Abstract
Free full text

Sleep Function: Toward Elucidating an Enigma
Summary
Sleep function remains controversial. Individual perspectives frame the issue of sleep function differently. We briefly illustrate how sleep measurement and the evolution, tissue organization levels, molecular mechanisms, and regulation of sleep could influence one’s view of sleep function. Then we discuss six viable theories of sleep function. Sleep serves host-defense mechanisms and conserves caloric expenditures, but these functions likely are opportunistic functions evolving later in evolution. That sleep replenishes brain energy stores and that sleep serves a glymphatic function by removing toxic byproducts of waking activity are attractive ideas, but lack extensive supporting experimental evidence. That sleep restores performance is experimentally demonstrated and has obvious evolutionary value. However, this hypothesis lacks experimentally verified mechanisms although ideas relating to this issue are presented. Finally, the ideas surrounding the broad hypothesis that sleep serves a connectivity/plasticity function are many and attractive. There is experimental evidence that connectivity changes with sleep, sleep loss, and with changing afferent input, and that those changes are linked to sleep regulatory mechanisms. In our view, this is the leading contender for the primordial function of sleep. However, much refinement of ideas and innovative experimental approaches are needed to clarify the sleep-connectivity relationship.
Introduction
Sleep remains a scientific enigma. It is the last major physiological process for which there is a lack of consensus concerning its function. While asleep one does not eat, drink, or reproduce and one is vulnerable to predation due to reduced responsiveness to environmental stimuli. Whatever function(s) sleep may serve, it would seem that it must provide an evolutionary advantage above and beyond these seemingly negative selective pressures for sleep. Sleep may serve many functions although its evolutionary origins were likely driven by a primordial function and additional opportunistic functions were added later in evolution.1 By analogy, lungs likely evolved for gas exchange but now also serve acid-base balance, thermoregulatory, and vocalization functions. Two themes are emphasized in this review. First, viable theories of sleep function need to offer an explanation of the evolutionary value of the function, and why reduced responsiveness to afferent stimuli is required for, or a consequence of, the proposed function. Second, evolutionary adaptations, including sleep function, are mechanistically selected for and enabled at the biochemical level. Thus, sleep function and sleep regulation are inseparable as the same biochemical mechanisms serve both.
Many functions for sleep have been proposed; e.g. see the 1995 special issue “The Function of Sleep” of Behavioral Brain Research.2 We limit this review to six theories for one or more of the following reasons. 1) There is substantial evidence in their support, e.g., saving calories, immune enhancement, brain connectivity, performance restoration. 2) Known biochemical sleep mechanisms are inseparable from the function, e.g., brain connectivity. 3) They are consistent with the unfolding ideas concerning local sleep, e.g., brain connectivity, glymphatics, performance restoration. And 4) they are new and directly related to pathology, e.g., glymphatics. Each theory is proposed from unique vantage points and investigator history and thus upon how the proposers view sleep. We begin by addressing issues that affect these perspectives because they provide a broader understanding of proposals for, and allow a greater inclusiveness of, ideas for sleep function.
Perspectives on Sleep
Measurement of sleep
There is no single measure of sleep but many features can be monitored to define states of arousal. Thus sleep is identified from two or more physiological and behavioral variables that correlate with sleep. No single measure, e.g., the electroencephalogram (EEG), electromyogram, behavior, amplitude of evoked response potentials (ERPs), burst-pause patterns of action potentials, respiratory rate, gene expression, etc., always correlates with a sleep state. Further, sleep is unusual in that while most other physiological processes are typically defined at a single organizational level, sleep crosses multiple levels and may be defined at several of them alone or combined. Thus, the parameters used to define sleep prejudice views of sleep functions by constraining the definition of which organisms are capable of sleep and the tissue organizational level at which sleep occurs.3, 4
Evolution of sleep
All the parameters used to define sleep are ultimately dependent upon neuronal action potentials. Accordingly if there is a universal function for sleep, as defined by those parameters, it is by necessity confined to that portion of the phylogenetic tree that harbors interacting excitable cells.1 Sleep appears to occur in any organism with a neuronal/glial network; e.g., C. elegans, fruit flies, cuttlefish, and other non-homoeothermic animals appear to sleep. If we accept, for example, that C. elegans sleeps5, then one is led to several conclusions: a) Sleep is an emergent property of very small neuronal networks (C. elegans has only 302 neurons), b) Sleep is largely independent of anatomy because the loose networks of C. elegans sleep, as well as the highly organized complex brains of mammals. c) Sleep serves a function at the small network level.
Organizational levels of sleep
Countless mammalian lesion studies and post-stroke clinical outcomes indicate that regardless of the site of the lesion, sleep always occurs if the animal/human survives.6 This strongly suggests that sleep is a fundamental property of many local networks regardless of where they are located in brain. Further, experimental evidence directly demonstrates emergent sleep-like states in small neuronal/glial networks. Cortical columns oscillate between distinct states as defined by amplitudes of ERPs.4, 6, 7 Somatosensory or auditory ERPs induced by afferent input during sleep are of greater magnitude than ERPs induced by identical afferent input during waking periods.7 Thus, the afferent input to cortical column output (I/O) relationship, herein used to define cortical column state, discriminates between wake and sleep. When the whole animal is asleep, most cortical columns are in the sleep-like state in that their ERPs are of high amplitude. When the animal is awake, most, but not all, of the columns are in the wake-like state characterized by low-amplitude ERPs.7
Individually columns exhibit state homeostasis such that the longer an individual column is in the wake-like state, the higher the probability that it will transition into a sleep-like state, and vice versa. Further, the state of a cortical column can affect whole-animal behavior in a cognitive performance paradigm dependent upon activation of a single somatosensory cortical column.6 Thus, cortical columns oscillate between states and those states possess many of the characteristics that are used to define whole-animal sleep, i.e., reversibility, homeostasis, and state-dependent behavior outcomes, but independently of the state of the whole brain. These findings suggest that the search for sleep function begin with small neuronal/glial networks.
Neuronal/glial networks grown in vitro also possess properties characteristic of organism sleep. The default state of mature mixed cultures of mammalian neurons and glia is a neuronal action potential burst/pause firing pattern similar to that observed in thalamic and cortical neurons during non-rapid eye movement sleep (NREMS).8, 9, 10, 11 To obtain such cultures, cells from murine embryos or 1–3 day old mice are used and the development of electrical activity over the course of 2–3 weeks in culture parallels developmental changes in mammalian neonatal sleep.11 Stimulation of mature cultures with a mixed cocktail of excitatory neurotransmitters10 or electrical pulses11, 12 induces a wake-like state as determined by measures also used to characterize organism sleep in vivo. Thus, upon stimulation culture gene expression profiles parallel those of an awake mouse10 and the fraction of total action potentials occurring within a burst decreases (reduced burstiness).11, 12 Further, after electrical stimulation of cultures, there is a rebound of the sleep-like state suggesting that sleep homeostasis also occurs in vitro.11
Application of acetylcholine to cultured networks reduces culture action potential synchronization, whereas treatment with a gamma aminobutyric acid agonist enhances it.13 Optogenetic stimulation of mature cultures transfected with Channelrhodopsin 2 induces release, or neuronal expression of, substances involved in sleep regulation, including adenosine triphosphate (ATP), interleukin-1 beta (IL1) and tumor necrosis factor alpha (TNF).11 Application of TNF to the cultures induces a more intense sleep state as the burstiness, slow wave synchronization and slow wave spectral power increase; these effects are reversed during electrical stimulation.11 If cells are taken from mice that lack the IL1 receptor neuron-specific accessory protein (called AcPb) the cells fail to respond to electrical stimulation by decreasing slow wave synchronization or delta power as do cells from wild type mice.14 AcPb knockout mice sleep less after sleep deprivation, rather than more as wild type mice do.15 Collectively these results indicate that cultured networks display properties that also characterize sleep in intact animals.
Whether individual cells sleep is difficult to address experimentally. Individual neurons oscillate between states, as defined by characteristic firing patterns, e.g., the thalamic and cortical neuron action potential burst-pause patterns characteristically occurring during sleep. However, directly linking such patterns or ‘states’ of an individual neuron to a behavioral outcome, or other parameters characteristic of organism sleep or network sleep-like states, has proven elusive.
This issue of whether sleep serves a function related to small networks versus individual cells is central to the differences between two currently popular ideas of sleep function. Connectivity theories are fundamentally network theories because they involve synapses between two or more cells. In contrast, the idea that sleep serves to restore neuronal energy stores in the form of ATP16 or glycogen17 is fundamentally a cellular theory of sleep function. Theories that sleep serves a connectivity function and serves a role in brain energy homeostasis are not mutually exclusive. In fact, sleep could be a cellular property precisely because the cell’s biochemistry, including its metabolism, is driven by network activity. At the same time, ATP, adenosine and other cellular metabolic substrates that vary in proportion to neuronal energy demand, such as oxidized glutathione18, 19, promote sleep. The idea that sleep is dependent on prior I/O activity may provide an important clue for sleep function by linking activity-dependent biochemical changes to sleep causation (Figure 1).
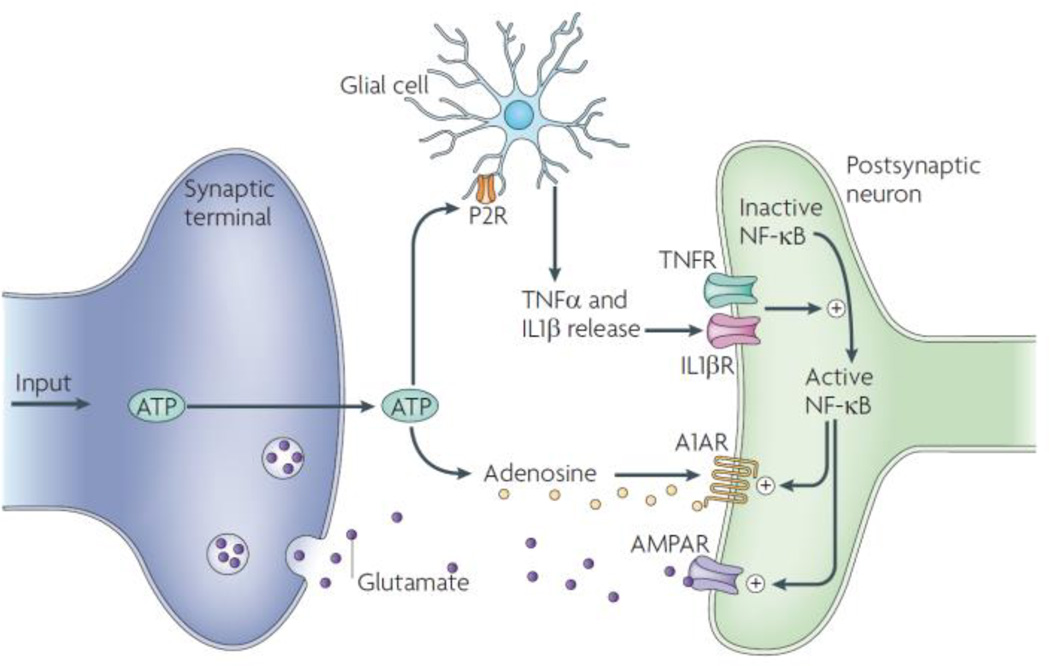
Cell activity-driven changes in sleep regulatory and neuronal connectivity related molecules. This simplified molecular network illustrates the principal of activity-driven changes in small neuronal networks. Adenosine triphosphate (ATP) is released as a consequence of action potentials in neurons and glial membrane potential changes into the extracellular space. ATP binds to purine type 2 receptors on glia causing the processing and release of multiple substances including interleukin-1 (IL1), tumor necrosis factor (TNF) (shown) and brain derived neurotrophic factor (not shown). These substances in turn alter neurotransmitter receptor populations and their location within the cell (called receptor trafficking). These actions change synaptic efficacy and thereby the input/output state of the neuronal network within which such synapses are located. Additional activity-dependent molecules, including NO, Ca++, neurotrophins and hormones, are also involved in small neuronal network state changes. For example, in order for IL1 to signal in neurons it requires the neuron-specific IL1 receptor accessory protein (AcPb); AcPb plays a role in sleep homeostasis15 and neuronal synaptogenesis.26 Abbreviations: P2R–purine type two receptor; R-receptor; NFkB-nuclear factor kappa B; A1AR-an adenosine type 1 R; AMPAR- a glutamate receptor. Figure from20.
Molecular sleep mechanisms
Substantial information concerning the biochemical sleep homeostat is available.20 The molecules involved, e.g., adenosine, ATP, nitric oxide, IL1, TNF, related molecules and receptors, and other proposed, albeit less studied, sleep regulatory substances, e.g., interleukin 6, growth hormone releasing hormone, epidermal growth factor, estrogen, neurotrophins21, all are involved in the regulation of synaptogenesis, synaptic efficacy, and/or neuronal-glial interactions, often via their actions on neurotransmitter receptor trafficking.22, 23, 24, 25, 26 They are also synthesized and/or released as a consequence of cell activity.27 The molecular regulators of sleep at a cell or small network level are involved in nervous system connectivity. To put this idea in perspective, Figure 1 illustrates an activity-dependent molecular network. Synaptic efficacy and connectivity within a neuronal network containing many such synapses will change as a consequence of the activity-ATP driven events illustrated. These events ultimately change neurotransmitter receptor populations thereby changing the neuronal network’s I/O relationships. Every molecule illustrated in Figure 1 also is implicated in sleep regulation. Thus it is easy to envision how within a small neuronal network containing many such synapses the synthesis/release of these sleep regulatory substances in the short run (milliseconds to seconds) lead to alterations in I/O relationships within the neuronal network (state change), and in the longer-term (minutes to days) alter receptor trafficking and thereby the frequency and duration of network state changes.
Sleep regulation
Lesion studies suggest that no particular area of brain is essential for sleep. The loss of any of the known sleep regulatory centers does not result in the complete absence of sleep. Further, slow stimulation of many areas of the brain induces a synchronized EEG that outlasts the stimulus suggesting “the whole encephalon has hypnogenic properties”.28 Jouvet rejected this conclusion based partially on evidence that the anterior hypothalamus is involved in sleep regulation29 and stimulation of the reticular activating system induces wakefulness.30 Although these findings demonstrate a clear role for these areas in sleep regulation, the lesion and slow stimulation studies strongly imply that sleep regulation, or at least local initiation of sleep, is fundamentally a bottom-up local circuit process rather than being merely imposed upon the brain by top-down sleep regulatory circuitry.6 For instance, acute in vivo manipulations of global sleep regulatory neurons by cell type-specific optogenetic activation do not acutely precipitate sleep as one would expect them to do if sleep were a top-down globally-controlled phenomenon, but rather such stimulation increases sleep over longer time scales (hours).31, 32
The transition from wakefulness to whole-organism sleep may begin as state changes within small networks, e.g., cortical columns, within complex brains6, 20, 33, 34 (Figure 2). The extensive and well-studied brain circuitry for global top-down sleep regulation29, 35 may not be essential to sleep as a phenomenon, but its existence suggests that orchestrating sleep at the whole brain level is important. This brings to mind the question of what is so important about sleep that evolution has preserved it – why not have brain circuitry designed to keep the brain awake and avoid the vulnerable state of sleep altogether? In the context of whole-organism sleep theories, no satisfactory answer to this question has been proposed. In the context of local sleep theories, however, the sleep state may be an emergent consequence of local neuronal/glial use – which needs to be orchestrated to minimize the risk associated with altered I/O relationships when dealing with the environment.20 If indeed sleep is an emergent property of local neuronal/glial network activity, then regulating sleep through global circuitry to occur at niche-appropriate times confers an evolutionary advantage. Regardless, the view that sleep is initiated at a local level suggests local sleep functions whereas if one views sleep being regulated by global top-down mechanisms different functions would be sought.

This figure illustrates how a small network, e.g. cortical column, is influenced by activity-dependent mechanisms involving cytokines and neurotrophins. The circle (left) and diamond (right) represent the same small network in the wake and sleep state receiving the same input 1 at two different times. The large square (center) shows molecular changes that drive the changes in state and connectivity (via Hebbian and scaling mechanisms). On the left, the wake state is illustrated with a particular input/output (I/O) relationship. The activity associated with waking induces activity-dependent molecular expressions that in turn alter connectivity and state. On the right, the sleep state is represented by a different I/O and the new output (O2) influences connectivity because O2 is different from O1 even though both receive the same input 1. Different synapses within the network are affected by O2 than those affected by O1 (left side). These actions set up an oscillation within the small network (cortical column). The actions set in motion by the cellular activities responsible for O1 and O2 can increase or decrease synaptic efficacy. The network’s connectivity and sensitivity to input signals never comes back to the initial condition as new experience is integrated into the network while old stimulus patterns are reinforced.
Proposed Sleep Functions
Sleep serves an immune function
Caregivers and physicians have historically suggested sleep as an aid for recuperation from disease states. Following the discovery that bacterial products (e.g., muramyl peptides) and endogenous immune response modifiers (e.g., IL1) enhance sleep, it was formally proposed that sleep serves host defenses.36 There is substantial evidence indicating that sleep or sleep loss affects immune system parameters.37, 38 For example, sleep loss is associated with a reduction in subsequent immunization-induced antibody titers.39 Further, multiple studies describe changes in sleep over the course of infectious diseases.40 An association was subsequently shown between robust sleep responses to infectious challenge and lowered morbidity and mortality.41 This correlation suggests that sleep does indeed help with the bodily function of recuperation from infectious disease. However, this theory of sleep function does not offer a reason for why unconsciousness accompanies sleep. The theory does offer an evolutionary value: conservation of calories during periods of high energy demand (e.g., fever). An immune function for sleep could be an example of an opportunistic function of sleep.
Sleep reduces caloric use
Whole-organism metabolic rate during sleep is lower than resting waking basal metabolic rate. In mammals, body and brain temperatures are regulated at a lower level during NREMS and torpor/hibernation is entered into from NREMS.42 The sleep function hypothesis that sleep serves to reduce caloric use is related to the idea that energy stores depleted during wakefulness are restored during sleep; the organism cannot continue to consume the same amount of energy during sleep as during wakefulness or it would not be able to catch up on energy supply. Regardless of the validity of this rationale, reduced energy use during sleep is likely to have adaptive value. Rest-activity cycles, even in bacteria, serve as an energy-saving and predator-avoidance strategy. It seems plausible that sleep evolved in lock-step within the context of rest-activity cycles.1 Whether reduction of caloric use is a primordial reason for the evolution of sleep, however, is not evident. Further, why loss of responsiveness to environmental stimuli is necessary for this function is not obvious or proposed.
Sleep restores brain energy stores
If the whole-body metabolic benefit of sleep is modest relative to the price paid in the inability to interact with the environment, perhaps there is a metabolic function specific to the brain.43 The idea that sleep serves a brain-specific metabolic function is supported by positron emission tomography studies in humans showing that brain glucose consumption is approximately twice as high during waking as during slow wave sleep.44, 45, 46 If brain metabolic down-regulation is a sleep function, it appears to be specific to NREMS, since brain metabolic rate is higher during rapid eye movement sleep than during waking.47
The NREMS-dependent decline in glucose utilization is not due to a lack of fuel supply: circulating glucose levels during sleep are not different from between-meal waking values48 and voltammetric measurements indicate an increase in brain glucose concentration, presumably due to reduced glucose consumption in slow wave sleep relative to wake.49 Rather, it may be the case that NREMS serves to reduce the brain’s demand for glucose, but why demand for glucose is reduced during NREMS relative to the desynchronized states is uncertain. Much of the brain’s energetic demand is driven by action potential-dependent synaptic transmission.50 Accordingly, the quiescence of large ensembles of neurons that coincides with slow wave activity in NREMS51 would be expected to precipitate a reduction in brain energetic demand.
Metabolic demand is thus a function of events at the cellular level. Addressing hypotheses that relate sleep to metabolism requires measurement of cellular energetic substrates. ATP is essential for maintaining the ion concentration gradients necessary for neuronal excitability, in large part as the energy source for the sodium-potassium ATPase.52 Glucose-derived fuels, especially glycogen, are necessary for the production of ATP in the brain. Therefore, ATP and glycogen are potential biochemical markers for an energetic function of sleep. Yet, testing of the energetic hypothesis of sleep has been hindered due to the rapid turnover of ATP within the cell and its exquisite sensitivity to changes in oxygen availability such as those occurring during dissection.53 Thus, direct measurement of changes in intracellular ATP concentration as a function of sleep wake cycles has not been performed. Phosphorylation of adenosine monophosphate kinase (AMPK), which occurs in response to elevation of the concentration of AMP relative to that of ATP, serves as a marker for fluctuations in cellular energetic status.54 Phospho-AMPK is elevated in the brains of animals euthanized immediately after sleep deprivation relative to those euthanized after sleep.55, 56 Therefore, the brain is either in a state of elevated AMP/ATP ratio in vivo during wake relative to sleep or is vulnerable to energetic shifts that would increase AMPK phosphorylation to a greater extent during dissection after protracted wake than after sleep.
It is also possible that due to its fundamental importance to the cell, ATP concentration is relatively invariant across sleep wake states, but that cellular substrates for ATP production, including glycogen, are mobilized due to the energetic demand imposed by protracted wakefulness as proposed previously.43 Glycogen, like ATP, is labile in the face of fluctuating conditions during dissection, which may to an extent explain inconsistencies in the literature on sleep/wake-dependent changes in brain glycogen. This lability can be mitigated to an extent by using microwave irradiation at the time of uthanasia57. In rodents, brain glycogen levels decrease during the first few minutes of waking and then stay low until the next sleep period.58 More recent findings suggest greater complexity. For example, glycogen declines in cerebellum, but not in the cerebral cortex, during sleep deprivation.59, 60 Additionally, some mouse strains exhibit a decrease in glycogen in cerebellum during sleep deprivation while others exhibit elevated glycogen in the cerebral cortex and no change in the cerebellum60; glycogen increases in cerebral cortex and cerebellum during sleep deprivation61; glycogen increases in whole brain and most sub-regions thereof during sleep deprivation.57 A study in Drosophila found that glycogen decreases in whole brain during the first three hours of rest deprivation, and then is restored to baseline levels.62 Given the limitations inherent in steady-state measurements of glycogen, the effects of sleep/wake cycles on the enzymes that regulate glycogen synthesis may be more informative with regard to the energetic hypothesis of sleep. As reviewed very recently,17 neurochemical changes associated with wakefulness, including elevated adenosine concentration and monoaminergic signaling upregulate enzymes that promote glycogenesis. This mechanism promotes increased glycogen synthesis during wake relative to sleep. The resulting glycogen must be utilized at a faster rate to address energetic demand during wake, resulting in no net change in glycogen concentration, but rather elevated glycogen turnover in wake relative to sleep. Accordingly, sleep does not reverse wake-dependent depletion of glycogen, but instead may free energetic resources for reactions other than those that facilitate the local production and use of glycogen in service of wake-related neuronal excitability.
A metabolic-transcriptional network links sleep and cellular energetics in the brain.63 Further, several cellular metabolic sensors (including adenosine monophosphate-activated protein kinases) and their metabolic regulators (ATP, glycogen, and nicotinamide adenine dinucleotide) are linked to sleep. For instance, sleep seems to be regulated, in part, by extracellular ATP via activation of purine type 2 receptors64 (Figure 1), but this is related to ATP as an extracellular signaling molecule and its release as a consequence of cell activity, not to its intracellular function in energy homeostasis although it seems likely that these two functions of ATP are linked. Until techniques are developed whereby putative metabolic regulators such as ATP can be simultaneously manipulated and monitored in the brain, the brain energy restoration function of sleep remains enticing but theoretical.
Sleep serves a glymphatic function
The proposed glymphatic function of sleep65 is dependent upon enhanced convective flow from the brain to the circulation during sleep. The convective flow would drag toxins and other brain products, thereby removing them from brain. Increased cell activity associated with wakefulness increases the conversion of large substances, e.g., glycogen, to small molecules, e.g., H+, CO2, and lactate, thereby increasing cellular osmotic pressure and volume. Because the brain is a closed compartment and water is essentially not compressible, cell activity is associated with changing ratios of cell volume to extracellular fluid volume. Increased brain cell activity also induces transient local increases in cerebral blood flow, thereby increasing local extracellular fluid volume. Very recently a brain lymphatic system has been described66; it may provide an avenue for removal of extracellular fluid and the substances it contains including hypnotoxins initially proposed over 100 years ago.67
Sleep-dependent enhancement of the removal of extracellular amyloid-β from the brain suggests that the sleep-wake cycle plays a role in the pathogenesis of Alzheimer’s disease.68 This work was recently extended by showing that during sleep or anesthesia, the ratio of extracellular fluid volume to cell volume is higher than during waking.65 This correlates with an increase in convective exchange of interstitial fluid with cerebrospinal fluid, which may also serve to remove neurotoxic products that accumulate during wakefulness, as had been shown previously.69
While conceptually attractive, the idea that sleep serves a glymphatic function currently needs more investigation, including distinguishing between states of anesthesia and sleep and addressing the issue of rapid state changes characteristic of polyphasic sleep of most vertebrates. It is also unclear if this proposed function is directly linked to sleep homeostasis. Finally, it is not clear why unconsciousness is needed for, or a consequence of, the proposed function.
Sleep restores waking-induced performance degradation
Sleep loss leads to cognitive and behavioral performance dysfunction. This is observed at the whole-organism cognitive-behavioral level70, 71 and in a cognitive performance paradigm dependent upon activation of a single somatosensory cortical column.6 The degree of impairment is wake-dependent and use-dependent.72 Following sleep deprivation, performance is restored by sleep73 in a sleep dose-dependent manner.74 Sleep is thus proposed to serve a function of restoring optimal performance.
It is posited that task performance during sleep deprivation may be degraded by sleep occurring locally in response to prior use in neuronal networks subservient to the cognitive processes associated with a given task.72, 75 At the level of cortical columns involved in the specific task, neuronal/glial activity results in release of ATP into the extracellular space initiating the events depicted in Figure 1 thereby facilitating the cortical column network sleep-like state.20 While in the sleep-like state, the neuronal network I/O changes (Figure 2) and no longer processes information meaningfully in relation to the task at hand, resulting in degradation of cognitive processing.76, 77 Similar mechanisms may underlie the time-on-task effect and the transient state of sleep inertia immediately following awakening from sleep.72, 75, 78
The proposed local mechanism for cognitive impairment is fundamentally local and use-dependent and thus task-specific. It provides a possible explanation for why performance vulnerability to sleep loss on “simple” tasks such as the psychomotor vigilance test is measurable.79 Such tasks use limited, task-specific, 78 cognitive pathways, and as such repeatedly activate the same neuronal pathways causing local sleep and performance degradation.77 This proposed mechanism of performance impairment due to sleep deprivation remains to be experimentally demonstrated. Regardless, the proposed function of sleep optimizing performance is attractive as it provides an obvious evolutionary advantage.
Sleep serves a connectivity function
The concept that sleep may serve a neuronal/glial connectivity (plasticity) function developed from knowledge of the mechanisms of the dynamics of neuronal plasticity and logical extensions of that body of knowledge.33, 80, 81, 82, 83, 84 Neurons and glia spontaneously connect to each other to form cellular networks connected via synapses between neurons and via extracellular signaling regulatory molecules (Figure 1). These processes are influenced by gene expression and the affected signaling molecules and synapses alter network properties. The expressions of many of the genes involved are activity-dependent and are involved in the regulation of connectivity and sleep (Figure 2).
The activity-dependence of the expression of these genes provides a mechanism to target synapses that are used. Use-dependent gene expression of connectivity molecules is an established epigenetic brain plasticity mechanism. However, this mechanism leads to strengthening synaptic efficacy of active synapses and therefore re-use. This positive feedback leads to even more re-use. The eventual consequence would be highly connected, rigid networks and a loss of brain plasticity, which would stifle adaptation and would be detrimental to survival.33, 81 Negative feedback mechanisms are needed to curb and stabilize plasticity.
To investigate this proposed function of sleep, it is important to establish whether sleep or sleep loss affects synaptic efficacy or synaptogenesis, the directions of those effects, and whether sleep is interacting with afferent input to elicit such changes. As discussed elsewhere85, an inclusive review of the literature shows that sleep does not have a simple, unidirectional effect on synapses. Instead, the effects of sleep depend on a number of factors including the circuit under examination, the age of the animal, and the kinds of experience that precede sleep. For example, in rodents excitatory electrophysiological potentials (EPSPs) in frontal/parietal cortices are larger when measured after long periods of continuous wakefulness (e.g., 6–12 hours) compared to sleep. There is also a reduction in NREMS neuronal spike rate after long periods of sleep, while long periods of waking are associated with a general increase in cortical excitability. In these studies, however, the animals were simply analyzed after normal or enforced periods of wakefulness. The animals were not challenged in ways that cause demonstrable changes in synapse number or strength.
Sleep has very different effects on synapses when it is instead preceded by experience that induces plasticity as has been demonstrated in motor and sensory cortex. In adult mice, sleep is required for long term potentiation (LTP) in the visual cortex induced by visual experience86. This form of plasticity, stimulus response plasticity, is considered an in vivo form of LTP because it satisfies basic criteria for Hebbian plasticity (e.g. input specificity) and shares mechanisms in common with classic hippocampal LTP (e.g. dependence on NMDA receptors, AMPAR trafficking).87, 88 At the level of single neurons this potentiation does not occur until mice sleep. Comparable results are reported in a canonical model of experience-dependent plasticity in the cat.89 In these experiments, 6 hours of monocular deprivation in the awake animal is sufficient to weaken cortical circuits serving the deprived eye. After sleep, cortical circuits serving the non-deprived eye become stronger based on electrophysiological measurements of single neurons.89, 90, 91 These changes are accompanied by molecular changes consistent with long-term synaptic potentiation. During sleep, AMPA receptors are phosphorylated in ways that increase receptor trafficking to the post-synaptic membrane. The first few hours of sleep are also characterized by the activation of protein kinases and the synthesis of proteins known to mediate LTP.89, 90, 92
Similarly, rotorod training in mice leads to a form of motor learning and new dendritic spine formation in motor cortex in vivo.93 The rate of spine formation is maximal during sleep and reduced after sleep deprivation. In addition to promoting new spine formation, sleep also stabilized spines formed after learning. Interestingly, there was no evidence of spine removal after sleep, either in the untrained or trained conditions.
These studies have not gone unchallenged because they call into question a popular theory that sleep globally weakens synapses.83, 94 It was argued, for example, that the effects of sleep in the mouse visual cortex merely reflect a relative change in the response to one stimulus vs. another, or could be explained by a general increase in excitability after training.94 There is, however, no evidence that stimulus response plasticity involves any change other than an increase in synaptic potentiation to the trained stimulus.87, 88 Nor can a general rise in excitability after training explain a gain of response only to the trained stimulus. It was also claimed that the results in motor cortex do not demonstrate a specific role for sleep because spine number after training is similar when measured after the active or sleep phase.94 However, sleep amounts were neither measured or controlled in that one supplementary study.93 Therefore one cannot deduce the role of sleep based on this single figure. With respect to the cat studies, it was claimed that monocular deprivation produced ‘massive’ synaptic weakening and reduced NREM slow wave activity.83 In fact, 6 hours of monocular deprivation, does not lead to massive synaptic weakening89 and has no significant effect on visual cortical slow wave activity.90 Therefore these criticisms are without basis. A reasonable conclusion is that sleep promotes synaptic strengthening or new synapse formation when it is preceded by experience that triggers plasticity.
Theories stating that sleep serves a connectivity function comprise a range of more specific hypotheses for sleep functions. These include hypotheses that sleep erases obsolete memories,80 consolidates new memories,95, 96, 97 solidifies neuromuscular circuitry,98 stabilizes and preserves plasticity,33, 81 downscales glutamatergic synaptic transmission,82, 83 increases synaptic efficacy,99 and allows prophylactic cellular maintenance.100 As briefly reviewed herein, there is a broad spectrum of experimental evidence showing the effects of sleep and sleep loss on a variety of specific brain plasticity/connectivity mechanisms. The wide scope of effects is sufficient to accommodate many specific brain plasticity/connectivity theories for sleep function. These theories for sleep function are attractive because of the obvious benefit that brain plasticity/connectivity has for survival. Regardless, despite our accumulating knowledge of sleep-connectivity issues and the many creative ideas surrounding this issue, these experimental findings and theories do not provide an adequate explanation for the occurrence of unconsciousness associated with sleep. They do, however, provide an explanation for the need for unconsciousness as outlined in the conclusion and this may be a constructive step towards the understanding of unconsciousness.
Conclusion
During wakefulness, repeated activation of neurons in a network results in changes in the network’s electrical and chemical outputs and responsiveness to inputs and thus a new state (Figure 1). The new network outputs associated with local sleep states are qualitatively and quantitatively different from the original outputs (Figure 2) and are emergent as a different state we call sleep. These network state changes may summate and synchronize across multiple local networks leading to the emergence of sleep.34 Sleep is thus fundamentally initiated as a local network state change and is dependent on prior use. While awake, local network I/O relationships generally are adaptive as evidenced by their net summated output originating from a living animal. The transition into a different state as a consequence of prior activity induces different local I/O relationships. These new I/O relationships may lack relevancy to the immediate environment status. If too many local circuits transition simultaneously into the new I/O relationships (the sleep state) then a need is created to prevent the animal from behaviorally interacting with the environment. Global sleep–wake regulatory circuits likely ensure the absence of behavior at such times in a circadian-mediated, niche-appropriate manner.
Acknowledgments
We thank Drs. Greg Belenky and Hans Van Dongen for their input to this manuscript. This work was supported by National Institutes of Health grants R01NS025378 and R01HD036520 to JMK, R03NS082973 and R01NS078498 to JW, R01EY019022 and R01HL114161 to MF; and by National Science foundation grant CNS-1058124 to SR.
Abbreviations
A1AR | adenosine type 1 receptor |
AcPb | brain-specific interleukin 1 receptor accessory protein |
AMP | adenosine monophosphate |
AMPA | a glutamate receptor |
AMPK | adenosine monophosphate kinase |
ATP | adenosine triphosphate |
EEG | electroencephalogram |
ERP | evoked response potential |
EPSP | excitatory postsynaptic potential |
IL1 | interleukin-1 beta |
I/O | input/output |
LTP | long-term potentiation |
NFkB | nuclear factor kappa B |
NMDA | a glutamate receptor |
NREMS | non-rapid eye movement sleep |
P2R | purine type 2 receptor |
TNF | tumor necrosis factor alpha |
Glossary of terms
Evoked response potential | The extracellular localized electrical response of the brain to an afferent input. ERPs can also be obtained from co-cultures of cells grown in vitro in response to a stimulus |
Hebbian plasticity | Brain processes that strengthen effective synapses and weaken ineffective synapses |
Optogenetic stimulation | The stimulation of cells with blue light transfected with a gene derived from algae called channelrhodopsin2. Channelrhodopsin2 is sensitive to blue light and is an excitatory ion channel |
Synaptic scaling | Synaptic scaling is a homeostatic process whereby an increase in network activity causes a slow compensatory decrease in the efficacy of excitatory synapses and a decrease in network activity increases excitatory synaptic strength. Synaptic scaling serves to regulate Hebbian processes |
Footnotes
Publisher's Disclaimer: This is a PDF file of an unedited manuscript that has been accepted for publication. As a service to our customers we are providing this early version of the manuscript. The manuscript will undergo copyediting, typesetting, and review of the resulting proof before it is published in its final citable form. Please note that during the production process errors may be discovered which could affect the content, and all legal disclaimers that apply to the journal pertain.
The authors declare no conflicts of interest.
References
Full text links
Read article at publisher's site: https://doi.org/10.1016/j.smrv.2015.08.005
Read article for free, from open access legal sources, via Unpaywall:
http://manuscript.elsevier.com/S1087079215001033/pdf/S1087079215001033.pdf
Citations & impact
Impact metrics
Citations of article over time
Alternative metrics
Smart citations by scite.ai
Explore citation contexts and check if this article has been
supported or disputed.
https://scite.ai/reports/10.1016/j.smrv.2015.08.005
Article citations
Tapeworm infection affects sleep-like behavior in three-spined sticklebacks.
Sci Rep, 14(1):23395, 08 Oct 2024
Cited by: 0 articles | PMID: 39379533 | PMCID: PMC11461891
NLRP3-mediated autophagy dysfunction links gut microbiota dysbiosis to tau pathology in chronic sleep deprivation.
Zool Res, 45(4):857-874, 01 Jul 2024
Cited by: 3 articles | PMID: 39004863 | PMCID: PMC11298670
The abnormalities of brain function in females with primary insomnia: a resting-state functional magnetic resonance imaging study.
Front Neurosci, 18:1414154, 31 Jul 2024
Cited by: 0 articles | PMID: 39145301 | PMCID: PMC11322055
Effectiveness of Brain Gym Exercises Over Cognitive Behavioural Therapy in Improving Sleep Quality Among Healthcare University Students: A Comparative Study.
Cureus, 16(4):e58463, 17 Apr 2024
Cited by: 0 articles | PMID: 38765356 | PMCID: PMC11100550
Alterations in Circadian Rhythms, Sleep, and Physical Activity in COVID-19: Mechanisms, Interventions, and Lessons for the Future.
Mol Neurobiol, 03 May 2024
Cited by: 0 articles | PMID: 38702566
Review
Go to all (134) article citations
Similar Articles
To arrive at the top five similar articles we use a word-weighted algorithm to compare words from the Title and Abstract of each citation.
Sleep homeostasis.
Curr Opin Neurobiol, 23(5):799-805, 17 Mar 2013
Cited by: 44 articles | PMID: 23510741
Review
Local use-dependent sleep; synthesis of the new paradigm.
Curr Top Med Chem, 11(19):2490-2492, 01 Jan 2011
Cited by: 48 articles | PMID: 21906015 | PMCID: PMC3248786
Review Free full text in Europe PMC
Sleep function: current questions and new approaches.
Eur J Neurosci, 29(9):1830-1841, 28 Apr 2009
Cited by: 45 articles | PMID: 19473236
Review
Sleep and synaptic plasticity in the developing and adult brain.
Curr Top Behav Neurosci, 25:123-149, 01 Jan 2015
Cited by: 26 articles | PMID: 24671703 | PMCID: PMC7485264
Review Free full text in Europe PMC
Funding
Funders who supported this work.
NEI NIH HHS (1)
Grant ID: R01 EY019022
NHLBI NIH HHS (1)
Grant ID: R01 HL114161
NICHD NIH HHS (1)
Grant ID: R01 HD036520
NINDS NIH HHS (3)
Grant ID: R01 NS078498
Grant ID: R01 NS025378
Grant ID: R03 NS082973
National Institutes of Health (6)
Grant ID: R01NS025378
Grant ID: R01HD036520
Grant ID: R01NS078498
Grant ID: R01EY019022
Grant ID: R01HL114161
Grant ID: R03NS082973
National Science Foundation (1)
Grant ID: CNS-1058124