’s protocol. Values were recorded as percentage of each sample activity before heating.
ELISA.
The antigens used in direct ELISAs consisted of native uricase (for detection of anti-uricase antibody), BSA-PEG conjugates (for detection of anti-PEG antibody), and BSA-PCB conjugates (for detection of anti-PCB antibody). BSA-PEG conjugates were made following the same procedure as uricase-PEG samples. BSA-PCB conjugates were made following similar procedure as making uricase-PCBX but with no cross-linkers during polymerization. For ELISA experiments, 100 μL antigen solution (10 μg/mL of protein concentration) prepared in 0.1 M sodium carbonate buffer, pH 10.5, was used to coat each well of the 96-well plates. During coating procedure, plates were incubated at 4 °C overnight. After removing antigen solutions, the plates were washed five times using PBS (PBS 7.4) and then filled with blocking buffer (1% BSA solution in 0.1 M Tris buffer, pH 8.0). It is important to avoid using any buffer that contains PEG-like detergents, e.g., Tween 20 and Tween 80. After incubation at room temperature for 1 h, blocking buffer was removed, and all wells were washed by PBS for another five times. Serial dilutions of rat sera in PBS containing 1% BSA were added to the plates (100 μL/well), which were incubated for 1 h at 37 °C. The plates were then washed five times with PBS. Goat anti-rat IgM or IgG conjugated to HRP (Bethyl Laboratories) was used as the secondary antibody for detection of IgM and IgG. After adding the secondary antibody, plates were incubated at room temperature for 1 h and then washed five times using PBS before the addition of 100 μL/well HRP substrate 3,3′,5,5′-tetramethylbenzidine (TMB; Bethyl Laboratories). The plates were shaken for 15 min, and 100 μL stop solution (0.2 M H2SO4) was added to each well. Absorbance at 450 (signal) and 570 nm (background) was recorded by a microplate reader. Prebleeding sera were used as negative control for all ELISA detections. Commercially available rat anti-PEG antibodies were used as positive control for anti-PEG detections. The positive signal was defined as absorbance significantly larger than corresponding negative control.
SPR Detection of Anti-Polymer Antibodies.
Both PCB and PEGMA polymer brushes were grafted onto gold-coated SPR sensor chips following previously published methods (37). Brush thickness was measured by a spectroscopic ellipsometer (Sentech SE-850). SPR detection of anti-polymer antibodies was optimized at different polymer brush thickness, and ~10-nm film thickness was found to have the best signal/noise ratio. A custom-built SPR sensor was used in this study. All experiments were done following the sequence of flowing PBS for 10 min, 1:20 diluted serum in PBS for 15 min, and PBS for 15 min at flow rate of 30 µL/min. For the SPR sensor used in the study, a 1-nm SPR wavelength shift represents a surface coverage of ~17 ng/cm2 for proteins. Detection limit for the SPR sensor used in this work is 0.3 ng/cm2.
Acknowledgments
This work was supported by the Defense Threat Reduction Agency (HDTRA1-13-1-0044) and National Institutes of Health (1R21EB020781-01).
Footnotes
The authors declare no conflict of interest.
This article is a PNAS Direct Submission.
This article contains supporting information online at www.pnas.org/lookup/suppl/10.1073/pnas.1512465112/-/DCSupplemental.
References
Articles from Proceedings of the National Academy of Sciences of the United States of America are provided here courtesy of National Academy of Sciences
Full text links
Read article at publisher's site: https://doi.org/10.1073/pnas.1512465112
Read article for free, from open access legal sources, via Unpaywall:
https://www.pnas.org/content/pnas/112/39/12046.full.pdf
Citations & impact
Impact metrics
Article citations
A Stealthiness Evaluation of Main Chain Carboxybetaine Polymer Modified into Liposome.
Pharmaceutics, 16(10):1271, 28 Sep 2024
Cited by: 0 articles | PMID: 39458603 | PMCID: PMC11510557
Hydrolytically Degradable Zwitterionic Polyphosphazene Containing HEPES Moieties as Side Groups.
Biomacromolecules, 25(10):6791-6800, 24 Sep 2024
Cited by: 0 articles | PMID: 39315416 | PMCID: PMC11480972
Impact of the Hydrophilicity of Poly(sarcosine) on Poly(ethylene glycol) (PEG) for the Suppression of Anti-PEG Antibody Binding.
ACS Omega, 9(32):34577-34588, 12 Jul 2024
Cited by: 0 articles | PMID: 39157078 | PMCID: PMC11325419
Enzyme stability in polymer hydrogel-enzyme hybrid nanocarrier containing phosphorylcholine group.
RSC Adv, 14(26):18807-18814, 11 Jun 2024
Cited by: 0 articles | PMID: 38863819
Polymeric Nanoparticles for Drug Delivery.
Chem Rev, 124(9):5505-5616, 16 Apr 2024
Cited by: 14 articles | PMID: 38626459
Review
Go to all (91) article citations
Similar Articles
To arrive at the top five similar articles we use a word-weighted algorithm to compare words from the Title and Abstract of each citation.
Polyethylene Glycol-Like Brush Polymer Conjugate of a Protein Drug Does Not Induce an Antipolymer Immune Response and Has Enhanced Pharmacokinetics than Its Polyethylene Glycol Counterpart.
Adv Sci (Weinh), 9(11):e2103672, 08 Feb 2022
Cited by: 12 articles | PMID: 35133079 | PMCID: PMC9008788
[Induction of Anti-PEG Immune Responses by PEGylation of Proteins].
Yakugaku Zasshi, 140(2):163-169, 01 Jan 2020
Cited by: 0 articles | PMID: 32009039
Pegloticase co-administered with high MW polyethylene glycol effectively reduces PEG-immunogenicity and restores prolonged circulation in mouse.
Acta Biomater, 170:250-259, 31 Aug 2023
Cited by: 1 article | PMID: 37659730 | PMCID: PMC10619887
Polymer Based Protein Therapeutics.
Curr Protein Pept Sci, 19(10):972-982, 01 Jan 2018
Cited by: 3 articles | PMID: 28828988
Review
Funding
Funders who supported this work.
DOD | Defense Threat Reduction Agency (1)
Grant ID: HDTRA1-13-1-0044
HHS | NIH | National Institute of Biomedical Imaging and Bioengineering (1)
Grant ID: 1R21EB020781-01
NIBIB NIH HHS (2)
Grant ID: 1R21EB020781-01
Grant ID: R21 EB020781